DOI:
10.1039/D2CS00267A
(Review Article)
Chem. Soc. Rev., 2022,
51, 7238-7259
Glycosylated gold nanoparticles in point of care diagnostics: from aggregation to lateral flow
Received
5th April 2022
First published on 27th July 2022
Abstract
Current point-of-care lateral flow immunoassays, such as the home pregnancy test, rely on proteins as detection units (e.g. antibodies) to sense for analytes. Glycans play a fundamental role in biological signalling and recognition events such as pathogen adhesion and hence they are promising future alternatives to antibody-based biosensing and diagnostics. Here we introduce the potential of glycans coupled to gold nanoparticles as recognition agents for lateral flow diagnostics. We first introduce the concept of lateral flow, including a case study of lateral flow use in the field compared to other diagnostic tools. We then introduce glycosylated materials, the affinity gains achieved by the cluster glycoside effect and the current use of these in aggregation based assays. Finally, the potential role of glycans in lateral flow are explained, and examples of their successful use given.
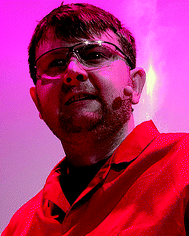
Alexander N. Baker
| Dr Alexander N. Baker is a research fellow in the Department of Chemistry at the University of Warwick. He completed his MChem (2007), and PhD (2022) in collaboration with Iceni Glycoscience at the University of Warwick. Alex's work, in the Gibson group, is focussed on organic and polymer synthesis to design medical diagnostics for low resource settings. His most recent work has developed diagnostic tools for the rapid detection of COVID-19 in collaboration with NHS partners and Iceni Glycoscience. Beyond his research, Alex is a founding fellow of the Warwick Institute of Engagement (WIE). |
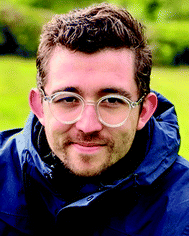
George W. Hawker-Bond
| Dr George W. Hawker-Bond is an Academic Foundation (AFP) doctor at Oxford University Hospitals NHS Foundation Trust and the Oxford University Clinical Academic Graduate School. He obtained his MChem (2017) degree from the University of Warwick, before qualifying in Medicine (MBChB) from Warwick Medical School in 2021. George's main research interests include the interplay between Chemistry and Medicine – with a particular focus in neuroscience and psychiatry – and medical education. He has also published on the involvement of under-represented groups in clinical trials. |
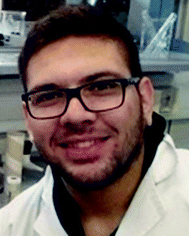
Panagiotis G. Georgiou
| Panagiotis G. Georgiou is a Marie Curie Early-Stage Research fellow and a PhD student in Chemistry at the University of Warwick. He obtained his BSc in Chemistry (with minor in Biological Chemistry) from the University in Cyprus in 2017. He then pursued an M.Sc. (by Research) in Chemistry at the University of Warwick under the supervision of Professor Rachel K. O’Reilly. His thesis involved the design and preparation of novel nanomaterials by polymerisation-induced self-assembly (PISA) for bio-related applications. He is currently pursuing his PhD at the University of Warwick (UK) under the supervision of Prof. Matthew I. Gibson. His project involves the development of novel nanomaterial biomimetics with advanced properties. |
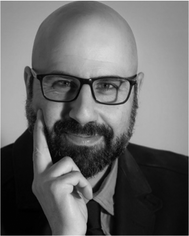
Simone Dedola
| Dr Simone Dedola is General R&D Manager at Iceni Glycoscience where he leads the chemistry and the assay development team. His specific expertise lies in the chemical synthesis of carbohydrates, total synthesis of glycoproteins, and the chemoenzymatic synthesis of glycoconjugates for vaccine and diagnostic programmes, assay development, in vitro diagnostics. Dr Dedola has experienced the journey from academia to SME, from academic high science to pragmatic SME R&D, he has been with the company from day one (> 6 years) and has a full appreciation of the company's activities, from concept and research through development to product prototyping. |
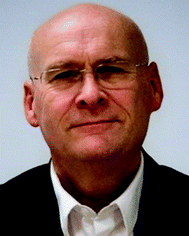
Robert A. Field
| Professor Rob Field is Director of the Manchester Institute of Biotechnology, Professor of Chemistry at the University of Manchester, and co-Founder/CSO of Iceni Glycoscience. He completed his degree and PhD at the University of East Anglia and went on to postdoctoral work at the University of Oxford (1989–91) followed by the University of Dundee (1992–94). He was appointed to the Chemistry Department (1994) at the University of St Andrews and promoted to full Professor (1999). Following this, he was at the University of East Anglia (2000–06) and the John Innes Centre (2007–19). Rob's research team investigates the chemistry of biological systems, including glycobiology. |
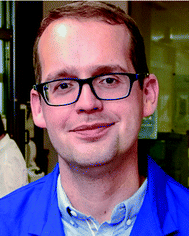
Matthew I. Gibson
| Professor Matthew I. Gibson holds a personal chair in the Department of Chemistry and also the Medical School at the University of Warwick, UK. Matt completed his Degree (2003) and PhD (2007) at the University of Durham (UK), before post-doctoral research at EPFL (Switzerland). Matt is currently a Royal Society Industry Fellow, ERC Consolidator Grant holder and co-founder of Cryologyx Ltd. Matt's multidisciplinary research group focusses on developing biomaterials to address healthcare challenges. Most recently they have developed tools for COVID-19 diagnostics and polymers to protect biologics during cold storage. |
1. Introduction – point of care
The diagnosis of infectious diseases should be achieved by; determining infection sites, considering patient needs and gaining a microbiological diagnosis. In many cases, however, diagnosis is guided by symptomatic presentation rather than therapeutic approaches as many of these methods can take hours or days to produce a result. Observational approaches alone can in some cases reduce patient outcomes and the injudicious prescribing of broad-spectrum drugs which can contribute to antimicrobial resistance (AMR).1
The first point-of-care (POC) diagnostic was developed in 1962 to measure blood glucose levels.2 This was followed by the rapid pregnancy test circa 1969.3,4 Both of these diagnostics revolutionised care: allowing for testing and diagnosis by or with the patient present. In 2011, point-of-care tests (POCT), or “bedside testing” devices were worth $15 billion USD with a projected increase of 4% per annum.5
While there is no accepted definition for point-of-care testing, it can be considered to be rapid testing at, or near to, the point of need that is used to make a medical decision.6–9 Current POCT devices include the aforementioned glucose biosensor, dipsticks and lateral flow devices (LFDs);10 such as Malaria Rapid Diagnostic Tests (MRDTs)11 and pregnancy tests, that separate samples through a solid phase.5
Surveys of medical professionals, in relation to POCT, have indicated that sensitivity (> 90%) is considered the most important attribute, followed by a price of less than $20 USD and a short detection time.12 This is summarised by the World Health Organisation (WHO) guidelines for the design of POCT in the ASSURED acronym (Fig. 1).13
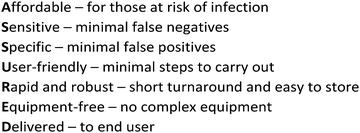 |
| Fig. 1 ASSURED acronym, WHO guidelines for the design of POCT. | |
The cost-effectiveness of POCT, compared to traditional laboratory techniques, has been demonstrated in the UK National Health Service (NHS) Health Checks, an early detection tool for cardiovascular disease;14 a disease which costs the NHS approximately £7 billion GBP per year.15 The total expected costs (TEC) per completed Health Check was markedly reduced for POCT compared to laboratory techniques – £18 GBP per completed Health Check compared to £25 GBP, respectively, and attendance rates were higher for POCT.14
It is equally important to consider the perspectives of patients and health care professionals (HCPs) to POCT. During an increase of Dengue fever cases in Singapore, POCT were found to be more convenient, and marginally better than non-POCT in improving the speed and accuracy of diagnoses.16
The alternative to POC testing is (highly accurate) laboratory-based testing, which require trained staff, embedded health infrastructures and specialised facilities. This makes lab-based methods, considered standard in wealthier nations, cost-prohibitive in low- and middle-income countries. This is evidenced by the COVID-19 (caused by SARS-COV-2) pandemic. PCR-based approaches have been the primary tool for diagnosis but is not ideal for mass testing due to price, turnaround time and the need for centralised infrastructure.17,18 It is estimated that laboratory-independent POCT for four common infections (syphilis, malaria, tuberculosis and bacterial pneumonia) could prevent more than 1.2 million deaths per annum in low- and middle-income countries.4 Chromatographic lateral flow paper-based immunoassays, as highlighted by MRDTs and pregnancy tests, are of particular interest as POCT because of their low cost, speed of use, laboratory-independence and wide range of devices and analytes currently in use.19
This review article introduces the potential of glycans (sugars/carbohydrates) to be deployed in rapid, lateral flow assays, compared to the currently used antibody-based LFDs. We first summarise the chemical basis of POC lateral flow immunoassay (LFIA) diagnostics that are based upon antibodies. We then provide a discussion on how a lateral flow assay has been deployed in the real world. Finally, we discuss glycosylated materials, in particular glycosylated gold nanoparticles. We focus on gold particles as these are the basis of commercial tests, however other material cores are highlighted. We cover the rapidly emerging literature showing the development of paper-based lateral flow glyco-assays, LFGA.
2. Lateral flow – an overview
Lateral flow devices (LFDs) typically employ antibodies as sensing components; these devices are often termed lateral flow (immuno) assays (LFIA), or “sol particle immunoassays” as originally termed by Leuvering et al.20 LFIAs have been developed for a variety of targets, including; complement;21 anabolic steroids;22 prostate-specific antigen;23 leprosy24 and meningitis25 in serum; evidence of kidney injury in urine;26V. cholerae in faeces;27 and other marker proteins28,29 or pathogens30 in whole blood samples.31 This ability to target a broad range of analytes has led to the use of lateral flow devices in policing illicit drug use, for example.32,33
LFDs typically contain two components; a mobile phase and a stationary phase. When a sample is applied to the strip's sample pad, it is drawn by capillary forces caused by the wick, through the device (Fig. 2A). Then the sample passes through the conjugate pad. If the sample contains the target analyte (Fig. 2Aa), it will bind antibody-coated gold nanoparticles (or other coloured nanomaterials) within the conjugate pad (Fig. 2A). The sample and nanoparticles (both bound and unbound) pass into the nitrocellulose membrane, where they move through two lines of the deposited material. One of these lines is a control line that containing antibodies specific to the primary antibody in the conjugate pad (Fig. 2Ad). Binding of the nanoparticles only to the control line indicates a successful test, but a negative sample (Fig. 2B). The test line contains antibodies specific to the analyte. A line will form if the analyte is “sandwiched” between antibodies immobilised in the test line and antibodies immobilised on the nanoparticles (Fig. 2Ac).
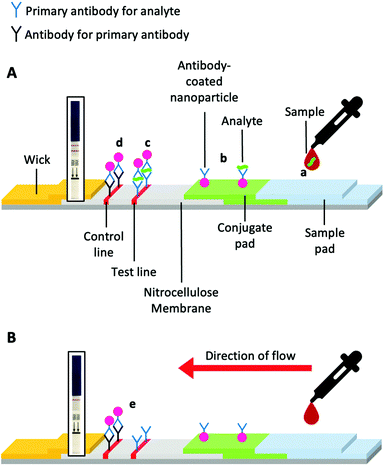 |
| Fig. 2 Schematic of a prototypical lateral flow device. (A) Device constituents in a successful positive test and (B) a successful negative test. Generic pregnancy tests for HCG detection inset. | |
There are examples of multiplexed lateral flow tests.34 This multiplexed approach allows for greater efficiency and greater diagnostic precision and may reduce overall cost when tests are combined into one device. An earlier form of the lateral flow test, called a flow-through assay, utilised an immobilised sample as a test line – these devices are often less sensitive than lateral flow devices. However, they are employed when lateral flow is not possible for economic or chemical reasons or during the prototyping and the development stages of LFD design.5,35
3. The components of lateral flow
The stationary phase
The stationary (immobile) phase comprises a receptor bound to the surface, usually an antibody. Larger antibodies do not require an immobilisation agent, with immunoglobulin G having an absorption of > 100 μg cm−2 on nitrocellulose; however, protein absorption can decrease with decreasing molecular weight.36 Bovine serum albumin (BSA), or less commonly ovalbumin, are used as carriers/immobilisation agents for small molecules such as nucleotides and small proteins.19,37 Less common immobilisation strategies include covalent epoxide–thiol and using fusion-proteins.38 Strategies to immobilise polynucleotides have also been reported.39,40
The low absorption of unconjugated antibodies was noted in polymeric systems by Aoyama et al.41 To overcome this they produced high surface area microcone architectures on polycarbonate sheets. The increased surface area enabled high antibody immobilisation levels. This step was inspired by the use of plastic and paper microfluidic systems,42 a rapidly growing technique that has been used to sense Ebola virus RNA,43Salmonella typhimurium44 and glucose.45
Studies with cellulose paper have also exploited biotin and the ability to covalently modify the cellulose surface for easy test line immobilisation.46,47 Tanaka et al. and Nagatani et al. have utilised the localised surface plasmon resonance (LSPR) phenomenon of gold nanoparticles in the stationary phase to enhance the signal-to-noise ratio when sensing for human chorionic gonadotrophin (HCG) and prostate-specific antigen respectively.23,48 This approach allowed for detection limits of 1 pg ml−1 of HCG,48 the hormone detected in the standard pregnancy test.
Reverse immunoassays that utilise “proteinticles” (protein-coated nanoparticles) in the stationary phase have also been developed.49 In these systems, antibodies against HIV and hepatitis A and B were tested in the mobile phase. However, the patient's immune system must mount an adaptive immune response for the test to be successful. Other tests, such as the Epstein-Barr Virus Antibody Test, also sense for an immune response to the pathogen. Although not POC, it does provide precedence for reverse immunoassay approaches.50
The mobile phase
Nanoparticles for biosensing have received significant attention due to their unique optical properties, making them ideal for sensing activities,51 and their tunability in terms of morphology and composition.52 Plasmonic, especially gold, particles are the most common signal generating units. Plasmonic nanoparticles are intensely coloured because their free electron density can couple with electromagnetic photons with a wavelength greater than the size of the nanoparticle.53–55 The colour of a nanoparticle is not only dependent on its size but also its shape and the dielectric constant of the metal and medium it is in, as shown by the Fröhlich condition.56–58 Only light resonant to electron oscillation can excite the localised surface plasmon and be absorbed by it; this absorption leads to localised surface plasmon resonance (LSPR), producing the distinctive colour of the nanoparticle system, which in the case of gold is from red to blue. Plasmonic nanoparticles have high molar attenuation (extinction) coefficients (ε), hence their use in LFDs. For example, 35 nm gold nanoparticles (AuNPs) and silver nanoparticles (AgNPs) have attenuation coefficients far higher than common organic dyes such as Sudan III, methylene blue and crystal violet. The best porphyrin dyes, such as 5,10,15,20-tetra-21H-23H-porphine zinc, also have lower attenuation coefficients than AuNPs (Table 1). High attenuation coefficients lead to bright, vivid colours even at low concentrations in nanoparticles systems, making them ideal for sensing applications.
Table 1 Attenuation coefficients of a selection of nanoparticles and dyes
Chromophore |
Molar attenuation coefficient (ε, M−1 cm−1) |
Wavelength, λMAX (nm) |
Gold nanoparticles, 35 nm59 |
∼6.1 × 109 |
506 |
Silver nanoparticles, 30 nm60 |
∼1.5 × 1010 |
406 |
Sudan III61 |
∼3.0 × 104 |
512 |
Methylene blue61 |
∼4.1 × 104 |
654 |
Crystal violet61 |
∼7.6 × 104 |
590 |
5,10,15,20-tetra-21H-23H-porphine zinc61 |
∼5.7 × 105 |
422 |
Gold nanoparticles (AuNPs, 15–800 nm) are the most widely used plasmonic particles31 due to their biocompatibility, simple synthesis, low cost (at the concentration used) and straightforward functionalisation with organic molecules, particularly thiols. The most common method for AuNP synthesis uses HAuCl4, and sodium citrate as both a reducing and capping agent.62–64 By using seeded growth, anisotropic nanocrystals, such as rods, wires and triangles can be obtained and used in lateral flow systems.65
AuNPs can be surface-modified with the analyte targeting components by surface passivation using sulphur-linked (for example, thiols and thioctic acids)66 or electrostatically charged antibodies or DNA strands for example.39 Capping ligands include; zwitterions, polymers, peptides, proteins, sugars and nucleic acids (Fig. 3).51 The vast majority of lateral flow systems utilise antibody capping groups and have been extensively reviewed.19,31,37
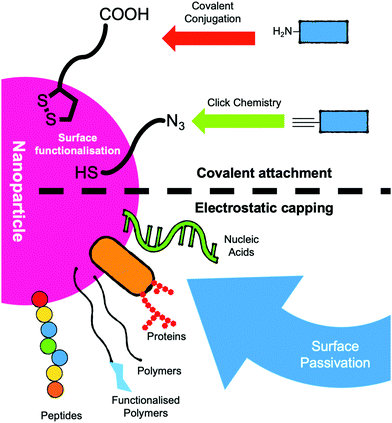 |
| Fig. 3 Schematic of strategies for the functionalisation of nanoparticles for use as the mobile phase in lateral flow devices. | |
In addition to gold, but outside of the scope of this review, other nanoparticles have also been tested in lateral flow systems, including; core–shell gold nanoparticles,67 quantum dots,68 graphene oxide69 and carbon nanotubes.70 Furthermore, Dou et al. have produced nanomaterial-free antibody systems71 using crystal violet-stained antibodies, enabling a test to run in 14 minutes.
While many lateral flow devices are designed for qualitative analysis, often by eye, it is possible to quantify analyte concentrations. Urusov et al. describe three methods for quantitative detection; optical detection using a camera, scanner, or smartphone etc and image analysis software, or magnetic labels or electrically conductive labels.72 In these methods the intensity of the test line is used as an indication of the analyte concentration, versus a calibration curve. The benefits of quantification, as described by Urusov et al. include the removal of human subjectivity, a lower limit of detection and an understanding of the target's concentration. However, the use of an additional device for analysis does decrease the applicability of some setups in low-resource scenarios.
The simplicity, diversity and broad applicability to many targets have made lateral flow systems an attractive diagnostic. Many other POCT devices and lab-based diagnostics have been designed for use in more economically developed countries (MEDCs) but are not compatible in less economically developed countries (LEDCs).73,74
4. Malaria rapid diagnostic tests – a case study
MRDTs in the field
Malaria Rapid Diagnostic Tests (MRDTs) have been deployed in low- and middle-income countries as a successful example of the benefits and drawbacks of lateral flow compared to other POC devices. The World Health Organisation (WHO) estimated 438,000 deaths from malaria in 2014, with 90% of these deaths occurring in sub-Saharan Africa (Fig. 4).30,75 In 2001, artemisinin-based combination therapies (ACTs) were recommended by WHO as first-line treatments.76 Within the decade (2009), resistance to first-line ACT treatments was observed in the Greater Mekong Sub-region.77–79 Resistance to ACTs has not been observed in Africa. However, there is concern that resistance will be observed.80,81 During the period from 2008–2015, 35% of suspected malaria cases in Africa were not confirmed with diagnostic tests, which led to antimalarial overuse, increasing the risk of ACT resistance.30,81,82 In the same period, resistance was further compounded by broader economic issues. Most malaria-affected countries use treatments costing less than $0.5 USD per capita, while artemisinin-based combinatorial therapies cost ∼$5 USD per capita.83–85 A shift to a diagnostics-led approach could alleviate this problem, with research in Nigerian community pharmacies demonstrating the cost-effectiveness of MRDT-led treatment.86 The United Nations Children's Fund (UNICEF) increased its procurement of Malaria Rapid Diagnostic Tests (MRDTs) from 3.8 million tests to 15 million.87
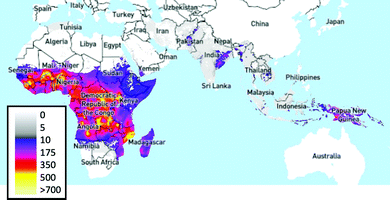 |
| Fig. 4
Plasmodium falciparum incidence per 1,000 people, 2017.88,89 Image produced using The Malaria Atlas Project, (Malaria Atlas Project, https://malariaatlas.org, (accessed 6 August 2019)).90 | |
MRDTs are POC immunochromatographic or photometric lateral flow devices utilising peripheral blood samples and selenium dyes or AuNPs conjugated to monoclonal antibodies as sensing units, and an example test is shown in Fig. 5.
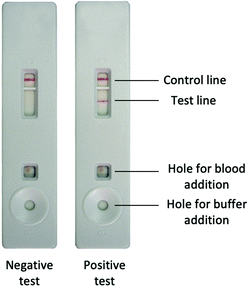 |
| Fig. 5 Image of negative and positive MRDT, taken from WHO documents.91 | |
They are handheld POCT for malaria detection and can differentiate four out of five malaria species (Plasmodium vivax, P. ovale, P. malariae and P. falciparum). The devices are, however, insensitive to P. knowlesi: a simian parasite known to infect humans. MRDTs target a variety of antigen analytes such as histidine-rich protein 2 (HRP-2 is synthesised by all P. falciparum parasites as an abundant water-soluble protein, unlike HRP-1 and HRP-3),92Plasmodium lactate dehydrogenase (pLDH) and Plasmodium aldolase.93,94
Alternatives to MRDTs and other lateral flow systems
MRDTs are less sensitive than alternative non-POCT techniques, such as microscopy of thick blood films and PCR techniques which can detect low parasite concentrations of 50 parasites per μL and 5 parasites per μL, respectively. While WHO does not test MRDTs at concentrations below 200 parasites per μL.30,93 MRDTs are also less capable of determining parasite species beyond P. falciparum and P. vivax, unlike Giesma-stained blood microscopy,95 the most common diagnostic technique, that can have a sensitivity of 95% and a specificity of 98%.30,93,96 Furthermore, this high sensitivity and specificity can be achieved at $0.2 USD per sample at a rate of one sample every 20 minutes.96 However, microscopy is a lab-based technique that requires an expert to carry out the analysis, plus the sensitivity can drop to 10% in the field.97
Due to the COVID-19 pandemic, diagnostics have been rapidly rolled out globally, with rRT-PCR (real-time reverse transcription-polymerase chain reaction) being the gold standard, but turn-around time can be a limiting factor. In July 2020, in the United States, the average wait time for a COVID-19 RT-PCR test was 4 days, and only 37% of people received results within 2 days.98,99 A comparison of rRT-PCR to LFDs showed that lateral flow device sensitivity was > 85% and its specificity > 95%, with a run time of just 15 minutes.100
Several other techniques have emerged to address the time and cost issues of (the very accurate) rRT-PCRs. Loop-mediated isothermal amplification101 (LAMP) can be run on a benchtop with various outputs generating the signal,101,102 in between 20–90 minutes.101 A LAMP device for malaria diagnosis was field-tested in India and Thailand and showed similar sensitivities and specificities to rRT-PCR. LAMP devices have also been developed for SARS-COV-2 and compared well to RT-PCR103 and for malaria diagnosis too.104 Aerts et al.,105 when discussing the cost-effectiveness of LAMP, microscopy and lateral flow tests in diagnosing cutaneous leishmaniasis in Afghanistan, suggested that all these diagnostic platforms have a role to play in different healthcare settings. They found that lateral flow systems reduce overall cost when used outside the clinic. Whereas microscopy is favourable in the clinic except in periods of high demand where LAMP and lateral flow devices are more cost-effective.
MRDT summary
In summary, MRDT lateral flow systems are cost-effective, fast to run and do not require a specialist to perform nor a dedicated laboratory. MRDTs provide an interesting case study in applying POC lateral flow as a low-cost, fast diagnostic versus lab-based and more expensive POC systems in LEDCs. Moreover, studies carried out in MEDCs (the United States of America) have found that MRDTs can outperform blood smear microscopy,106 showing the potential of lateral flow systems in more economically developed countries where patients and health care professionals value short turnaround times.5,12 Therefore, while more expensive reusable POCTs and lab-based diagnostic systems have the potential to provide higher specificities, they do not have the same ease of use, minimal power needs, token maintenance requirements, and low costs of LFDs. It is essential to highlight, though, that some reports of MRDTs suggest they are still costly and challenging to use.107
Crucially, antibody-based MRDTs can degrade when not transported and stored at low temperatures (“cold-chained”), becoming less-sensitive in conditions common in the field108 – highlighting a potential weakness of protein-based LFDs and hence there is interest in developing antibody-free lateral flow devices.
5. Beyond antibodies: glycans as recognition units
Current POC diagnostics, particularly lateral flow systems (above), rely on antibodies as recognition units, owing to their high specificity and established methods for their production and nanoparticle conjugation. However, they are not the only biological recognition motifs; nucleic acids109 and lectins have also been incorporated but are still far less commonly used than antibodies.110 Glycans (carbohydrates) offer an alternative to these recognition units, and their study might bring new opportunities.
Glycans are ubiquitous in biological systems;111 with over half of all human proteins glycosylated.112 Glycosylation covalently adds glycans to proteins, lipids or other molecules (including RNA113) to form glycoconjugates in a dynamic process, regulated by glycosyl transferases and glycosidases.114 As it is not template driven, glycosylation cannot be (readily) predicted from genomic information alone. Glycoconjugates perform a vast range of roles, including; cell signalling,115 hormonal actions,116 cancer progression,117 correct protein folding/structure118,119 and mediating immune responses.120 Many of these processes occur at the glycocalyx (Fig. 6), found on the surface of eukaryotic and bacterial cells, providing the primary interface for cells with their external environment.121 The nature of glycan presentation on mammalian cells is a crucial marker of a disease state,122,123 influenza species specificity124 and influenza A viruses’ zoonotic potential.125 Antigens from P. falciparum have been shown to bind to sialyllactoses and other derivatives found on human erythrocytic glycoprotein A.126
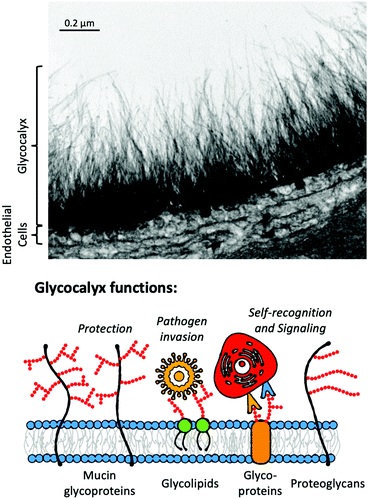 |
| Fig. 6 Electron micrograph of goat coronary capillary stained with Alcian blue and diagrammatic representation of the glycocalyx highlighting glycocalyx functions. Electron micrograph from van den Berg et al. reproduced from ref. 127 (Figure 75.1.) with permission from publisher Cambridge University Press, copyright 2007. | |
Consequently, there is a significant opportunity to target the glycans, or the “glycan-readers” termed lectins, in diagnostics. Lectins are a broad family defined as glycan-binding proteins which are neither enzymes, transporters or antibodies and often have highly conserved carbohydrate-binding domains (CBD).128,129 Examples of lectins (or lectin-like proteins) relevant to healthcare include the Shiga toxins,130 cholera toxin131 and ricin,132 while also being found in snake venoms133 and biocides in marine algae.134 Notably, the use of lectins for staining histology samples has been established for decades.128
The use of lectins in biosensor design has been extensively reviewed.135,136 For example, Damborský et al. have reported a lectin-based LFD for prostate-specific antigen (PSA).110 There is extensive literature on the use of glycans in various applications, which is beyond the scope of this focussed review, but includes; anti-adhesion therapies,137,138 glyconanotechnology,139,140 antimicrobial applications141 and, influenza142 and human immunodeficiency virus (HIV)143 vaccine development. A challenge (and opportunity) lies in the synthesis of oligosaccharides, which inhabit a vast chemical space beyond that of other biological macromolecules of nucleic acid and protein origin.144 However, access to glycans for recognition studies is rapidly expanding,145 thanks to the emergence of automated glycan synthesis146–148 and the use of chemo-enzymatic strategies for greater glycan diversification.149–151
Multivalency and glyco-materials
Glycan–lectin interactions are typically weak with Kd (dissociation constants) ranging from mM to μM,139,152 which is significantly weaker than antibody–ligand interactions (in the nM to pM range). This is overcome in Nature by the presentation of multiple copies of glycans leading to statistical rebinding and chelation, resulting in significant enhancements to binding affinities – known as the “cluster glycoside effect”.153,154 The observed affinity enhancement (per glycan) as valency increases is non-linear and is dependent on the architecture of the glycan, its linker and its accessibility to the target. Due to this, there has been significant interest in using materials chemistry (polymers, particles and surfaces) to present multiple copies of glycans. In the context of diagnostic devices, the cluster glycoside effect is crucial to obtaining the necessary affinity and selectivity, which is not possible using individual glycans. To show the power of the cluster glycoside effect, Fig. 7 shows a summary of 37 glycoconjugates from a previous review.152 This simple representation highlights enhancement effects, ranging from 2 to >10
000-fold increases associated with multivalency. However, it also shows that the simplified assumption that ever larger, higher valency systems lead to increased affinity is not valid: the effects are more subtle. For example, one of the most potent inhibitors of the cholera toxin has just five precisely placed glycans.155 This is crucial to understand how glycosylated gold (or other) nanoparticles may apply in lateral flow diagnostics and how they can be designed more effectively.
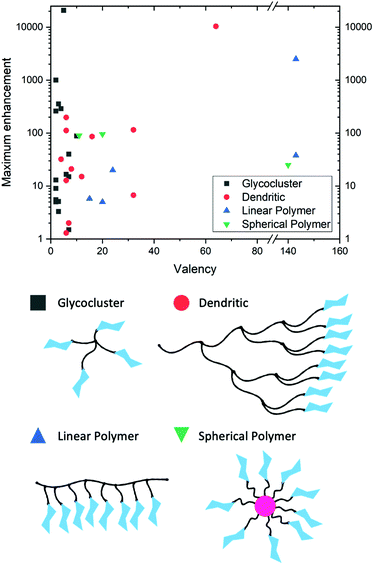 |
| Fig. 7 Graphical representation of a selection of glycoconjugates in “Table 1” from Lundquist et al.152 highlighting the maximum enhancement in binding (corrected for ligand valency) as valency changes. | |
6. Structural and synthetic strategies for glycan presentation
In order to integrate glycans into nanoparticles for LFDs, it is crucial to consider the synthesis (and glycan-binding potential) of multivalent glycosylated materials, which is essential to generate the needed affinity/avidity. Whilst current LFDs use gold cores, other nanomaterials can be employed, and it is crucial to also consider these routes to multivalency, including polymeric methods. It is important to note that beyond multivalency/presentation on polymeric scaffolds, glycan arrays have allowed the probing of multi-antennary/branched glycans.156 The benefits of this approach are well illustrated by Wang et al., who synthesised a series of asymmetrically substituted multi-antennary glycans and found differential binding between the structures despite presenting similar terminal sugars.157
Glycoclusters and dendrimers
Glycoclusters provide well defined and controlled glycan presenting architectures. André et al. designed a series of glycoclusters to increase affinity relative to the free sugar and enhance selectivity towards galectins, in some cases more than halving the IC50 in a tetravalent arrangement.158 More complex glycocluster systems have allowed linker length and valency tuning to target virulence factors such as LecA of Pseudomonas aeruginosa159 and the fimbriae-mediated adhesion of E. coli,160 respectively. Other groups have harnessed non-synthetic cores and directed evolution to design glycoclusters.161
Dendrimers are oligomeric or polymeric structures produced iteratively, forming tree-like, highly branched regular structures and have been used as platforms for glycodendrimers.162,163 A study by Woller et al. measured the binding enhancement realised as dendrimer generation increased in mannose-functionalised dendrimers and saw a 660-fold increase in activity per sugar, versus free sugar, when targeting Concanavalin A (ConA).164 However, glycodendrimers often present a compromise – higher valences are accessible at the cost of decreased structural control and more challenging syntheses, unlike in glycoclusters, where structural control is often favoured over high valences. The high tunability of dendrimers also makes them an attractive approach for sugar presentation, with void spaces in larger dendrimers providing room for molecular cargo, potentially for clinical applications.165,166
Polymeric approaches
Glycopolymers present glycans either on their side chains or end-groups. Due to the relative ease of polymer synthesis, glycopolymers present a convenient route to engineering multivalency.167,168 Controlled radical polymerisation techniques have been crucial to glycopolymer development enabling the synthesis of macromolecules with predictable chain lengths, compositions, and architectures – essential to dissect the impact of multivalency. These techniques include atom transfer radical polymerisation (ATRP),169 reversible addition–fragmentation chain-transfer (RAFT)170 polymerisation, ring-opening metathesis polymerisation (ROMP)171 and nitroxide-mediated polymerisation (NMP).172
Glycopolymers have been reported (non-exhaustively) to bind with enhanced affinity to lectins and as inhibitors of influenza,173,174 to sequester (and inhibit quorum sensing of) bacteria,175 and to prevent bacterial adhesion.176 It is emerging how the polymer backbone, which presents the glycans, can itself play a key role in fine-tuning affinity and selectivity. For example, Kiessling showed that cis-backbones (from ROMP) in mucin mimetics were more potent inhibitors than trans equivalents (Fig. 8A).177 While amide-linked mannose side-chains were found to bind ConA less than ester-linked side-chains, this difference was attributed to subtle mobility differences.178
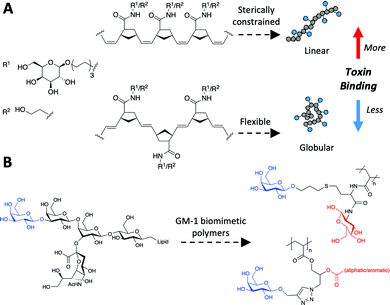 |
| Fig. 8 Macromolecular structure to tune cholera toxin binding. (A) control of cis/trans backbone;177 (B) GM-1 mimetic polymer through polymer/glycan linker modifications.179,180 | |
Macromolecular engineering approaches have also been deployed to engineer specificity. For example, side-chain length (i.e. linker length between backbone and glycan) was varied to enable control over the access of galactose into the relatively deep GM1 binding pocket of the cholera toxin.181,182 While adding branching units on the glycan–polymer linker, the allosteric sialic acid binding site could be targeted to further increase selectivity, Fig. 8B.179,180,183 This approach also utilised multiple different glycans within a single polymer chain and was shown to increase affinity through a range of mechanisms184 beyond just targeting a second binding site – potentially steric shielding too.185
An alternative approach to increase affinity with heterogeneous glycopolymers was introduced by Mahon et al. Polymer–Scaffolded Dynamic Combinatorial Libraries were used to dynamically select for high-affinity pendant groups (Fig. 9).186
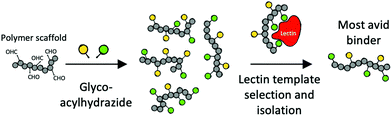 |
| Fig. 9 Dynamic combinatorial selection of avid lectin binders using poly-aldehydes and acylhydrazide glycans.186 | |
Glycopolymers have been deployed, or show potential for, therapeutic roles and biomedical applications, including enhanced immune responses in vaccine candidates187 MRI contrast agents,188,189 drug delivery,190,191 anti-cancer agents192 and to re-program the glycocalyx.193 Chikae et al.194 demonstrated that glycopolymer-coated nanoparticles immobilised on carbon electrodes, can detect amyloid-β, a peptide produced in Alzheimer's disease. Others have shown the potential of glycopolymer-coated AuNPs as anti-cancer195 and transfection agents.196
Each of the above examples shows how the underpinning high-affinity binding from glycopolymers to targets has the potential to be translated into innovative biosensors and diagnostics. The structural versatility of multivalent sugar presenting systems can be used to display a glycan and precisely adjust the nature of the presentation in 3D space197 (as seen for native glycans) providing opportunities to tune the interaction.
7. Glycosylated gold nanoparticles
As discussed in the previous sections, antibody-functionalised gold nanoparticles are the most common core of lateral flow devices. There is limited literature on glyco-gold nanoparticles for LFD (discussed in the section below), but there is a broad literature on the synthesis and use of glycosylated gold nanoparticles, particularly for lectin binding, which is summarised here. Notably, gold nanoparticles are not the only nanoparticles systems used to detect lectins; examples include quantum dots and magnetic nanoparticles.198
Whilst many glycosylated nanoparticle syntheses report direct immobilisation, or short linkers to attach the glycan, this can lead to colloidal stability challenges and irreversible aggregation when in biologically relevant media, such as saline buffers or blood plasma. Hence, the use of polymeric tethers that provide steric stabilisation and act as non-fouling199 interfaces has been explored.
Polymer chains can be added to the surface of AuNPs by three conceptual methods; “grafting to” (a polymer is added to the surface of pre-prepared AuNPs), in situ (a polymer is added to the surface of growing AuNPs) and “grafting from” (polymerisation occurs on CTAs or initiators bound to the pre-prepared AuNP surface), Fig. 10.200 The advantage of “grafting to” is that the precursor polymer can be synthesised and characterised, and installation of the glycan (or other functionality) can be confirmed, which is challenging when added directly to a nanoparticle surface. It is, however, important to note that the diffusion of a macromolecule onto the surface and steric constraints limit the grafting density. This is overcome with “grafting from”, which gives high densities, but it must be isolated from the surface to characterise the polymer. Finally in situ methods, to produce particles in the presence of a polymeric capping agent, can be used which is a balance between the two other approaches.
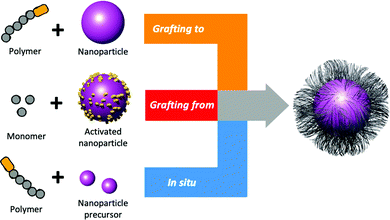 |
| Fig. 10 Representation of the three conceptual methods of polymer grafting to a surface. | |
Poly(ethylene glycol) (PEG) is widely used as a nanoparticle coating due to its low-biofouling, low cytotoxicity and commercial availability. Russel and co-workers used a PEG linker to immobilise a tri-functional sialoside to gold nanoparticles, enabling tuning of avian versus human influenza binding (dictated by the 2,3 versus 2,6 linkages).201 Penadés and co-workers have used PEGylated gold nanoparticles202 to investigate glycosphingolipid mediated carbohydrate–carbohydrate interactions when coupled with an SPR sensor.203
Whilst PEG is widely used, there are thousands of other potential monomeric building blocks for the polymer tether. RAFT polymerisation has attracted interest in this respect, as it installs an α-terminal (latent) thiol on every chain, which is suitable for immobilisation onto gold surfaces.204,205 Cameron and co-workers used poly(galactosides) derived by RAFT for the in situ formation of small (<20 nm) glycosylated AuNPs, capable of lectin recognition and to generate glyco-conjugate cancer vaccines.206 RAFT polymerisation has also been used to make pH responsive207 and thermoresponsive208 glycopolymers on AuNPs. Other (non-RAFT) approaches using disulphide, double-headed ATRP initiators209 or “grafting from” methods have also been used.210,211 The versatility of these approaches enables a mix and match approach whereby polymer ligands can be applied, and the coated gold nanoparticles are easily isolated by centrifugation/washing cycles (Fig. 11).
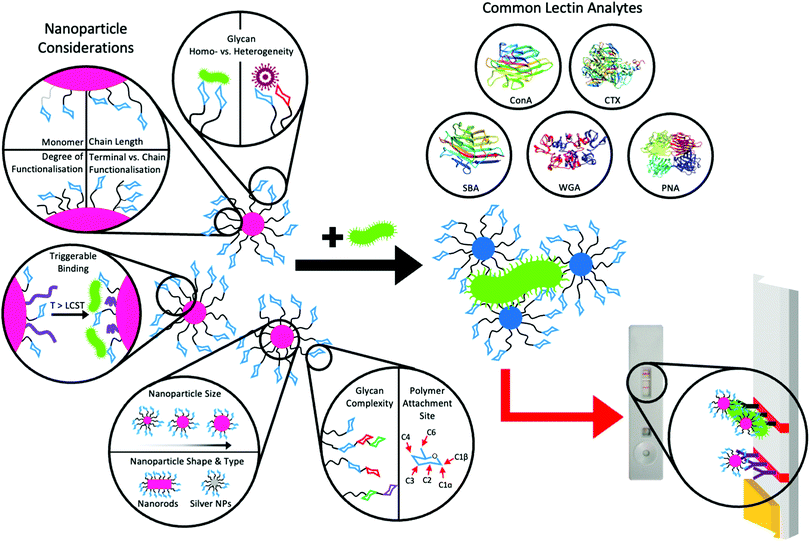 |
| Fig. 11 Major nanoparticle design considerations and common lectin analytes for aggregation assays and potential for use in lateral flow assays. Lectin structures were taken from the Protein Data Bank212 as follows; soybean agglutinin (SBA) – 1SBF,213 concanavalin A (ConA) – 3CNA,214 cholera toxin (CTX) – 1XTC,215 wheat germ agglutinin (WGA) – 2X52216 and peanut agglutinin (PNA) – 2DVD.217 | |
8. Glycosylated AuNPs in sensors and diagnostics – from aggregation to lateral flow
Considering the large body of research on the synthesis of glycosylated materials, and integration into (gold) nanomaterials there has been obvious interest in their deployment for diagnostics and biosensing applications, such as colorimetric bioassays.218 When nanoparticles become localised, the surface plasmon absorption energy maximum of a plasmonic nanoparticle is altered by collective conduction-band electron oscillation i.e. when nanoparticles aggregate there is a strong colour change.51,56 Mirkin et al., demonstrated that oligonucleotide-functionalised AuNPs aggregated in the presence of complementary DNA sticky ends showing how specific DNA sequences can be identified by a simple colour change in solution.219 Due to the fact that lectins often have multiple binding sites (i.e. Concanavalin A (ConA) has 4,220 Soybean agglutinin (SBA) has 4),221 multivalent glycosylated gold nanoparticles have been explored as colourimetric sensors for lectins based on red-blue colour shifts.
Many studies have focused on model (plant) lectins, such as G-rutin functionalised AuNPs (via a catechol) or mannosylated polymers to bind ConA,222,223 galactose-functionalised glycopolymers to bind Ricinus communis agglutinin I (RCAI or RCA120),223 galactose-functionalised glycopolymers made by copper-free click immobilised on core–shell AuNPs to bind peanut agglutinin (PNA)224,225 and lactose-functionalised glycopolymers to bind cholera toxin.226 By using polymeric linkers, nanoparticle (steric) stability in complex media is introduced, avoiding false positives and unwanted aggregation which can occur with direct-surface conjugation.227 The use of polymer tethers also enables additional functionality and properties, such as thermal control of glycan presentation.228
Toyoshima et al.229 synthesised variable density mannose- and N-acetylglucosamine-functionalised glycopolymers immobilised on 15, 40 and 100 nm AuNPs. Aggregation experiments were carried out with target and off-target lectins. The mannose functionalised AuNPs were assayed against ORN178 (an E. coli species specific for α-mannose) and aggregated.229 While Richards et al. showed how fimbrae-differing phenotypes (FimH+ and FimH−) of E.coli can be discriminated by glycosylated AuNPs.230
Aggregation is a crucial consideration of lateral flow (below) but the use of gold nanoparticles for SERS (Surface enhanced Raman scattering) for other device formats is also possible but is beyond the scope of this review. SERS has been demonstrated,231 for example, for both ConA232 (to pM levels) and human galectins.233
Lateral flow
Despite the above, showing the significant body of literature on how multivalent presentation of glycans leads to large binding responses with glycosylated nanoparticles, the incorporation of these into lateral flow glyco-assays (LFGAs) has not been widely explored yet. As glycans can be chemically synthesised, there is no need to raise antibodies against emergent pathogens and the sheer range of tools to alter their presentation make them appealing targets. However, thus far there have been few examples of lateral flow glyco-assays, at the time of writing and to the best of our knowledge, this review covers all published examples (November 2021).
Toyoshima et al.234 utilised α-galactose- and α-mannose- p-acrylamidophenyl pyranosides against lectins in aggregation assays and surface plasmon resonance (SPR) Fig. 12(A). In this work, however, they also showed a flow-through assay, where Shiga toxin-1 was deposited as the test line, facilitating some binding against the galacto–AuNPs Fig. 12(B). This was taken further by Mirua and co-workers using manno-AuNPs to detect ConA. In this case, the glyconanoparticles were used in the mobile phase and a rabbit anti-ConA antibody as the stationary phase test line Fig. 12(C). The dipsticks gave a visible ‘red line’ response to the naked eye. Whilst not a full glyco lateral flow, this study clearly demonstrated that glycoLFDs are possible.235 Further studies varied the mannose density providing optimisation of the output signal.236 A key learning point from this work, is the challenge of maintaining a good signal-to-noise ratio in the final device, with smearing of the nanoparticles as they run up the nitrocellulose due to non-specific interactions. This shows how the tuning of the polymer coating is essential alongside the use of conventional blocking agents such as BSA, to reduce non-specific interactions. The importance of the polymer coating has previously been observed in aggregation assays where a compromise must be reached between stability in aqueous media and rapid optical readout, usually tuned by adjusting polymer length.227,230 Similarly, alterations to the polymer itself i.e., to a more sterically bulky polymer can be used to stabilise AuNPs237 and favour binding but avoid aggregation.238
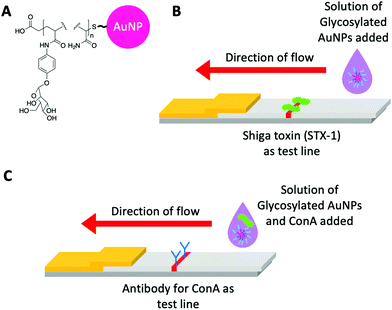 |
| Fig. 12 (A) Representative structure of glycosylated acrylamide coated AuNPs used by Toyoshima et al.234 and Ishii et al.235 (B) Schematic of the flow-through dipstick used by Toyoshima et al.234 and (C) Schematic of the lateral flow dipstick used by Ishii et al.235 | |
Baker et al., reported that SARS-COV-2 (the causative agent of COVID-19) spike protein has affinity towards certain sialic acids,239 (confirmed with microarrays240 and STD NMR241) discovered using glycosylated nanoparticles against spike protein immobilised on a biolayer interferometry sensor. N-Acetyl neuraminic acid functionalised poly(hydroxyethyl acrylamide) were immobilised onto AuNPs and were used to detect a SARS-COV-2 spike protein bearing pseudovirus in what was the first report of a complete lateral flow glyco-assay device (using a BSA–glycoconjugate test-line).239 This was developed further into a flow-through device, whereby viral samples from patient swabs were dried as the test line, so no glycan test line was needed, allowing correct identification of positive/negative patients (Fig. 13A).242
Controlled radical polymerisation (RAFT) was employed to undertake a detailed structure-function study exploring how the polymeric glyco tether impacted the performance of a lectin-detecting lateral flow system.243 Using galactose as the glyco-ligand, the delicate balance between polymer chain length to give specificity, but avoid non-specific binding was revealed, demonstrated by differential binding profiles to SBA and RCA120 using the same glycan on different polymers (Fig. 13A).
Further work has also been carried out by Baker et al. to remove proteins from all sensing components of an LFD i.e., no protein in the test line or on the particle surface. They did this by using poly(vinyl pyrrolidone) anchors decorated with glycans.244 This was further developed by Kim et al. who used glycosaminoglycans from the glycocalyx, to make a protein-free test line for SARS-COV-2.245 This approach of using non-protein-based polymers, both synthetic and biological, to present sugars marks a step change in LFD development and could lead to improved workflows.
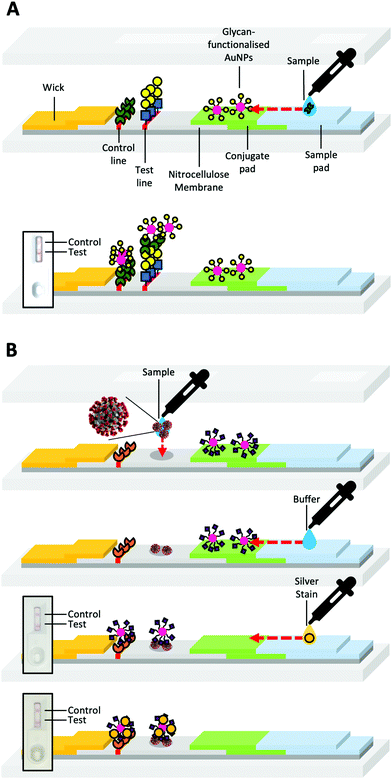 |
| Fig. 13 Representative lateral flow glyco-assay and flow through glyco-assay for sensing for SBA243 (A) and SARS-COV-2242 (B) respectively. | |
9. Conclusions and perspectives
The purpose of this review was to introduce lateral flow diagnostic technology, including its chemical basis, and the real-world application of these devices as low-cost diagnostic tools. We then set out to show that rather than just considering these as immuno-assays, with antibodies as the detection systems, that glyco-assays are also possible by exploiting the diverse range of biochemical interactions driven by glycans. Whilst this is a very new field, with less than 10 publications, this makes it the ideal time to introduce the topic, and in particular highlight the key considerations of multivalent presentation and the importance of how glycans are tethered to the nanoparticle (e.g. gold) core. The potential for glycopolymer-functionalised AuNPs (or other cores) integrated into lateral flow systems, for use as POCT devices is vast, underpinned by the fast-growing field of glycomics. These low-cost, yet potentially robust glycopolymer-based lateral flow diagnostic devices could be ideal for low- and middle-income, and more economically developed countries alike.
As this is a new and exciting interdisciplinary area, spanning materials chemistry, analytical science, biochemistry, and biomedicine there exist several “challenge areas”, or areas where continued scientific exploration is needed to drive the field forward.
• The integration of new core materials, not just those based on gold or plasmonic nanoparticles.
• A greater understanding of how the linker interface impacts the performance of devices and in particular to reduce non-specific binding.
• The development of chemical tools to integrate glycans into particles more efficiently and at scale, including increasing access to rarer (often low quantity) glycans, alongside the development of the corresponding analytical tools.
• Greater integration of multiple recognition modalities and further development of multiplexed tests.
• The integration of glycan-based sensing into the various ‘flavours’ of lateral flow, or flow-through devices. (Fig. 14) both as all glyco systems, or in combination (“hybrid”) with antibodies, nanobodies, nucleic acids or other small molecule binders.
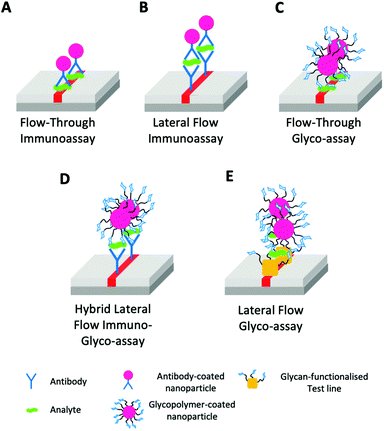 |
| Fig. 14 Range of lateral-flow and related assays classes highlighting the stationary phase (SP) test line and mobile phase (MP). (A) Flow-through immunoassay (SP – analyte & MP – antibody-coated AuNP); (B) Lateral flow immunoassay (SP – antibody & MP – antibody-coated AuNP and analyte); (C) Flow-through glyco-assay (SP – analyte & MP – glycan-coated AuNP); (D) Hybrid lateral flow immuno-glyco-assay (SP – antibody & MP – glycan-coated AuNP and analyte); (E) Lateral flow glyco-assay (SP – glycan-functionalised test line & MP – glycan-coated AuNP and analyte). | |
• The demonstration of function in clinically relevant situations and settings, including in complex media.
• Ensuring the devices are sufficiently sensitive and specific to be deployed for real world applications.
In summary, we have introduced the concept of lateral flow glyco-assays and their chemical basis, whilst laying out potential areas of application and the role of chemistry in developing these. It is the hope of the authors that lateral flow glyco-assays will become useful in the coming years, especially in resource-limited settings.
Literature review methods
A review of the literature was carried out to find papers containing AuNP aggregation assays and glycopolymer use in lateral flow assays.
A review of the literature was conducted through the following databases: Web of Science (all years), Embase (1947–2021) and Scopus (all years). Search date November 2021.
The following search terms were used:
Search 1: ((gold AND nanoparticle*) OR (AuNP*)) AND glycopolymer* AND (aggregat* OR surfac*).
Search 2: (“lateral flow” OR assay*) AND glycopolymer*.
Search 3: (((gold AND nanoparticle*) OR (AuNP*)) AND glycopolymer* AND (aggregat* OR surfac*)) AND ((“lateral flow” OR assay*) AND glycopolymer*).
Search 1 returned 132 results (Embase 17, Scopus 28, Web of Science 87).
Search 2 returned 632 results (Embase 166, Scopus 221, Web of Science 245).
Search 3 returned 17 results (Embase 2, Scopus 6, Web of Science 9).
Abstracts and full-texts were screened to determine relevant reports in the literature.
Conflicts of interest
RF is a shareholder and director of Iceni Glycoscience limited. ANB and MIG are named inventors on a patent relating to underpinning technology reported here.
Acknowledgements
This project has received funding from the European Research Council (ERC) under the European Union's Horizon 2020 research and innovation programme (grant agreement n° 866056) and under the Marie Skłodowska-Curie grant agreement No. 814236. MIG is supported by a Royal Society (Industry Fellowship 191037). This research was funded in whole or in part by the BBSRC [BB/M01116X/1]. For the purpose of open access, the author has applied a Creative Commons Attribution (CC BY) licence to any Author Accepted Manuscript version arising from this submission. This work was also supported by Iceni Glycoscience. Electron micrograph reproduced from van den Berg et al.127 (figure 75.1.) under licence from Cambridge University Press, PLSclear Ref No: 53530
References
- S. Leekha, C. L. Terrell and R. S. Edson, General Principles of Antimicrobial Therapy, Mayo Clin. Proc., 2011, 86(2), 156–167 CrossRef PubMed.
- L. C. Clark and C. Lyons, Electrode Systems for Continuous Monitoring in Cardiovascular Surgery, Ann. N. Y. Acad. Sci., 1962, 102, 29–45 CrossRef CAS PubMed.
-
M. M. Crane and M. V. Organon, Diagnostic Test Device, US3579306A, 1969 Search PubMed.
- P. K. Drain, E. P. Hyle, F. Noubary, K. A. Freedberg, D. Wilson, W. R. Bishai, W. Rodriguez and I. V. Bassett, Diagnostic Point-of-Care Tests in Resource-Limited Settings, Lancet Infect. Dis., 2014, 14, 239–249 CrossRef PubMed.
- A. St John and C. P. Price, Point-of-Care Testing Technologies Existing and Emerging Technologies for Point-of-Care Testing, Clin. Biochem. Rev., 2014, 35(3), 155–167 Search PubMed.
-
C. P. Price; A. St John and J. Hicks, Point-of-Care Testing, American Association for Clinical Chemistry, Washington D.C., 2nd edn, 2004 Search PubMed.
- S. S. Ehrmeyer and R. H. Laessig, Point-of-Care Testing, Medical Error, and Patient Safety: A 2007 Assessment, Clin. Chem. Lab. Med., 2007, No. 45, 766–773 Search PubMed.
- N. P. Pai, C. Vadnais, C. Denkinger, N. Engel and M. Pai, Point-of-Care Testing for Infectious Diseases: Diversity, Complexity, and Barriers in Low-And Middle-Income Countries The Promise of Point-of-Care Testing, PLoS Med., 2012, 9(9), e1001306 CrossRef PubMed.
- N. P. Pai and M. Pai, Point-of-Care Diagnostics for HIV and Tuberculosis: Landscape, Pipeline, and Unmet Needs, Discovery Med., 2012, 13, 34–45 Search PubMed.
- H. Hsieh, J. Dantzler and B. Weigl, Analytical Tools to Improve Optimization Procedures for Lateral Flow Assays, Diagnostics, 2017, 7(2), 29 CrossRef CAS PubMed.
- T. Liang, R. Robinson, J. Houghtaling, G. Fridley, S. A. Ramsey and E. Fu, Investigation of Reagent Delivery Formats in a Multivalent Malaria Sandwich Immunoassay and Implications for Assay Performance, Anal. Chem., 2016, 88(4), 2311–2320 CrossRef CAS PubMed.
- Y.-H. Hsieh, C. A. Gaydos, M. T. Hogan, O. M. Uy, J. Jackman, M. Jett-Goheen, A. Albertie, D. T. Dangerfield, C. R. Neustadt, Z. S. Wiener and A. M. Rompalo, What Qualities Are Most Important to Making a Point of Care Test Desirable for Clinicians and Others Offering Sexually Transmitted Infection Testing?, PLoS One, 2011, 6(4), e19263 CrossRef CAS PubMed.
-
C. S. Kosack, A.-L. Page and P. R. Klatser, Bulletin of the World Health Organization: A Guide to Aid the Selection of Diagnostic Tests, 2017.
- A. El-Osta, M. Woringer, E. Pizzo, T. Verhoef, C. Dickie, M. Z. Ni, J. R. Huddy, M. Soljak, G. B. Hanna and A. Majeed, Does Use of Point-of-Care Testing Improve Cost-Effectiveness of the NHS Health Check Programme in the Primary Care Setting? A Cost-Minimisation Analysis, BMJ Open, 2017, 7(8), e015494 CrossRef PubMed.
- P. Bhatnagar, K. Wickramasinghe, J. Williams, M. Rayner and N. Townsend, The Epidemiology of Cardiovascular Disease in the UK 2014, Heart, 2015, 101(15), 1182–1189 CrossRef CAS PubMed.
- Q. Tan, Z. J. L. Hildon, S. Singh, J. Jing, T. L. Thein, R. Coker, H. J. M. Vrijhoef and Y. S. Leo, Comparing Patient and Healthcare Worker Experiences during a Dengue Outbreak in Singapore: Understanding the Patient Journey and the Introduction of a Point-of-Care Test (POCT) toward Better Care Delivery, BMC Infect. Dis., 2017, 17(1), 503–519 CrossRef PubMed.
- Z. Li, Y. Yi, X. Luo, N. Xiong, Y. Liu, S. Li, R. Sun, Y. Wang, B. Hu, W. Chen, Y. Zhang, J. Wang, B. Huang, Y. Lin, J. Yang, W. Cai, X. Wang, J. Cheng, Z. Chen, K. Sun, W. Pan, Z. Zhan, L. Chen and F. Ye, Development and Clinical Application of A Rapid IgM-IgG Combined Antibody Test for SARS-CoV-2 Infection Diagnosis, J. Med. Virol., 2020, jmv.25727 Search PubMed.
- G. Kolifarhood, M. Aghaali, H. Mozafar Saadati, N. Taherpour, S. Rahimi, N. Izadi and S. S. Hashemi Nazari, Epidemiological and Clinical Aspects of COVID-19; a Narrative Review, Arch. Acad. Emerg. Med., 2020, 8(1), e41 Search PubMed.
- G. A. Posthuma-Trumpie, J. Korf and A. Van Amerongen, Lateral Flow (Immuno)Assay: Its Strengths, Weaknesses, Opportunities and Threats. A Literature Survey, Anal. Bioanal. Chem., 2009, 393(2), 569–582 CrossRef CAS PubMed.
- J. H. W. Leuvering, P. J. H. M. Thal, M. Waart and A. H. W. M. van der; Schuurs, Sol Particle Immunoassay (SPIA), J. Immunoassay, 1980, 1(1), 77–91 CrossRef CAS PubMed.
- E. C. Schramm, N. R. Staten, Z. Zhang, S. S. Bruce, C. Kellner, J. P. Atkinson, V. C. Kyttaris, G. C. Tsokos, M. Petri, E. Sander Connolly and P. K. Olson, A Quantitative Lateral Flow Assay to Detect Complement Activation in Blood, Anal. Biochem., 2015, 477, 78–85 CrossRef CAS PubMed.
- L. Liu, C. Peng, Z. Jin and C. Xu, Development and Evaluation of a Rapid Lateral Flow Immunochromatographic Strip Assay for Screening 19-Nortestosterone, Biomed. Chromatogr., 2007, 21(8), 861–866 CrossRef CAS PubMed.
- N. Nagatani, T. Yuhi, M. Chikae, K. Kerman, T. Endo, Y. Kobori, M. Takata, H. Konaka, M. Namiki, H. Ushijima, Y. Takamura and E. Tamiya, A Sensitive Immunochromatographic Assay Using Gold Nanoparticles for the Semiquantitative Detection of Prostate-Specific Antigen in Serum, Nanobiotechnology, 2006, 2(3–4), 79–86 CrossRef CAS.
- S. Buhrer-Sekula, H. L. Smits, G. C. Gussenhoven, J. van Leeuwen, S. Amador, T. Fujiwara, P. R. Klatser and L. Oskam, Simple and Fast Lateral Flow Test for Classification of Leprosy Patients and Identification of Contacts with High Risk of Developing Leprosy, J. Clin. Microbiol., 2003, 41(5), 1991–1995 CrossRef CAS PubMed.
- J. N. Jarvis, A. Percival, S. Bauman, J. Pelfrey, G. Meintjes, G. N. Williams, N. Longley, T. S. Harrison and T. R. Kozel, Evaluation of a Novel Point-of-Care Cryptococcal Antigen Test on Serum, Plasma, and Urine from Patients with HIV-Associated Cryptococcal Meningitis, Clin. Infect. Dis., 2011, 53, 1019–1023 CrossRef CAS PubMed.
- V. S. Vaidya, G. M. Ford, S. S. Waikar, Y. Wang, M. B. Clement, V. Ramirez, W. E. Glaab, S. P. Troth, F. D. Sistare, W. C. Prozialeck, J. R. Edwards, N. A. Bobadilla, S. C. Mefferd and J. V. Bonventre, A Rapid Urine Test for Early Detection of Kidney Injury, Kidney Int., 2009, 76, 108–114 CrossRef CAS PubMed.
- A.-L. Page, K. P. Alberti, V. Mondonge, J. Rauzier, M.-L. Quilici and P. J. Guerin, Evaluation of a Rapid Test for the Diagnosis of Cholera in the Absence of a Gold Standard, PLoS One, 2012, 7(5), e37360 CrossRef CAS PubMed.
- S. H. Ang, M. Rambeli, T. M. Thevarajah, Y. B. Alias and S. M. Khor, Quantitative, Single-Step Dual Measurement of Hemoglobin A1c and Total Hemoglobin in Human Whole Blood Using a Gold Sandwich Immunochromatographic Assay for Personalized Medicine, Biosens. Bioelectron., 2016, No. 78, 187–193 CrossRef PubMed.
- R. E. Biagini, D. L. Sammons, J. P. Smith, B. A. MacKenzie, C. A. F. Striley, J. E. Snawder, S. A. Robertson and C. P. Quinn, Rapid, Sensitive, and Specific Lateral-Flow Immunochromatographic Device to Measure Anti-Anthrax Protective Antigen Immunoglobulin G in Serum and Whole Blood, Clin. Vaccine Immunol., 2006, 12, 541–546 CrossRef PubMed.
- World Health Organisation, Malaria Rapid Diagnostic Test Performance: Summary Results of WHO Product Testing of Malaria RDTs: Rounds 1–6 (2008–2015), 2015.
- R. Banerjee and A. Jaiswal, Recent Advances in Nanoparticle-Based Lateral Flow Immunoassay as a Point-of-Care Diagnostic Tool for Infectious Agents and Diseases, Analyst, 2018, pp. 1970–1996 RSC.
- S. Gentili, R. Solimini, R. Tittarelli, G. Mannocchi and F. P. Busardò, A Study on the Reliability of an On-Site Oral Fluid Drug Test in a Recreational Context, J. Anal. Methods Chem., 2016, 1–10 Search PubMed.
- T. R. Arkell, R. C. Kevin, J. Stuart, N. Lintzeris, P. S. Haber, J. G. Ramaekers and I. S. McGregor, Detection of Δ9 THC in Oral Fluid Following Vaporized Cannabis with Varied Cannabidiol (CBD) Content: An Evaluation of Two Point-of-collection Testing Devices, Drug Test. Anal., 2019, 11(10), 1486–1497 CrossRef CAS PubMed.
-
L. Huang, S. Tian, W. Zhao, K. Liu, X. Ma and J. Guo, Multiplexed Detection of Biomarkers in Lateral-Flow Immunoassays, The Royal Society of Chemistry, 2020, vol. 145, pp. 2828–2840 Search PubMed.
- M. Z. Zheng, J. L. Richard and J. Binder, A Review of Rapid Methods for the Analysis of Mycotoxins, Mycopathologia, 2006, 161(5), 261–273 CrossRef CAS PubMed.
-
M. A. Mansfield, Nitrocellulose Membranes for Lateral Flow Immunoassays: A Technical Treatise, In Lateral Flow Immunoassay, 2009, pp. 95–114 Search PubMed.
- E. B. Bahadır and M. K. Sezgintürk, Lateral Flow Assays: Principles, Designs and Labels, TrAC, Trends Anal. Chem., 2016, 286–306 CrossRef.
- C. A. Holstein, A. Chevalier, S. Bennett, C. E. Anderson, K. Keniston, C. Olsen, B. Li, B. Bales, D. R. Moore, E. Fu, D. Baker and P. Yager, Immobilizing Affinity Proteins to Nitrocellulose: A Toolbox for Paper-Based Assay Developers, Anal. Bioanal. Chem., 2016, 408(5), 1335–1346 CrossRef CAS PubMed.
- M. Hiratsuka, A. Ebisawa, Y. Matsubara, S. Kure, Y. Konno, T. Sasaki and M. Mizugaki, Genotyping of Single Nucleotide Polymorphisms (SNPs) Influencing Drug Response by Competitive Allele-Specific Short Oligonucleotide Hybridization (CASSOH) with Immunochromatographic Strip, Drug Metab. Pharmacokinet., 2004, 19(4), 303–307 CrossRef CAS PubMed.
- J. J. Storhoff, R. Elghanian, R. C. Mucic, C. A. Mirkin and R. L. Letsinger, One-Pot Colorimetric Differentiation of Polynucleotides with Single Base Imperfections Using Gold Nanoparticle Probes, J. Am. Chem. Soc., 1998, 120(9), 1959–1964 CrossRef CAS.
- S. Aoyama, Y. Akiyama, K. Monden, M. Yamada and M. Seki, Thermally Imprinted Microcone Structure-Assisted Lateral-Flow Immunoassay Platforms for Detecting Disease Marker Proteins, Analyst, 2019, 144(5), 1519–1526 RSC.
- A. H. C. Ng, U. Uddayasankar and A. R. Wheeler, Immunoassays in Microfluidic Systems, Anal. Bioanal. Chem., 2010, 397(3), 991–1007 CrossRef CAS PubMed.
- L. Magro, B. Jacquelin, C. Escadafal, P. Garneret, A. Kwasiborski, J.-C. Manuguerra, F. Monti, A. Sakuntabhai, J. Vanhomwegen, P. Lafaye and P. Tabeling, Paper-Based RNA Detection and Multiplexed Analysis for Ebola Virus Diagnostics, Sci. Rep., 2017, 7(1), 1347 CrossRef PubMed.
- M. Srisa-Art, K. E. Boehle, B. J. Geiss and C. S. Henry, Highly Sensitive Detection of Salmonella Typhimurium Using a Colorimetric Paper-Based Analytical Device Coupled with Immunomagnetic Separation, Anal. Chem., 2018, 90(1), 1035–1043 CrossRef CAS PubMed.
- E. Gabriel, P. Garcia, F. Lopes and W. Coltro, Paper-Based Colorimetric Biosensor for Tear Glucose Measurements, Micromachines, 2017, 8(4), 104 CrossRef.
- K. Kaneko, M. Hara, T. Nishino and T. Maruyama, One-Step Biotinylation of Cellulose Paper by Polymer Coating to Prepare a Paper-Based Analytical Device, Anal. Chem., 2020, 92(2), 1978–1987 CrossRef CAS PubMed.
- A. Yu, J. Shang, F. Cheng, B. A. Paik, J. M. Kaplan, R. B. Andrade and D. M. Ratner, Biofunctional Paper via the Covalent Modification of Cellulose, Langmuir, 2012, 28(30), 11265–11273 CrossRef CAS PubMed.
- R. Tanaka, T. Yuhi, N. Nagatani, T. Endo, K. Kerman, Y. Takamura and E. Tamiya, A Novel Enhancement Assay for Immunochromatographic Test Strips Using Gold Nanoparticles, Anal. Bioanal. Chem., 2006, 385(8), 1414–1420 CrossRef CAS PubMed.
- J.-H. Lee, H. S. Seo, J.-H. Kwon, H.-T. Kim, K. C. Kwon, S. J. Sim, Y. J. Cha and J. Lee, Multiplex Diagnosis of Viral Infectious Diseases (AIDS, Hepatitis C, and Hepatitis A) Based on Point of Care Lateral Flow Assay Using Engineered Proteinticles, Biosens. Bioelectron., 2015, 69, 213–225 CrossRef CAS PubMed.
- M. De Paschale, Serological Diagnosis of Epstein-Barr Virus Infection: Problems and Solutions, World J. Virol., 2012, 1(1), 31 CrossRef PubMed.
- P. D. Howes, R. Chandrawati and M. M. Stevens, Colloidal Nanoparticles as Advanced Biological Sensors, Science, 2014, 346(6205), 53–66 CrossRef CAS PubMed.
- E. C. Dreaden, A. M. Alkilany, X. Huang, C. J. Murphy and M. A. El-Sayed, The Golden Age: Gold Nanoparticles for Biomedicine, Chem. Soc. Rev., 2012, 2740–2779 RSC.
- E. Roduner, Size Matters: Why Nanomaterials Are Different, Chem. Soc. Rev., 2006, 35, 583–592 RSC.
- G. Mie, Beiträge Zur Optik Trüber Medien, Speziell Kolloidaler Metallösungen, Ann. Phys., 1908, 330(3), 377–445 CrossRef.
- M. X. Faraday, The Bakerian Lecture. —Experimental Relations of Gold (and Other Metals) to Light, Philos. Trans. R. Soc. London, 1857, 147, 145–181 CrossRef.
- S. Eustis and M. A. El-Sayed, Why Gold Nanoparticles Are More Precious than Pretty Gold: Noble Metal Surface Plasmon Resonance and Its Enhancement of the Radiative and Nonradiative Properties of Nanocrystals of Different Shapes, Chem. Soc. Rev., 2006, 35, 209–217 RSC.
- V. Amendola, R. Pilot, M. Frasconi, O. M. Maragò and M. A. Iatì, Surface Plasmon Resonance in Gold Nanoparticles: A Review, J. Phys.: Condens. Matter, 2017, 29(20), 203002 CrossRef PubMed.
-
U. Kreibig and M. Vollmer, Optical Properties of Metal Clusters; Springer Series in Materials Science, Springer Berlin Heidelberg, Berlin, Heidelberg, 1995, vol. 25 Search PubMed.
- X. Liu, M. Atwater, J. Wang and Q. Huo, Extinction Coefficient of Gold Nanoparticles with Different Sizes and Different Capping Ligands, Colloids Surf., B, 2007, 58(1), 3–7 CrossRef CAS PubMed.
- D. Paramelle, A. Sadovoy, S. Gorelik, P. Free, J. Hobley and D. G. Fernig, A Rapid Method to Estimate the Concentration of Citrate Capped Silver Nanoparticles from UV-Visible Light Spectra, Analyst, 2014, 139(19), 4855–4861 RSC.
- M. Taniguchi and J. S. Lindsey, Database of Absorption and Fluorescence Spectra of >300 Common Compounds for Use in PhotochemCAD, Photochem. Photobiol., 2018, 94(2), 290–327 CrossRef CAS PubMed.
- J. Turkevich, P. C. Stevenson and J. Hillier, A Study of the Nucleation and Growth Processes in the Synthesis of Colloidal Gold, Discuss. Faraday Soc., 1951, 11, 55 RSC.
- C. Ziegler and A. Eychmüller, Seeded Growth Synthesis of Uniform Gold Nanoparticles with Diameters of 15–300 Nm, J. Phys. Chem. C, 2011, 115(11), 4502–4506 CrossRef CAS.
- N. G. Bastús, J. Comenge and V. Puntes, Kinetically Controlled Seeded Growth Synthesis of Citrate-Stabilized Gold Nanoparticles of up to 200 Nm: Size Focusing
versus Ostwald Ripening, Langmuir, 2011, 27(17), 11098–11105 CrossRef PubMed.
-
M. Venkataramasubramani and L. Tang, Development of Gold Nanorod Lateral Flow Test for Quantitative Multi-Analyte Detection. In IFMBE Proceedings; 2009, pp. 199–202.
- R. Karamanska, B. Mukhopadhyay, D. A. Russell and R. A. Field, Thioctic Acid Amides: Convenient Tethers for Achieving Low Nonspecific Protein Binding to Carbohydrates Presented on Gold Surfaces, Chem. Commun., 2005, 3334 RSC.
- X. Lu, T. Mei, Q. Guo, W. Zhou, X. Li, J. Chen, X. Zhou, N. Sun and Z. Fang, Improved Performance of Lateral Flow Immunoassays for Alpha-Fetoprotein and Vanillin by Using Silica Shell-Stabilized Gold Nanoparticles, Mikrochim. Acta, 2018, 186(1), 2 CrossRef PubMed.
- J. Wu, J. Ma, H. Wang, D. Qin, L. An, Y. Ma, Z. Zheng, X. Hua, T. Wang and X. Wu, Rapid and Visual Detection of Benzothiostrobin Residue in Strawberry Using Quantum Dot-Based Lateral Flow Test Strip, Sens. Actuators, B, 2019, 283, 222–229 CrossRef CAS.
- L. Yu, P. Li, X. Ding and Q. Zhang, Graphene Oxide and Carboxylated Graphene Oxide: Viable Two-Dimensional Nanolabels for Lateral Flow Immunoassays, Talanta, 2017, 165, 167–175 CrossRef CAS PubMed.
- L. Yao, J. Teng, M. Zhu, L. Zheng, Y. Zhong, G. Liu, F. Xue and W. Chen, MWCNTs Based High Sensitive Lateral Flow Strip Biosensor for Rapid Determination of Aqueous Mercury Ions, Biosens. Bioelectron., 2016, 85, 331–336 CrossRef CAS PubMed.
- L. Dou, T. Bu, W. Zhang, B. Zhao, Q. Yang, L. Huang, S. Li, C. Yang, J. Wang and D. Zhang, Chemical-Staining Based Lateral Flow Immunoassay: A Nanomaterials-Free and Ultra-Simple Tool for a Small Molecule Detection, Sens. Actuators, B, 2019, 279, 427–432 CrossRef CAS.
- A. E. Urusov, A. v Zherdev and B. B. Dzantiev, Towards Lateral Flow Quantitative Assays: Detection Approaches, Biosensors, 2019, 9(3), 89 CrossRef CAS PubMed.
- R. W. Peeling and D. Mabey, Point-of-Care Tests for Diagnosing Infections in the Developing World, Clin. Microbiol. Infect., 2010, 16, 1062–1069 CrossRef CAS PubMed.
- C. M. Morel, Neglected Diseases: Under-funded Research and Inadequate Health Interventions, EMBO Rep., 2003, 4, S35–S38 CrossRef CAS PubMed.
- D. J. Weiss, T. C. D. Lucas, M. Nguyen, A. K. Nandi, D. Bisanzio, K. E. Battle, E. Cameron, K. A. Twohig, D. A. Pfeffer, J. A. Rozier, H. S. Gibson, P. C. Rao, D. Casey, A. Bertozzi-Villa, E. L. Collins, U. Dalrymple, N. Gray, J. R. Harris, R. E. Howes, S. Y. Kang, S. H. Keddie, D. May, S. Rumisha, M. P. Thorn, R. Barber, N. Fullman, C. K. Huynh, X. Kulikoff, M. J. Kutz, A. D. Lopez, A. H. Mokdad, M. Naghavi, G. Nguyen, K. A. Shackelford, T. Vos, H. Wang, D. L. Smith, S. S. Lim, C. J. L. Murray, S. Bhatt, S. I. Hay and P. W. Gething, Mapping the Global Prevalence, Incidence, and Mortality of Plasmodium Falciparum, 2000-17: A Spatial and Temporal Modelling Study, Lancet, 2019, 394(10195), 322–331 CrossRef.
- World Health Organization, Global Report on Antimalarial Drug Efficacy and Drug Resistance: 2000–2010, 2010.
- A. M. Dondorp, F. Nosten, P. Yi, D. Das, A. P. Phyo, J. Tarning, K. M. Lwin, F. Ariey, W. Hanpithakpong, S. J. Lee, P. Ringwald, K. Silamut, M. Imwong, K. Chotivanich, P. Lim, T. Herdman, S. S. An, S. Yeung, P. Singhasivanon, N. P. J. Day, N. Lindegardh, D. Socheat and N. J. White, Artemisinin Resistance in Plasmodium Falciparum Malaria, N. Engl. J. Med., 2009, 361(5), 455–467 CrossRef CAS PubMed.
- R. M. Fairhurst and A. M. Dondorp, Artemisinin-Resistant Plasmodium Falciparum Malaria, Microbiol. Spectrum, 2016, 4(3) DOI:10.1128/microbiolspec.EI10-0013-2016.
- S. D. Otienoburu, I. Suay, S. Garcia, N. V. Thomas, S. Srisutham, A. Björkman and G. S. Humphreys, An Online Mapping Database of Molecular Markers of Drug Resistance in Plasmodium Falciparum: The ACT Partner Drug Molecular Surveyor, Malar. J., 2019, 18(1), 12–21 CrossRef PubMed.
- D. Shahinas, R. Lau, K. Khairnar, D. Hancock and D. R. Pillai, Artesunate Misuse and Plasmodium Falciparum Malaria in Traveler Returning from Africa, Emerging Infect. Dis., 2010, 16(10), 1608–1610 CrossRef.
- A. A. A. Elmannan, K. A. Elmardi, Y. A. Idris, J. M. Spector, N. A. Ali and E. M. Malik, Anti-Malarial Prescribing Practices in Sudan Eight Years after Introduction of Artemisinin-Based Combination Therapies and Implications for Development of Drug Resistance, BMC Pharmacol. Toxicol., 2015, 360(5), 491–499 Search PubMed 2015 16:1.
-
World Health Organization, Guidelines for the Treatment of Malaria, World Health Organization, 2nd edn, 2010 Search PubMed.
- J. D. Njau, C. A. Goodman, S. P. Kachur, J. Mulligan, J. S. Munkondya, N. Mchomvu, S. Abdulla, P. Bloland and A. Mills, The Costs of Introducing Artemisinin-Based Combination Therapy: Evidence from District-Wide Implementation in Rural Tanzania, Malar. J., 2008, 7(1), 4–17 CrossRef PubMed.
- P. Olliaro, W. R. J. Taylor, J. Rigal, J. Mulligan, J. S. Munkondya, N. Mchomvu, S. Abdulla, P. Bloland and A. Mills, Controlling Malaria: Challenges and Solutions, Trop. Med. Int. Health, 2001, 6(11), 922–927 CrossRef CAS PubMed.
- N. J. White, Antimalarial Drug Resistance, J. Clin. Invest., 2004, 113(8), 1084–1092 CrossRef CAS PubMed.
- I. J. Ezennia, S. O. Nduka and O. I. Ekwunife, Cost Benefit Analysis of Malaria Rapid Diagnostic Test: The Perspective of Nigerian Community Pharmacists, Malar. J., 2017, 16(1), 7–16 CrossRef PubMed.
- UNICEF. Malaria Rapid Diagnostic Tests Market & Supply Update; 2016.
- D. J. Weiss, T. C. D. Lucas, M. Nguyen, A. K. Nandi, D. Bisanzio, K. E. Battle, E. Cameron, K. A. Twohig, D. A. Pfeffer, J. A. Rozier, H. S. Gibson, P. C. Rao, D. Casey, A. Bertozzi-Villa, E. L. Collins, U. Dalrymple, N. Gray, J. R. Harris, R. E. Howes, S. Y. Kang, S. H. Keddie, D. May, S. Rumisha, M. P. Thorn, R. Barber, N. Fullman, C. K. Huynh, X. Kulikoff, M. J. Kutz, A. D. Lopez, A. H. Mokdad, M. Naghavi, G. Nguyen, K. A. Shackelford, T. Vos, H. Wang, D. L. Smith, S. S. Lim, C. J. L. Murray, S. Bhatt, S. I. Hay and P. W. Gething, Mapping the Global Prevalence, Incidence, and Mortality of Plasmodium Falciparum, 2000-17: A Spatial and Temporal Modelling Study, Lancet, 2019, 394(10195), 322–331 CrossRef.
- S. I. Hay and R. W. Snow, The Malaria Atlas Project: Developing Global Maps of Malaria Risk, PLoS Med., 2006, 3(12), e473 CrossRef PubMed.
- S. I. Hay and R. W. Snow, The Malaria Atlas Project: Developing Global Maps of Malaria Risk, PLoS Med., 2006, 3(12), e473 CrossRef PubMed.
- World Health Organization, How To Use a Rapid Diagnostic Test (RDT), 2008.
- E. P. Rock, K. Marsh, A. J. Saul, T. E. Wellems, D. W. Taylor, W. L. Maloy and R. J. Howard, Comparative Analysis of the Plasmodium Falciparum Histidine-Rich Proteins HRP-I, HRP-II and HRP-III in Malaria Parasites of Diverse Origin, Parasitology, 1987, 95(Pt 2), 209–227 CrossRef CAS PubMed.
- A. Moody, Rapid Diagnostic Tests for Malaria Parasites, Clin. Microbiol. Rev., 2002, 15(1), 66–78 CrossRef CAS PubMed.
- B. E. Barber, M. J. Grigg, T. William, T. W. Yeo and N. M. Anstey, The Treatment of Plasmodium Knowlesi Malaria, Trends Parasitol., 2017, 33(3), 242–253 CrossRef CAS PubMed.
-
J. Field; P. Shute and A. Sandosham, The Microscopic Diagnosis of Human Malaria, Kuala Lumpur, 1956.
- M. S. Cordray and R. R. Richards-Kortum, Review: Emerging Nucleic Acid-Based Tests for Point-of-Care Detection of Malaria, Am. J. Trop. Med. Hyg., 2012, 87(2), 223–230 CrossRef CAS PubMed.
- R. E. Coleman, N. Maneechai, N. Rachaphaew, C. Kumpitak, R. S. Miller, V. Soyseng, K. Thimasarn and J. Sattabongkot, Comparison of Field and Expert Laboratory Microscopy for Active Surveillance for Asymptomatic Plasmodium Falciparum and Plasmodium Vivax in Western Thailand, Am. J. Trop. Med. Hyg., 2002, 67, 141–144 CrossRef PubMed.
-
D. Lazer, M. A. Baum, A. Quintana, J. Druckman, J. Della and M. Simonson, The State Of The Nation: A 50-State Covid-19 Survey – Report #8: Failing the Test: Waiting Times for COVID Diagnostic Tests Across The U.S, 2020.
- P. Raftery, O. Condell, C. Wasunna, J. Kpaka, R. Zwizwai, M. Nuha, M. Fallah, M. Freeman, V. Harris, M. Miller, A. Baller, M. Massaquoi, V. Katawera, J. Saindon, P. Bemah, E. Hamblion, E. Castle, D. Williams, A. Gasasira and T. Nyenswah, Establishing Ebola Virus Disease (EVD) Diagnostics Using GeneXpert Technology at a Mobile Laboratory in Liberia: Impact on Outbreak Response, Case Management and Laboratory Systems Strengthening, PLoS Neglected Trop. Dis., 2018, 12(1), e0006135 CrossRef PubMed.
- J. C. Phan, J. Pettitt, J. S. George, L. S. Fakoli, F. M. Taweh, S. L. Bateman, R. S. Bennett, S. L. Norris, D. A. Spinnler, G. Pimentel, P. K. Sahr, F. K. Bolay and R. J. Schoepp, Lateral Flow Immunoassays for Ebola Virus Disease Detection in Liberia, J. Infect. Dis., 2016, 214(suppl 3), S222–S228 CrossRef CAS PubMed.
- A. Niemz, T. M. Ferguson and D. S. Boyle, Point-of-Care Nucleic Acid Testing for Infectious Diseases, Trends Biotechnol., 2011, 29(5), 240–250 CrossRef CAS PubMed.
- Y. Cao, L. Wang, L. Duan, J. Li, J. Ma, S. Xie, L. Shi and H. Li, Development of a Real-Time Fluorescence Loop-Mediated Isothermal Amplification Assay for Rapid and Quantitative Detection of Ustilago Maydis, Sci. Rep., 2017, 7(1), 13394 CrossRef PubMed.
- G.-S. Park, K. Ku, S.-H. Baek, S.-J. Kim, S. I. Kim, B.-T. Kim and J.-S. Maeng, Development of Reverse Transcription Loop-Mediated Isothermal Amplification Assays Targeting Severe Acute Respiratory Syndrome Coronavirus 2 (SARS-CoV-2), J. Mol. Diagn., 2020, 22(6), 729–735 CrossRef CAS PubMed.
- J. C. Patel, N. W. Lucchi, P. Srivastava, J. T. Lin, R. Sug-aram, S. Aruncharus, P. K. Bharti, M. M. Shukla, K. Congpuong, W. Satimai, N. Singh, V. Udhayakumar and S. R. Meshnick, Field Evaluation of a Real-Time Fluorescence Loop-Mediated Isothermal Amplification Assay, RealAmp, for the Diagnosis of Malaria in Thailand and India, J. Infect. Dis., 2014, 210(8), 1180–1187 CrossRef PubMed.
- C. Aerts, M. Vink, S. J. Pashtoon, S. Nahzat, A. Picado, I. Cruz and E. Sicuri, Cost Effectiveness of New Diagnostic Tools for Cutaneous Leishmaniasis in Afghanistan, Appl. Health Econ. Health Policy, 2019, 17(2), 213–230 CrossRef PubMed.
- W. M. Stauffer, C. P. Cartwright, D. A. Olson, B. A. Juni, C. M. Taylor, S. H. Bowers, K. L. Hanson, J. E. Rosenblatt and D. R. Boulware, Diagnostic Performance of Rapid Diagnostic Tests versus Blood Smears for Malaria in US Clinical Practice, Clin. Infect. Dis., 2009, 49(6), 908–913 CrossRef PubMed.
- M. Hawkes, J. P. Katsuva and C. K. Masumbuko, Use and Limitations of Malaria Rapid Diagnostic Testing by Community Health Workers in War-Torn Democratic Republic of Congo, Malar. J., 2009, 8(1), 308 CrossRef PubMed.
- P. Jorgensen, L. Chanthap, A. Rebueno, R. Tsuyuoka and D. Bell, Malaria Rapid Diagnostic Tests in Tropical Climates: The Need for a Cool Chain, Am. J. Trop. Med. Hyg., 2006, 74(5), 750–754 CrossRef PubMed.
- X. Mao, Y. Ma, A. Zhang, L. Zhang, L. Zeng and G. Liu, Disposable Nucleic Acid Biosensors Based on Gold Nanoparticle Probes and Lateral Flow Strip, Anal. Chem., 2009, 81(4), 1660–1668 CrossRef CAS PubMed.
- P. Damborský, K. M. Koczula, A. Gallotta and J. Katrlík, Lectin-Based Lateral Flow Assay: Proof-of-Concept, Analyst, 2016, 141(23), 6444–6448 RSC.
- R. Bertozzi C and L. L. Kiessling, Chemical Glycobiology, Science, 2001, 291(5512), 2357–2364 CrossRef PubMed.
- R. Apweiler, H. Hermjakob and N. Sharon, On the Frequency of Protein Glycosylation, as Deduced from Analysis of the SWISS-PROT Database, Biochim. Biophys. Acta, Gen. Subj., 1999, 1473(1), 4–8 CrossRef CAS.
- R. A. Flynn, K. Pedram, S. A. Malaker, P. J. Batista, B. A. H. Smith, A. G. Johnson, B. M. George, K. Majzoub, P. W. Villalta, J. E. Carette and C. R. Bertozzi, Small RNAs Are Modified with N-Glycans and Displayed on the Surface of Living Cells, Cell, 2021, 184(12), 3109–3124.e22 CrossRef CAS PubMed.
- S. Dedola, M. D. Rugen, R. J. Young and R. A. Field, Revisiting the Language of Glycoscience: Readers, Writers and Erasers in Carbohydrate Biochemistry, ChemBioChem, 2020, 21(3), 423–427 CrossRef CAS PubMed.
- R. S. Haltiwanger, Regulation of Signal Transduction Pathways in Development by Glycosylation, Curr. Opin. Struct. Biol., 2002, 593–598 CrossRef CAS.
- J. J. Bustamante, L. Gonzalez, C. A. Carroll, S. T. Weintraub, R. M. Aguilar, J. Muñoz, A. O. Martinez and L. S. Haro, O-Glycosylated 24 KDa Human Growth Hormone Has a Mucin-like Biantennary Disialylated Tetrasaccharide Attached at Thr-60, Proteomics, 2009, 9(13), 3474–3488 CrossRef CAS PubMed.
-
A. Varki, R. Kannagi and B. P. Toole, Glycosylation Changes in Cancer, Cold Spring Harbor Laboratory Press, 2009 Search PubMed.
- D. Shental-Bechor and Y. Levy, Folding of Glycoproteins: Toward Understanding the Biophysics of the Glycosylation Code, Curr. Opin. Struct. Biol., 2009, 19(5), 524–533 CrossRef CAS PubMed.
- A. Helenius and M. Aebi, Roles of N-Linked Glycans in the Endoplasmic Reticulum, Annu. Rev. Biochem., 2004, 73(1), 1019–1049 CrossRef CAS PubMed.
- J. D. Marth and P. K. Grewal, Mammalian Glycosylation in Immunity, Nat. Rev. Immunol., 2008, 8(11), 874–887 CrossRef CAS PubMed.
- O. Yilmaz, B. Afsar, A. Ortiz and M. Kanbay, The Role of Endothelial Glycocalyx in Health and Disease, Clin. Kidney J., 2019, 12(5), 611–619 CrossRef CAS PubMed.
- Y. Xiong, Y. Chen, L. Ding, X. Liu and H. Ju, Fluorescent Visual Quantitation of Cell-Secreted Sialoglycoconjugates by Chemoselective Recognition and Hybridization Chain Reaction, Analyst, 2019, 144(15), 4545–4551 RSC.
- P. M. Chaudhary, R. V. Murthy, R. Yadav and R. Kikkeri, A Rationally Designed Peptidomimetic Biosensor for Sialic Acid on Cell Surfaces, Chem. Commun., 2015, 51(38), 8112–8115 RSC.
- J. S. Long, B. Mistry, S. M. Haslam and W. S. Barclay, Host and Viral Determinants of Influenza A Virus Species Specificity, Nat. Rev. Microbiol., 2019, 17(2), 67–81 CrossRef CAS PubMed.
- A. Mostafa, E. Abdelwhab, T. Mettenleiter and S. Pleschka, Zoonotic Potential of Influenza A Viruses: A Comprehensive Overview, Viruses, 2018, 10(9), 497 CrossRef PubMed.
- N. H. Tolia, E. J. Enemark, B. K. L. Sim and L. Joshua-Tor, Structural Basis for the EBA-175 Erythrocyte Invasion Pathway of the Malaria Parasite Plasmodium Falciparum, Cell, 2005, 122(2), 183–193 CrossRef CAS PubMed.
-
B. M. van den Berg, M. Nieuwdorp, E. Stroes and H. Vink, Endothelial Luminal Glycocalyx: Protective Barrier between Endothelial Cells and Flowing Blood, in Endothelial Biomedicine, ed. W. C. Aird, Cambridge University Press, Cambridge, 2007, pp. 689–695 Search PubMed.
- J. C. Manning, A. Romero, F. A. Habermann, G. García Caballero, H. Kaltner and H.-J. Gabius, Lectins: A Primer for Histochemists and Cell Biologists, Histochem. Cell Biol., 2017, 147(2), 199–222 CrossRef CAS PubMed.
- H. Ghazarian, B. Idoni and S. B. Oppenheimer, A Glycobiology Review: Carbohydrates, Lectins and Implications in Cancer Therapeutics, Acta Histochem., 2011, 113(3), 236–247 CrossRef CAS PubMed.
- A. A. Lindberg, J. E. Brown, N. Strömberg, M. Westling-Ryd, J. E. Schultz and K. A. Karlsson, Identification of the Carbohydrate Receptor for Shiga Toxin Produced by Shigella Dysenteriae Type 1, J. Biol. Chem., 1987, 262(4), 1779–1785 CrossRef CAS PubMed.
- R. L. Richards, J. Moss, C. R. Alving, P. H. Fishman and R. O. Brady, Choleragen (Cholera Toxin): A Bacterial Lectin, Proc. Natl. Acad. Sci. U. S. A., 1979, 76(4), 1673–1676 CrossRef CAS PubMed.
- S. S. Li, C. H. Wei, J.-Y. Lin and T.-C. Tung, Amino-Terminal Sequences of the Anti-Tumor Lectin Ricin A- and B-Chains, Biochem. Biophys. Res. Commun., 1975, 65(4), 1191–1195 CrossRef CAS PubMed.
- M. A. Sartim and S. V. Sampaio, Snake Venom Galactoside-Binding Lectins: A Structural and Functional Overview, J. Venomous Anim. Toxins Incl. Trop. Dis., 2015, 21, 35 CrossRef PubMed.
- W.-R. Liao, J.-Y. Lin, W.-Y. Shieh, W.-L. Jeng and R. Huang, Antibiotic Activity of Lectins from Marine Algae against Marine Vibrios, J. Ind. Microbiol. Biotechnol., 2003, 30(7), 433–439 CrossRef CAS PubMed.
- R. Jelinek and S. Kolusheva, Carbohydrate Biosensors, Chem. Rev., 2004, 104(12), 5987–6015 CrossRef CAS PubMed.
- S. Cunningham, J. Q. Gerlach, M. Kane and L. Joshi, Glyco-Biosensors: Recent Advances and Applications for the Detection of Free and Bound Carbohydrates, Analyst, 2010, 135(10), 2471–2480 RSC.
- A. Bernardi, J. Jiménez-Barbero, A. Casnati, C. De Castro, T. Darbre, F. Fieschi, J. Finne, H. Funken, K.-E. Jaeger, M. Lahmann, T. K. Lindhorst, M. Marradi, P. Messner, A. Molinaro, P. V. Murphy, C. Nativi, S. Oscarson, S. Penadés, F. Peri, R. J. Pieters, O. Renaudet, J.-L. Reymond, B. Richichi, J. Rojo, F. Sansone, C. Schäffer, W. B. Turnbull, T. Velasco-Torrijos, S. Vidal, S. Vincent, T. Wennekes, H. Zuilhof and A. Imberty, Multivalent Glycoconjugates as Anti-Pathogenic Agents, Chem. Soc. Rev., 2013, 42(11), 4709–4727 RSC.
- N. Sharon, Carbohydrates as Future Anti-Adhesion Drugs for Infectious Diseases, Biochim. Biophys. Acta, Gen. Subj., 2006, 1760(4), 527–537 CrossRef CAS PubMed.
- M. Marradi, F. Chiodo, I. García, S. Penadés, G. M. Whitesides, D. Y. Lee, H. Shin, R. J. Pieters, J. M. Fuente, S.-H. de la; Nishimura, P. Arosio, A. Lascialfari, D. Gatteschi and C. Sangregorio, Glyconanoparticles as Multifunctional and Multimodal Carbohydrate Systems, Chem. Soc. Rev., 2013, 42(11), 4728 RSC.
- N. C. Reichardt, M. Martín-Lomas, S. Penadés, M. Andersson, A. Thran, R. Proksa, Z. A. Fayad, D. P. Cormode, C. Y. Wu, C. H. Wong, M. L. H. Green, K. Kostarelos, B. G. Davis and S. P. Vincent, Glyconanotechnology, Chem. Soc. Rev., 2013, 42(10), 4358–4376 RSC.
- L. T. Lui, X. Xue, C. Sui, A. Brown, D. I. Pritchard, N. Halliday, K. Winzer, S. M. Howdle, F. Fernandez-Trillo, N. Krasnogor and C. Alexander, Bacteria Clustering by Polymers Induces the Expression of Quorum-Sensing-Controlled Phenotypes, Nat. Chem., 2013, 5(12), 1058–1065 CrossRef CAS PubMed.
- D. Chang and J. Zaia, Why Glycosylation Matters in Building a Better Flu Vaccine, Mol. Cell. Proteomics, 2019, 18(12), 2348–2358 CrossRef CAS PubMed.
- T. Tokatlian, B. J. Read, C. A. Jones, D. W. Kulp, S. Menis, J. Y. H. Chang, J. M. Steichen, S. Kumari, J. D. Allen, E. L. Dane, A. Liguori, M. Sangesland, D. Lingwood, M. Crispin, W. R. Schief and D. J. Irvine, Innate Immune Recognition of Glycans Targets HIV Nanoparticle Immunogens to Germinal Centers, Science, 2019, 363(6427), 649–654 CrossRef CAS PubMed.
- G.-J. Boons, Strategies in Oligosaccharide Synthesis, Tetrahedron, 1996, 52(4), 1095–1121 CrossRef CAS.
- B. Lepenies, J. Yin and P. H. Seeberger, Applications of Synthetic Carbohydrates to Chemical Biology, Curr. Opin. Chem. Biol., 2010, 14(3), 404–411 CrossRef CAS PubMed.
- O. J. Plante, E. R. Palmacci and P. H. Seeberger, Automated Solid-Phase Synthesis of Oligosaccharides, Science, 2001, 291(5508), 1523–1527 CrossRef CAS PubMed.
- A. A. Joseph, A. Pardo-Vargas and P. H. Seeberger, Total Synthesis of Polysaccharides by Automated Glycan Assembly, J. Am. Chem. Soc., 2020, 142(19), 8561–8564 CrossRef CAS PubMed.
- M. Guberman and P. H. Seeberger, Automated Glycan Assembly: A Perspective, J. Am. Chem. Soc., 2019, 141(14), 5581–5592 CrossRef CAS PubMed.
- S. Flitsch, Chemical and Enzymatic Synthesis of Glycopolymers, Curr. Opin. Chem. Biol., 2000, 4(6), 619–625 CrossRef CAS PubMed.
- T. Li, L. Liu, N. Wei, J. Y. Yang, D. G. Chapla, K. W. Moremen and G. J. Boons, An Automated Platform for the Enzyme-Mediated Assembly of Complex Oligosaccharides, Nat. Chem., 2019, 11(3), 229–236 CrossRef CAS PubMed.
- S. J. Richards, T. Keenan, J. B. Vendeville, D. E. Wheatley, H. Chidwick, D. Budhadev, C. E. Council, C. S. Webster, H. Ledru, A. N. Baker, M. Walker, M. C. Galan, B. Linclau, M. A. Fascione and M. I. Gibson, Introducing Affinity and Selectivity into Galectin-Targeting Nanoparticles with Fluorinated Glycan Ligands, Chem. Sci., 2021, 12(3), 905–910 RSC.
- J. J. Lundquist and E. J. Toone, The Cluster Glycoside Effect, Chem. Rev., 2002, 102(2), 555–578 CrossRef CAS PubMed.
- Y. C. Lee and R. T. Lee, Carbohydrate-Protein Interactions: Basis of Glycobiology, Acc. Chem. Res., 1995, 28(8), 321–327 CrossRef CAS.
- R. J. Pieters, Maximising Multivalency Effects in Protein–Carbohydrate Interactions, Org. Biomol. Chem., 2009, 7(10), 2013–2025 RSC.
- T. R. Branson, T. E. McAllister, J. Garcia-Hartjes, M. A. Fascione, J. F. Ross, S. L. Warriner, T. Wennekes, H. Zuilhof and W. B. Turnbull, A Protein-Based Pentavalent Inhibitor of the Cholera Toxin B-Subunit, Angew. Chem., Int. Ed., 2014, 53(32), 8323–8327 CrossRef CAS PubMed.
- S.-J. Richards and M. I. Gibson, Toward Glycomaterials with Selectivity as Well as Affinity, JACS Au, 2021, 1(12), 2089–2099 CrossRef CAS PubMed.
- Z. Wang, Z. S. Chinoy, S. G. Ambre, W. Peng, R. McBride, R. P. de Vries, J. Glushka, J. C. Paulson and G.-J. Boons, A General Strategy for the Chemoenzymatic Synthesis of Asymmetrically Branched N-Glycans, Science, 2013, 341(6144), 379–383 CrossRef CAS PubMed.
- S. André, M. Lahmann, H.-J. Gabius and S. Oscarson, Glycocluster Design for Improved Avidity and Selectivity in Blocking Human Lectin/Plant Toxin Binding to Glycoproteins and Cells, Mol. Pharmaceutics, 2010, 7(6), 2270–2279 CrossRef PubMed.
- S. Wang, L. Dupin, M. Noël, C. J. Carroux, L. Renaud, T. Géhin, A. Meyer, E. Souteyrand, J.-J. Vasseur, G. Vergoten, Y. Chevolot, F. Morvan and S. Vidal, Toward the Rational Design of Galactosylated Glycoclusters That Target Pseudomonas Aeruginosa Lectin A (LecA): Influence of Linker Arms That Lead to Low-Nanomolar Multivalent Ligands, Chem. – Eur. J., 2016, 22(33), 11785–11794 CrossRef CAS PubMed.
- T. K. Lindhorst, C. Kieburg and U. Krallmann-Wenzel, Inhibition of the Type 1 Fimbriae-Mediated Adhesion of Escherichia Coli to Erythrocytes by Multiantennary α-Mannosyl Clusters: The Effect of Multivalency, Glycoconjugate J., 1998, 15, 605–613 CrossRef CAS PubMed.
- I. S. MacPherson, J. S. Temme, S. Habeshian, K. Felczak, K. Pankiewicz, L. Hedstrom and I. J. Krauss, Multivalent Glycocluster Design through Directed Evolution, Angew. Chem., Int. Ed., 2011, 50(47), 11238–11242 CrossRef CAS PubMed.
- N. Jayaraman, S. A. Nepogodiev and J. F. Stoddart, Synthetic Carbohydrate-Containing Dendrimers, Chem. – Eur. J., 1997, 3(8), 1193–1199 CrossRef CAS.
- W. B. Turnbull, S. A. Kalovidouris and J. F. Stoddart, Large Oligosaccharide-Based Glycodendrimers Synthetic Carbohydrate Dendrimers, Chem. – Eur. J., 2002, 8(13), 2988–3000 CrossRef CAS PubMed.
- E. K. Woller and M. J. Cloninger, The Lectin-Binding Properties of Six Generations of Mannose-Functionalized Dendrimers, Org. Lett., 2002, 4(1), 7–10 CrossRef CAS PubMed.
- S. Mignani, J. Rodrigues, H. Tomas, R. Roy, X. Shi and J.-P. Majoral, Bench-to-Bedside Translation of Dendrimers: Reality or Utopia? A Concise Analysis, Adv. Drug Delivery Rev., 2018, 136–137, 73–81 CrossRef CAS PubMed.
- S. Zhang, Q. Xiao, S. E. Sherman, A. Muncan, A. D. M. Ramos Vicente, Z. Wang, D. A. Hammer, D. Williams, Y. Chen, D. J. Pochan, S. Vértesy, S. André, M. L. Klein, H. J. Gabius and V. Percec, Glycodendrimersomes from Sequence-Defined Janus Glycodendrimers Reveal High Activity and Sensor Capacity for the Agglutination by Natural Variants of Human Lectins, J. Am. Chem. Soc., 2015, 137(41), 13334–13344 CrossRef CAS PubMed.
- S. G. Spain, M. I. Gibson and N. R. Cameron, Recent Advances in the Synthesis of Well-Defined Glycopolymers, J. Polym. Sci., Part A: Polym. Chem., 2007, 45(11), 2059–2072 CrossRef CAS.
- C. von der Ehe, C. Weber, M. Gottschaldt and U. S. Schubert, Immobilized Glycopolymers: Synthesis, Methods and Applications, Prog. Polym. Sci., 2016, 57, 64–102 CrossRef CAS.
- V. Vázquez-Dorbatt and H. D. Maynard, Biotinylated Glycopolymers Synthesized by Atom Transfer Radical Polymerization, Biomacromolecules, 2006, 7(8), 2297–2302 CrossRef PubMed.
- A. B. Lowe, B. S. Sumerlin and C. L. McCormick, The Direct Polymerization of 2-Methacryloxyethyl Glucoside via Aqueous Reversible Addition-Fragmentation Chain Transfer (RAFT) Polymerization, Polymer, 2003, 44(22), 6761–6765 CrossRef CAS.
- L. E. Strong and L. L. Kiessling, A General Synthetic Route to Defined, Biologically Active Multivalent Arrays, J. Am. Chem. Soc., 1999, 121(26), 6193–6196 CrossRef CAS.
- K. Ohno, Y. Tsujii, T. Miyamoto, T. Fukuda, M. Goto, K. Kobayashi and T. Akaike, Synthesis of a Well-Defined Glycopolymer by Nitroxide-Controlled Free Radical Polymerization, Macromolecules, 1998, 31(4), 1064–1069 CrossRef CAS.
- M. Mammen, G. Dahmann and G. M. Whitesides, Effective Inhibitors of Hemagglutination by Influenza Virus Synthesized from Polymers Having Active Ester Groups. Insight into Mechanism of Inhibition, J. Med. Chem., 1995, 38(21), 4179–4190 CrossRef CAS PubMed.
- G. B. Sigal, M. Mammen, G. Dahmann and G. M. Whitesides, Polyacrylamides Bearing Pendant α-Sialoside Groups Strongly Inhibit Agglutination of Erythrocytes by Influenza Virus: The Strong Inhibition Reflects Enhanced Binding through Cooperative Polyvalent Interactions, J. Am. Chem. Soc., 1996, 118(16), 3789–3800 CrossRef CAS.
- X. Xue, G. Pasparakis, N. Halliday, K. Winzer, S. M. Howdle, C. J. Cramphorn, N. R. Cameron, P. M. Gardner, B. G. Davis, F. Fernμndez-Trillo, C. Alexander, F. Fernández-Trillo and C. Alexander, Synthetic Polymers for Simultaneous Bacterial Sequestration and Quorum Sense Interference, Angew. Chem., Int. Ed., 2011, 50(42), 9852–9856 CrossRef CAS PubMed.
- R. J. Pieters, Intervention with Bacterial Adhesion by Multivalent Carbohydrates, Med. Res. Rev., 2007, 27(6), 796–816 CrossRef CAS PubMed.
- A. G. Kruger, S. D. Brucks, T. Yan, G. Cárcarmo-Oyarce, Y. Wei, D. H. Wen, D. R. Carvalho, M. J. A. Hore, K. Ribbeck, R. R. Schrock and L. L. Kiessling, Stereochemical Control Yields Mucin Mimetic Polymers, ACS Cent. Sci., 2021, 7(4), 624–630 CrossRef CAS PubMed.
- M. Nagao, M. Kichize, Y. Hoshino and Y. Miura, Influence of Monomer Structures for Polymeric Multivalent Ligands: Consideration of the Molecular Mobility of Glycopolymers, Biomacromolecules, 2021, 22(7), 3119–3127 CrossRef CAS PubMed.
- L. E. Wilkins, N. Badi, F. Du Prez and M. I. Gibson, Double-Modified Glycopolymers from Thiolactones to Modulate Lectin Selectivity and Affinity, ACS Macro Lett., 2018, 7(12), 1498–1502 CrossRef CAS PubMed.
- M. W. Jones, L. Otten, S.-J. Richards, R. Lowery, D. J. Phillips, D. M. Haddleton and M. I. Gibson, Glycopolymers with Secondary Binding Motifs Mimic Glycan Branching and Display Bacterial Lectin Selectivity in Addition to Affinity, Chem. Sci., 2014, 5(4), 1611–1616 RSC.
- S.-J. Richards, M. W. Jones, M. Hunaban, D. M. Haddleton and M. I. Gibson, Probing Bacterial-Toxin Inhibition with Synthetic Glycopolymers Prepared by Tandem Post-Polymerization Modification: Role of Linker Length and Carbohydrate Density, Angew. Chem., Int. Ed., 2012, 51(31), 7812–7816 CrossRef CAS PubMed.
- B. D. Polizzotti and K. L. Kiick, Effects of Polymer Structure on the Inhibition of Cholera Toxin by Linear Polypeptide-Based Glycopolymers, Biomacromolecules, 2006, 7(2), 483–490 CrossRef CAS PubMed.
- H.-A. Tran, P. I. Kitov, E. Paszkiewicz, J. M. Sadowska and D. R. Bundle, Multifunctional Multivalency: A Focused Library of Polymeric Cholera Toxin Antagonists, Org. Biomol. Chem., 2011, 9(10), 3658–3671 RSC.
- B. Martyn, C. I. Biggs and M. I. Gibson, Comparison of Systematically Functionalized Heterogeneous and Homogenous Glycopolymers as Toxin Inhibitors, J. Polym. Sci., Part A: Polym. Chem., 2019, 57(1), 40–47 CrossRef CAS.
- D. Ponader, P. Maffre, J. Aretz, D. Pussak, N. M. Ninnemann, S. Schmidt, P. H. Seeberger, C. Rademacher, G. U. Nienhaus and L. Hartmann, Carbohydrate-Lectin Recognition of Sequence-Defined Heteromultivalent Glycooligomers, J. Am. Chem. Soc., 2014, 136(5), 2008–2016 CrossRef CAS PubMed.
- C. S. Mahon, M. A. Fascione, C. Sakonsinsiri, T. E. McAllister, W. B. Turnbull and D. A. Fulton, Templating Carbohydrate-Functionalised Polymer-Scaffolded Dynamic Combinatorial Libraries with Lectins, Org. Biomol. Chem., 2015, 13(9), 2756–2761 RSC.
- A. L. Parry, N. A. Clemson, J. Ellis, S. S. R. Bernhard, B. G. Davis and N. R. Cameron, “Multicopy Multivalent” Glycopolymer-Stabilized Gold Nanoparticles as Potential Synthetic Cancer Vaccines, J. Am. Chem. Soc., 2013, 135(25), 9362–9365 CrossRef CAS PubMed.
- Y. Qu, Y. Li, S. Liao, J. Sun, M. Li, D. Wang, C. Xia, Q. Luo, J. Hu, K. Luo, Q. Gong and B. Song, Linear and Core-Crosslinked Glycopolymer-Gadolinium Conjugates: Preparation and Their Behaviors as Nanoscale Magnetic Resonance Imaging Contrast Agents, J. Biomed. Nanotechnol., 2019, 15(8), 1637–1653 CrossRef CAS PubMed.
- Y. Wang, Y. Dai, Q. Luo, X. Wei, X. Xiao, H. Li, J. Hu, Q. Gong, J. Wu and K. Luo, Tumor Environment-Responsive Degradable Branched Glycopolymer Magnetic Resonance Imaging Contrast Agent and Its Tumor-Targeted Imaging, J. Biomed. Nanotechnol., 2019, 15(7), 1384–1400 CrossRef CAS PubMed.
- C. Cao, J. Zhao, M. Lu, C. J. Garvey and M. H. Stenzel, Correlation between Drug Loading Content and Biological Activity: The Complexity Demonstrated in Paclitaxel-Loaded Glycopolymer Micelle System, Biomacromolecules, 2019, 20(4), 1545–1554 CrossRef CAS PubMed.
- J. Wu, J. Yuan, B. Ye, Y. Wu, Z. Xu, J. Chen and J. Chen, Dual-Responsive Core Crosslinking Glycopolymer-Drug Conjugates Nanoparticles for Precise Hepatocarcinoma Therapy, Front. Pharmacol., 2018, 9, 663 CrossRef PubMed.
- R. S. Loka, E. T. Sletten, U. Barash, I. Vlodavsky and H. M. Nguyen, Specific Inhibition of Heparanase by a Glycopolymer with Well-Defined Sulfation Pattern Prevents Breast Cancer Metastasis in Mice, ACS Appl. Mater. Interfaces, 2019, 11(1), 244–254 CrossRef CAS PubMed.
- M. L. Huang, R. A. A. Smith, G. W. Trieger and K. Godula, Glycocalyx Remodeling with Proteoglycan Mimetics Promotes Neural Specification in Embryonic Stem Cells, J. Am. Chem. Soc., 2014, 136(30), 10565–10568 CrossRef CAS PubMed.
- M. Chikae, T. Fukuda, K. Kerman, K. Idegami, Y. Miura and E. Tamiya, Amyloid-β Detection with Saccharide Immobilized Gold Nanoparticle on Carbon Electrode, Bioelectrochemistry, 2008, 74(1), 118–123 CrossRef CAS PubMed.
- C. K. Adokoh, S. Quan, M. Hitt, J. Darkwa, P. Kumar and R. Narain, Synthesis and Evaluation of Glycopolymeric Decorated Gold Nanoparticles Functionalized with Gold-Triphenyl Phosphine as Anti-Cancer Agents, Biomacromolecules, 2014, 15(10), 3802–3810 CrossRef CAS PubMed.
- M. Ahmed, Z. Deng and R. Narain, Study of Transfection Efficiencies of Cationic Glyconanoparticles of Different Sizes in Human Cell Line, ACS Appl. Mater. Interfaces, 2009, 1(9), 1980–1987 CrossRef CAS PubMed.
- Z. Wang, Z. S. Chinoy, S. G. Ambre, W. Peng, R. McBride, R. P. de Vries, J. Glushka, J. C. Paulson and G.-J. Boons, A General Strategy for the Chemoenzymatic Synthesis of Asymmetrically Branched N-Glycans, Science, 2013, 341(6144), 379–383 CrossRef CAS PubMed.
- P. J. Hernando, S. Dedola, M. J. Marín and R. A. Field, Recent Developments in the Use of Glyconanoparticles and Related Quantum Dots for the Detection of Lectins, Viruses, Bacteria and Cancer Cells, Front. Chem., 2021, 9, 560 Search PubMed.
- A. Hucknall, S. Rangarajan and A. Chilkoti, In Pursuit of Zero: Polymer Brushes That Resist the Adsorption of Proteins, Adv. Mater., 2009, 21(23), 2441–2446 CrossRef CAS.
- J. O. Zoppe, N. C. Ataman, P. Mocny, J. Wang, J. Moraes and H. A. Klok, Surface-Initiated Controlled Radical Polymerization: State-of-the-Art, Opportunities, and Challenges in Surface and Interface Engineering with Polymer Brushes, Chem. Rev., 2017, 117(3), 1105–1318 CrossRef CAS PubMed.
- M. J. Marín, A. Rashid, M. Rejzek, S. A. Fairhurst, S. A. Wharton, S. R. Martin, J. W. McCauley, T. Wileman, R. A. Field and D. A. Russell, Glyconanoparticles for the Plasmonic Detection and Discrimination between Human and Avian Influenza Virus, Org. Biomol. Chem., 2013, 11(41), 7101 RSC.
- Á. G. Barrientos, J. M. De la Fuente, T. C. Rojas, A. Fernández and S. Penadés, Gold Glyconanoparticles: Synthetic Polyvalent Ligands Mimicking Glycocalyx-like Surfaces as Tools for Glycobiological Studies, Chem. – Eur. J., 2003, 9(9), 1909–1921 CrossRef PubMed.
- M. J. Hernáiz, J. M. De La Fuente, Á. G. Barrientos and S. Penadés, A Model System Mimicking Glycosphingolipid Clusters to Quantify Carbohydrate Self-Interactions by Surface Plasmon Resonance, Angew. Chem., Int. Ed., 2002, 41(9), 1554–1557 CrossRef.
- A. B. Lowe, B. S. Sumerlin, M. S. Donovan and C. L. McCormick, Facile Preparation of Transition Metal Nanoparticles Stabilized by Well-Defined (Co)Polymers Synthesized via Aqueous Reversible Addition-Fragmentation Chain Transfer Polymerization, J. Am. Chem. Soc., 2002, 124(39), 11562–11563 CrossRef CAS PubMed.
- S. G. Spain, L. Albertin and N. R. Cameron, Facile in Situ Preparation of Biologically Active Multivalent Glyconanoparticles, Chem. Commun., 2006, 4198–4200 RSC.
- A. L. Parry, N. A. Clemson, J. Ellis, S. S. R. Bernhard, B. G. Davis and N. R. Cameron, “Multicopy Multivalent” Glycopolymer-Stabilized Gold Nanoparticles as Potential Synthetic Cancer Vaccines, J. Am. Chem. Soc., 2013, 135(25), 9362–9365 CrossRef CAS PubMed.
- G. Yilmaz, E. Guler, C. Geyik, B. Demir, M. Ozkan, D. Odaci Demirkol, S. Ozcelik, S. Timur and C. R. Becer, PH Responsive Glycopolymer Nanoparticles for Targeted Delivery of Anti-Cancer Drugs, Mol. Syst. Des. Eng., 2018, 3(1), 150–158 RSC.
- F.-W. Shen, K.-C. Zhou, H. Cai, Y.-N. Zhang, Y.-L. Zheng and J. Quan, One-Pot Synthesis of Thermosensitive Glycopolymers Grafted Gold Nanoparticles and Their Lectin Recognition, Colloids Surf., B, 2019, 173, 504–511 CrossRef CAS PubMed.
- K. Mizukami, H. Takakura, T. Matsunaga and H. Kitano, Binding of Ricinus Communis Agglutinin to a Galactose-Carrying Polymer Brush on a Colloidal Gold Monolayer, Colloids Surf., B, 2008, 66(1), 110–118 CrossRef CAS PubMed.
- S. Nu, H. Bttcher, H. Wurm and M. L. Hallensleben, Gold Nanoparticles with Covalently Attached Polymer Chains, Angew. Chem., Int. Ed., 2001, 40(21), 4016–4018 CrossRef.
- L. Wu, U. Glebe and A. Böker, Surface-Initiated Controlled Radical Polymerizations from Silica Nanoparticles, Gold Nanocrystals, and Bionanoparticles, Polym. Chem., 2015, 6(29), 5143–5184 RSC.
- H. M. Berman, J. Westbrook, Z. Feng, G. Gilliland, T. N. Bhat, H. Weissig, I. N. Shindyalov and P. E. Bourne, The Protein Data Bank, Nucleic Acids Res., 2000, 28(1), 235–242 CrossRef CAS PubMed.
- L. R. Olsen, A. Dessen, D. Gupta, S. Sabesan, J. C. Sacchettini and C. F. Brewer, X-Ray Crystallographic Studies of Unique Cross-Linked Lattices between Four Isomeric Biantennary Oligosaccharides and Soybean Agglutinin, Biochemistry, 1997, 36(49), 15073–15080 CrossRef CAS PubMed.
- K. D. Hardman and C. F. Ainsworth, Structure of Concanavalin A at 2.4-Ang Resolution, Biochemistry, 1972, 11(26), 4910–4919 CrossRef CAS PubMed.
- R. G. Zhang, D. L. Scott, M. L. Westbrook, S. Nance, B. D. Spangler, G. G. Shipley and E. M. Westbrook, The Three-Dimensional Crystal Structure of Cholera Toxin, J. Mol. Biol., 1995, 251, 563–573 CrossRef CAS PubMed.
- D. Schwefel, C. Maierhofer, J. G. Beck, S. Seeberger, K. Diederichs, H. M. Moller, W. Welte and V. Wittmann, Structural Basis of Multivalent Binding to Wheat Germ Agglutinin, J. Am. Chem. Soc., 2010, 132, 8704–8719 CrossRef CAS PubMed.
- S. K. Natchiar, O. Srinivas, N. Mitra, A. Surolia, N. Jayaraman and M. Vijayan, Structural Studies on Peanut Lectin Complexed with Disaccharides Involving Different Linkages: Further Insights into the Structure and Interactions of the Lectin, Acta Crystallogr., Sect. D: Biol. Crystallogr., 2006, 62, 1413–1421 CrossRef PubMed.
- M. J. Marín, C. L. Schofield, R. A. Field and D. A. Russell, Glyconanoparticles for Colorimetric Bioassays, Analyst, 2015, 140(1), 59–70 RSC.
- C. A. Mirkin, R. L. Letsinger, R. C. Mucic and J. J. Storhoff, A DNA-Based Method for Rationally Assembling Nanoparticles into Macroscopic Materials, Nature, 1996, 382(6592), 607–609 CrossRef CAS PubMed.
- J. L. Wang and G. M. Edelman, Binding and Functional Properties of Concanavalin A and Its Derivatives. II. A Proteolytic Product with Saccharide Binding Activity, J. Biol. Chem., 1978, 253(9), 3008–3015 CrossRef CAS PubMed.
- H. de Boeck, H. Lis, H. van Tilbeurgh, N. Sharon and F. G. Loontiens, Binding of Simple Carbohydrates and Some of Their Chromophoric Derivatives to Soybean Agglutinin as Followed by Titrimetric Procedures and Stopped Flow Kinetics, J. Biol. Chem., 1984, 259(11), 7067–7074 CrossRef CAS PubMed.
- M. Toyoshima, K. Ooya, Y. Miura and K. Kobayashi, Preparation and Biological Properties of Saccharide-Modified Gold Nanoparticles, J. Jpn. Soc. Powder Powder Metall., 2007, 54(12), 843–848 CrossRef CAS.
- C. L. Schofield, B. Mukhopadhyay, S. M. Hardy, M. B. McDonnell, R. A. Field and D. A. Russell, Colorimetric Detection of Ricinus Communis Agglutinin 120 Using Optimally Presented Carbohydrate-Stabilised Gold Nanoparticles, Analyst, 2008, 133(5), 626–634 RSC.
- X.-L. Hu, H.-Y. Jin, X.-P. He, T. D. James, G.-R. Chen and Y.-T. Long, Colorimetric and Plasmonic Detection of Lectins Using Core–Shell Gold Glyconanoparticles Prepared by Copper-Free Click Chemistry, ACS Appl. Mater. Interfaces, 2015, 7(3), 1874–1878 CrossRef CAS PubMed.
- X.-P. He, Y.-L. Zeng, Y. Zang, J. Li, R. A. Field and G.-R. Chen, Carbohydrate CuAAC Click
Chemistry for Therapy and Diagnosis, Carbohydr. Res., 2016, 429, 1–22 CrossRef CAS PubMed.
- C. L. Schofield, R. A. Field and D. A. Russell, Glyconanoparticles for the Colorimetric Detection of Cholera Toxin, Anal. Chem., 2007, 79(4), 1356–1361 CrossRef CAS PubMed.
- S.-J. Richards and M. I. Gibson, Optimization of the Polymer Coating for Glycosylated Gold Nanoparticle Biosensors to Ensure Stability and Rapid Optical Readouts, ACS Macro Lett., 2014, 3(10), 1004–1008 CrossRef CAS PubMed.
- S. Won, S.-J. Richards, M. Walker and M. I. Gibson, Externally Controllable Glycan Presentation on Nanoparticle Surfaces to Modulate Lectin Recognition, Nanoscale Horiz., 2017, 3(2), 1593–1608 Search PubMed.
- M. Toyoshima and Y. Miura, Preparation of Glycopolymer-Substituted Gold Nanoparticles and Their Molecular Recognition, J. Polym. Sci., Part A: Polym. Chem., 2009, 47(5), 1412–1421 CrossRef CAS.
- S.-J. Richards, E. C. Fullam, G. S. Besra and M. I. Gibson, Discrimination between Bacterial Phenotypes Using Glyco-Nanoparticles and the Impact of Polymer Coating on Detection Readouts, J. Mater. Chem. B, 2014, 2(11), 1490–1498 RSC.
- I. García, J. Mosquera, J. Plou and L. M. Liz-Marzán, Plasmonic Detection of Carbohydrate-Mediated Biological Events, Adv. Opt. Mater., 2018, 6(23), 1800680 CrossRef.
- D. Craig, J. Simpson, K. Faulds and D. Graham, Formation of SERS Active Nanoparticle Assemblies via Specific Carbohydrate–Protein Interactions, Chem. Commun., 2013, 49(1), 30–32 RSC.
- J. Langer, I. García and L. M. Liz-Marzán, Real-Time Dynamic SERS Detection of Galectin Using Glycan-Decorated Gold Nanoparticles, Faraday Discuss., 2017, 205(0), 363–375 RSC.
- M. Toyoshima, T. Oura, T. Fukuda, E. Matsumoto and Y. Miura, Biological Specific Recognition of Glycopolymer- Modified Interfaces by RAFT Living Radical Polymerization, Polym. J., 2010, 42(2), 172–178 CrossRef CAS.
- J. Ishii, M. Toyoshima, M. Chikae, Y. Takamura and Y. Miura, Preparation of Glycopolymer-Modified Gold Nanoparticles and a New Approach for a Lateral Flow Assay, Bull. Chem. Soc. Jpn., 2011, 84(5), 466–470 CrossRef CAS.
- M. Takara, M. Toyoshima, H. Seto, Y. Hoshino and Y. Miura, Polymer-Modified Gold Nanoparticles via RAFT Polymerization: A Detailed Study for a Biosensing Application, Polym. Chem., 2014, 5(3), 931–939 RSC.
- N. Sze Ieong, C. I. Biggs, M. Walker and M. I. Gibson, Comparison of RAFT-Derived Poly(Vinylpyrrolidone) Verses Poly(Oligoethyleneglycol Methacrylate) for the Stabilization of Glycosylated Gold Nanoparticles, J. Polym. Sci., Part A: Polym. Chem., 2017, 55(7), 1200–1208 CrossRef CAS PubMed.
- P. G. Georgiou, A. N. Baker, S.-J. J. Richards, A. Laezza, M. Walker and M. I. Gibson, Tuning Aggregative versus Non-Aggregative Lectin Binding with Glycosylated Nanoparticles by the Nature of the Polymer Ligand, J. Mater. Chem. B, 2020, 8(1), 136–145 RSC.
- A. N. Baker, S. J. Richards, C. S. Guy, T. R. Congdon, M. Hasan, A. J. Zwetsloot, A. Gallo, J. R. Lewandowski, P. J. Stansfeld, A. Straube, M. Walker, S. Chessa, G. Pergolizzi, S. Dedola, R. A. Field and M. I. Gibson, The SARS-COV-2 Spike Protein Binds Sialic Acids and Enables Rapid Detection in a Lateral Flow Point of Care Diagnostic Device. ACS Central, Science, 2020, 6(11), 2046–2052 CAS.
- L. Nguyen, K. A. McCord, D. T. Bui, K. A. Bouwman, E. N. Kitova, D. Kumawat, G. C. Daskan, I. Tomris, L. Han, P. Chopra, T.-J. Yang, S. D. Williows, T. L. Lowary, L. J. West, S.-T. D. Hsu, S. M. Tompkins, G.-J. Boons, A. L. Mason, R. P. de Vries, M. S. Macauley and J. S. Klassen, Sialic Acid-Containing Glycolipids Mediate Binding and Viral Entry of SARS-CoV-2, Nat. Chem. Biol., 2022,(18), 81–90 CrossRef CAS PubMed.
- C. J. Buchanan, B. Gaunt, P. J. Harrison, Y. Yang, J. Liu, A. Khan, A. M. Giltrap, A. Le Bas, P. N. Ward, K. Gupta, M. Dumoux, T. K. Tan, L. Schimaski, S. Daga, N. Picchiotti, M. Baldassarri, E. Benetti, C. Fallerini, F. Fava, A. Giliberti, P. I. Koukos, M. J. Davy, A. Lakshminarayanan, X. Xue, G. Papadakis, L. P. Deimel, V. Casablancas-Antràs, T. D. W. Claridge, A. M. J. J. Bonvin, Q. J. Sattentau, S. Furini, M. Gori, J. Huo, R. J. Owens, C. Schaffitzel, I. Berger, A. Renieri, J. H. Naismith, A. J. Baldwin and B. G. Davis, Pathogen-Sugar Interactions Revealed by Universal Saturation Transfer Analysis, Science, 2022 DOI:10.1126/science.abm3125.
- A. N. Baker, S.-J. Richards, S. Pandey, C. S. Guy, A. Ahmad, M. Hasan, C. I. Biggs, P. G. Georgiou, A. J. Zwetsloot, A. Straube, S. Dedola, R. A. Field, N. R. Anderson, M. Walker, D. Grammatopoulos and M. I. Gibson, Glycan-Based Flow-Through Device for the Detection of SARS-COV-2, ACS Sens., 2021, 6(10), 3696–3705 CrossRef CAS PubMed.
- A. N. Baker, A. R. Muguruza, S. Richards, P. G. Georgiou, S. Goetz, M. Walker, S. Dedola, R. A. Field and M. I. Gibson, Lateral Flow Glyco-Assays for the Rapid and Low-Cost Detection of Lectins – Polymeric Linkers and Particle Engineering Are Essential for Selectivity and Performance, Adv. Healthcare Mater., 2022, 11, 2101784 CrossRef CAS PubMed.
- A. N. Baker, T. R. Congdon, S.-J. Richards, P. G. Georgiou, M. Walker, S. Dedola, R. A. Field and M. I. Gibson, End-Functionalized Poly(Vinylpyrrolidone) for Ligand Display in Lateral Flow Device Test Lines, ACS Polym. Au, 2022, 2(2), 69–79 CrossRef CAS PubMed.
- S. H. Kim, F. L. Kearns, M. A. Rosenfeld, L. Casalino, M. J. Papanikolas, C. Simmerling, R. E. Amaro and R. Freeman, GlycoGrip: Cell Surface-Inspired Universal Sensor for Betacoronaviruses, ACS Cent. Sci., 2022, 8(1), 22–42 CrossRef CAS PubMed.
|
This journal is © The Royal Society of Chemistry 2022 |