DOI:
10.1039/D2CC02431D
(Feature Article)
Chem. Commun., 2022,
58, 9991-10003
The advent of electrophilic hydroxylamine-derived reagents for the direct preparation of unprotected amines
Received
29th April 2022
, Accepted 20th July 2022
First published on 22nd August 2022
Abstract
Electrophilic aminating reagents have seen a renaissance in recent years as effective nitrogen sources for the synthesis of unprotected amino functionalities. Based on their reactivity, several noble and non-noble transition metal catalysed amination reactions have been developed. These include the aziridination and difunctionalisation of alkenes, the amination of arenes as well as the synthesis of aminated sulfur compounds. In particular, the use of hydroxylamine-derived (N–O) reagents, such as PONT (PivONH3OTf), has enabled the introduction of unprotected amino groups on various different feedstock compounds, such as alkenes, arenes and thiols. This strategy obviates undesired protecting-group manipulations and thus improves step efficiency and atom economy. Overall, this feature article gives a recent update on several reactions that have been unlocked by employing versatile hydroxylamine-derived aminating reagents, which facilitate the generation of unprotected primary, secondary and tertiary amino groups.
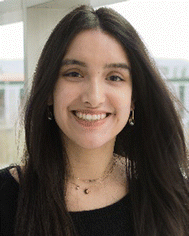
Valentina C. M. Gasser
| Valentina Gasser studied at the ETH Zürich (2011–2018), where she obtained her MSc degree in Chemistry and carried out research in the groups of Prof. P. Chen and Prof. E. M. Carreira. She joined the group of Prof. R. Sarpong (UC Berkeley) for her Master's thesis in the United States. After an industrial placement at Givaudan in Process Research in Zürich, CH, she joined the Morandi group as a doctoral candidate at ETH Zürich. Her research focus lies in iron-catalysed amination reactions. She is active in projects that strive to achieve more diversity, equity and inclusion in academia. |
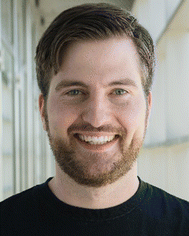
Szabolcs Makai
| Szabolcs Makai studied at the Ludwig-Maximilians University in Munich, Germany (2012–2018), where he obtained his MSc degree in Chemistry. After research stays in the groups of Prof. D. Trauner (LMU Munich), Prof. S. V. Ley (University of Cambridge) and an industrial placement at Novartis in Basel, CH, he joined the Morandi group for his Master's thesis. He continued as doctoral candidate within the same group at ETH Zurich where he is focusing on iron-catalysed amination reactions. During his PhD studies, he conducted an industrial placement at Syngenta Crop Protection in Stein, CH. |
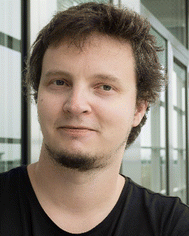
Bill Morandi
| Bill Morandi studied at the ETH Zurich (2003–2008), earning a BSc in Biology and an MSc in Chemical Biology. After a PhD with Prof. Erick M. Carreira, he moved in 2012 to CalTech to work with Prof. Robert H. Grubbs as a postdoctoral fellow. From 2014 to 2018, he was an independent Max Planck Research Group Leader at the Max-Planck-Institut für Kohlenforschung (Mülheim, Germany), before subsequently returning to ETH Zürich as a Professor in 2018. He is currently Full Professor of Synthetic Organic Chemistry and heads the Institute of Organic Chemistry (Laboratorium für Organische Chemie) at ETH Zürich. |
1 Introduction
The introduction of amino groups is one of the most important tasks in organic synthesis, as numerous bioactive compounds contain nitrogen atoms. More than 90% of the top 100 small molecule therapeutics sold in 2020 possess at least one C–N bond.1 Catalytic amination reactions represent a powerful toolbox to forge these bonds and create valuable building blocks from easily accessible hydrocarbon feedstocks. An emerging approach in this research area is the use of electrophilic nitrogen reagents to generate useful aminated products. However, modulating groups, such as electron-withdrawing moieties, are often critical to controlling the reactivity of the precursor and the active aminating species. The common issue among these methods is that the installed amino functionality bears hard to cleave protecting groups, such as sulfonamides. Their removal requires an additional step and operates usually under harsh reaction conditions, creating challenges in downstream functionalisation, thus limiting the step- and atom economy of the overall amination reaction. Obviating the installation of this protecting group would greatly streamline the way an amine is introduced in a synthetic sequence.
Recently, our group, among others, has been involved in the development of Fe catalysed amination reactions that allow the direct installation of unprotected amines into abundant feedstock compounds, such as alkenes, arenes and thiols. A key advance in establishing these reactions was the development and use of bench-stable, protonated hydroxylamine derivatives which exhibit sufficient reactivity to access the desired product in an unprotected form.2 A key reagent, O-pivaloylated hydroxylammonium reagent R7 (PivONH3OTf), has played a particularly enabling role in our research group.3 This reagent was initially reported by Guimond and Fagnou as a convenient building block to introduce an O-acyl hydroxamic acid directing group.4 Its protonated form prevents a facile rearrangement to the more stable N-hydroxy amide and thus makes the reagent an ideal NH2+ surrogate.5 We recently disclosed a reliable scale-up synthesis of this reagent on a decagram scale.6 This reagent has also become commercially available, further facilitating its use in preparative reactions. In this review, the acronym PONT (
iv![[O with combining low line]](https://www.rsc.org/images/entities/char_004f_0332.gif)
H3O
f, O-pivaloyl hydroxylammonium triflate) is used to describe this reagent. Besides PONT, other important hydroxylamine-derived (N–O) reagents as well as related N–X reagents, are presented within this review (Fig. 1).7–10
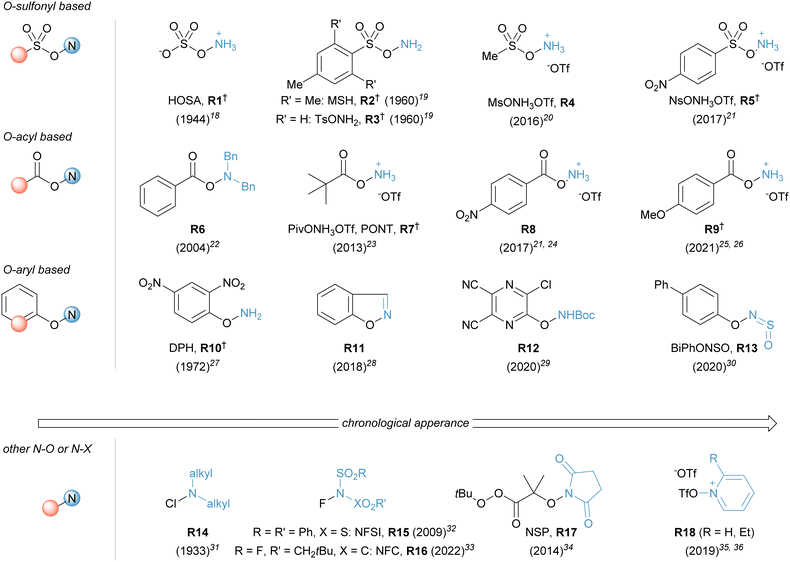 |
| Fig. 1 Selected N–X based reagents in electrophilic amination reactions ordered according to their core structure. Year of their first reported application in brackets.18–36 Reagents with known N-substituted congeners are marked with a dagger. | |
The aim of this feature article is to present the evolution of the field of unprotected amine preparation through the use of electrophilic nitrogen reagents. The focus lies in the specific areas of interest of our research group along with important literature precedent as well as contemporary methods. We will begin with recent advances in the field of alkene amination (Section 2.1), continue with methods for the amination of aromatic C–H bonds (Section 2.2) and conclude with a section on the amination of sulfur-containing moieties (Section 2.3). To complement our non-comprehensive article, the readers are referred to excellent reviews on catalytic aminations for extended reading.11–17
2 Introduction of nitrogen through electrophilic reagents
2.1 Amination of alkenes
Alkenes represent an important class of feedstock hydrocarbons suitable to react with hydroxylamine-derived aminating reagents. Practical ways to combine alkenes with electrophilic aminating reagents proceed through aziridination37 or difunctionalisation strategies.38 By applying the latter, an additional moiety is directly installed at the vicinal position relative to the amino group, thereby enabling the construction of densely functionalised target molecules. This distinguishes difunctionalisations from hydroamination reactions, which are not covered in this article.28,39–42
2.1.1 Aziridination of alkenes.
Two seminal reports from Hamelin43 and Bottaro44 independently showed the inherent reactivity of N-unprotected, O-arylsulfonyl reagents R2 (O-(mesitylsulfonyl)hydroxylamine, MSH) and R3 (O-(tosyl)hydroxylamine, TsONH2) to afford unprotected aziridines. Three decades later, a collaborative work between the groups of Ess, Kürti and Falck disclosed an improved protocol which uses safer45O-aryl reagent R10 (O-(2,4-dinitrophenyl)hydroxylamine, DPH) (Scheme 1A).46 The Rh catalysed reaction proceeds mildly with broad functional group tolerance on both activated and unactivated alkenes. Using a methylated DPH reagent allowed the installation of N-methyl aziridines.
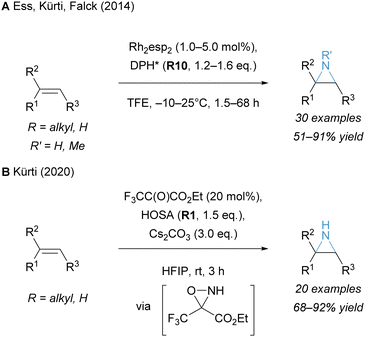 |
| Scheme 1 Unprotected, direct aziridination of alkenes. esp: α,α,α′,α′-tetramethyl-1,3-benzenedipropionic acid. | |
This transformation was further improved and resulted in variations where more inexpensive aminating reagents47,48 and non-noble metal catalysts, such as copper and iron,49,50 could be used instead.
Recently, an elegant organocatalytic version of this transformation was presented by the Kürti group (Scheme 1B).51 The key intermediate proved to be the corresponding oxaziridine, which was generated in situ from R1 (hydroxylamine-O-sulfonic acid, HOSA) and a catalytic amount of an electron-deficient ketone. This unusual N–O reagent transferred an NH equivalent to an alkene in a concerted fashion, mechanistically reminiscent of an epoxidation with dioxiranes. This similarity was further exploited in the induction of enantioselectivity when using a chiral ketone.52
Another notable example of transition-metal free N-methylaziridination was developed by Bower and co-workers using an in situ Boc-deprotected O-sulfonyl hydroxylamine reagent.53
Further references for exemplary intramolecular54–58 transformations and those that afford the nitrogen in a protected59–63 form or from enones64 are given, albeit not discussed in greater detail in this article.
2.1.2 Aminohalogenation of alkenes.
Despite very early initial reports65–67 about 2-haloamines and their synthesis from alkenes, it was only a decade ago that aminohalogenation reactions experienced a renaissance seen with the development of a series of catalytic reactions.68–70 Several of them were based on using N-sulfonamide reagents and consequently led to the installation of N-sulfonyl protected amino motifs. An additional deprotection step was required to liberate the free amine, often employing harsh conditions.71–73 In contrast, extensive work by Xu and co-workers showed that O-benzyl hydroxylamine-derived reagents were suitable for the introduction of N-carbamate protected β-haloamines under Fe catalysis.74,75
An Fe catalyst was used by our group for developing a mild aminochlorination reaction (Scheme 2A).76 Benign sodium chloride served as the halogen source, whereas the unprotected amino group was introduced by the use of O-pivaloylated hydroxylammonium reagent PONT (R7). The aminochlorination reaction proceeded under ambient conditions and transformed a wide range of vinyl arenes and unactivated, aliphatic alkenes with excellent Markovnikov selectivity to the desired 2-chloroamines. Several functional groups were tolerated (e.g. free alcohols, alkynes and N-heterocycles). Downstream derivatisations showed facile transformation to a broad range of products like aziridines and divergent reduction of the 2-chloroamine to either linear or branched primary amines.
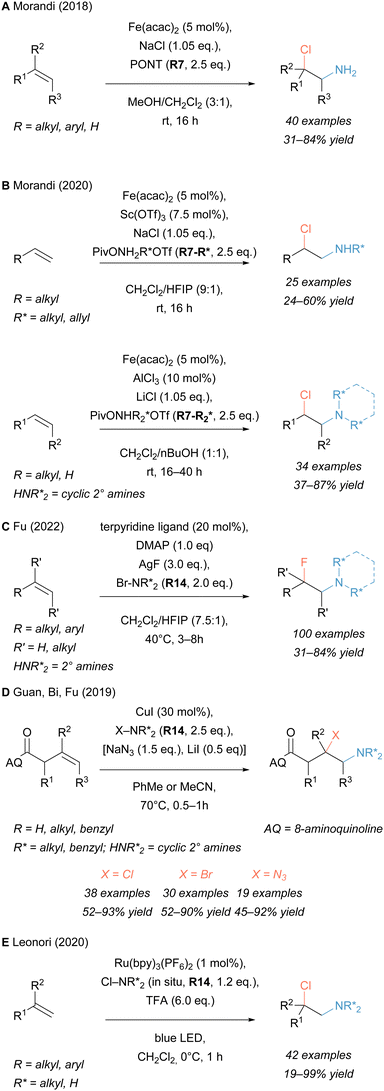 |
| Scheme 2 Unprotected aminohalogenation of alkenes. Acac: acetylacetonate. | |
Recent work by our group expanded the scope of this reaction to unprotected secondary and tertiary amino groups by developing new, structurally related, N-alkylated hydroxylammonium reagents R7-R* and R7-R2* to introduce medicinally relevant amino groups (Scheme 2B).77 Importantly, these reagents could be synthesised on a multigram scale and were isolated as stable, crystalline solids.
The application of those novel reagents in aminochlorination reactions led to the synthesis of a broad range of densely functionalised amines. Furthermore, it was shown that these reagents can partake in other difunctionalisation reactions, such as aminohydroxylation, aminoazidation and a tethered carboamination reaction.77
Among aminofunctionalisation reactions, the installation of fluoride along with an unprotected amine on unactivated alkenes has posed a long term challenge.78,79 Recently, the Fu lab reported the synthesis of tertiary 2-fluoroamines from alkenes in a silver mediated transformation using terpyridine ligands (Scheme 2C).80
A different approach towards the construction of tertiary β-(pseudo)haloamines (X = Cl, Br, N3) was disclosed by a collaboration between the groups of Guan, Bi, and Fu (Scheme 2D).81 A suitably positioned 8-aminoquinoline directing group assisted the Cu catalysed activation of the olefin in β,γ-position. In that process, N-haloamines R14 provided both the amino group and the halide. With the addition of exogenous azide, aminoazidation was observed which resulted in the first report of a one-step, unprotected aminoazidation for tertiary amine synthesis. The reaction allowed the installation of several amines but always required a bulky directing group, thus limiting its scope.
An N-chloroamine based strategy for the synthesis of a wide range of diamines from alkenes was described by Leonori and co-workers (Scheme 2E).82 In a one-pot reaction, an amine was first N-chlorinated before being added across an olefin via a photochemical, radical aminochlorination reaction. The resulting 2-chloroamines could be transformed in the same pot into an aziridinium intermediate, which was prone to open with various N-nucleophiles to yield heterodiamines. This protocol elegantly combines the broad functional group tolerance of the aminochlorination step with the wide range of easily accessible secondary amino nucleophiles. However, this transformation showed limitations when a sterically less demanding amine was used as the initial nitrogen source. In those cases, an alternative amino surrogate might be preferred (e.g. N-substituted hydroxylammonium reagents, vide supra).77
Recent work by other groups presents additional examples for the construction of heterodiamines via an aziridinium intermediate.83–85
2.1.3 Masked diamination of alkenes.
A direct approach to vicinal diamines with distinguishable amino groups is the installation of a masked amino motif on an alkene along with an amino group.86 Examples for such a masked amino moiety could be, for example, an orthogonally protected amine,87–91 a thiocyanate,92 or an azido group. Theoretically, both nitrogen groups could be masked in an identical way,93–96 however, challenging regioselective unmasking renders this approach less appealing.97
Considering an azido group as a masked amine resulted in several recent reports of aminoazidation reactions which are either intramolecular98–101 or afford the amine in a protected form, such as a bissulfonamide.102–105 An ideal masked diamine however would consist of one free amino function.
Based on the results of our aminochlorination reaction, we envisioned that the very same aminating agent (PONT, R7) might be capable to facilitate the formation of unprotected, 2-azidoamines in combination with sodium azide (Scheme 3).106 The same regioselectivity as in the aminochlorination reaction, i.e. the amino group being introduced at the less substituted carbon, was observed under iron catalysis and an extensive investigation of the alkene scope revealed that even a complex olefin-containing tripeptide and a polyene probe could be aminated with excellent chemoselectivity. The utility of the resulting 2-azidoamines was demonstrated in subsequent independent transformations of both nitrogen functionalities and the synthesis of important diamino structures, such as triazoles, diamines, ureas and azidopiperidines. Its practicality was further showcased by applying the methodology in the (formal) synthesis of three bioactive molecules. Preliminary mechanistic experiments hinted towards a radical pathway without the involvement of a subsequent carbocationic intermediate.
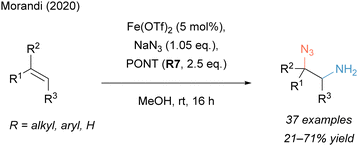 |
| Scheme 3 Unprotected aminoazidation of alkenes. | |
2.1.4 Aminohydroxylation of alkenes.
Besides 1,2-diamines, 1,2-amino alcohols are prevalent structures in bioactive molecules as well as catalysis.107 Their synthesis from alkenes was pioneered by Sharpless and co-workers using chloramine-T as a nitrogen source to install the amine in its sulfonamide-protected form using an Os catalyst.108
Following up on Cu and Fe catalysed work by Yoon and co-workers,109–111 the Xu lab reported an aminooxygenation reaction yielding carbamate protected amines.112,113 In an Fe catalysed reaction with pybox ligands, an N-carbamate and an O-acyl group were introduced in a variety of alkenes and dienes. Enantioselectivity could also be induced when using a chiral box or pybox ligand. Despite not yielding the unprotected amino alcohol as the primary product, this can be easily revealed by subsequent hydrolysis.
This concept to introduce an O-acyl group as an oxygen surrogate in aminooxygenation reactions has been a recurring strategy.114–116
In 2016, our group disclosed an aminohydroxylation reaction which afforded N- and O-unprotected aminoalcohols from styrenes and 1,1-disubstituted alkenes (Scheme 4A).117,118 A key element of this transformation was the use of a robust Fe catalyst (iron phthalocyanine, FePc) and PONT (R7) to deliver an unprotected amine. The aqueous solvent mixture served as a source of the hydroxyl group in the process. Alternatively, the use of an alcoholic medium led to O-alkylated products. The reaction found application in a synthetic route of drug molecules which started from cashew nut shell liquid as renewable, green feedstock.119 Recently, a collaboration between our group and the groups of Neese and DeBeer revealed important mechanistic features of this reaction.120
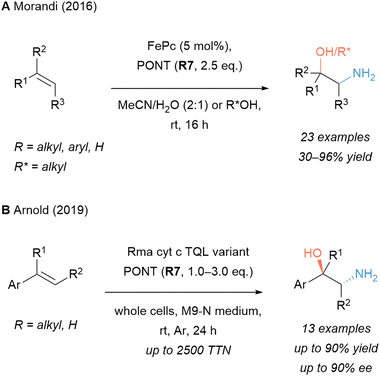 |
| Scheme 4 Selected aminooxygenation reactions of alkenes with hydroxylamine derived reagents. tfa: trifluoroacetate; Ns: p-nitrobenzenesulfonamide; Pc: phthalocyanine. | |
Inspired by the structural similarities between the Pc ligand and the structure of hemoproteins, the Arnold group developed an asymmetric variant of the aminohydroxylation reaction of vinyl arenes (Scheme 4B).121 Opting for a haemoprotein, a very thermostable Rhodotermus marinus cytochrome C enzyme was selected as a starting point for directed evolution. A bioengineered catalyst was created which resulted in excellent enantioselectivity and total turnover numbers (TTN) of up to 2500 in the asymmetric aminohydroxylation of alkenes with PONT (R7) as the aminating reagent.
2.2 Intermolecular aromatic C–H amination
The rise of novel hydroxylamine-derived aminating reagents in the last 20 years has also paved the way for innate C–H amination reactions catalysed by noble as well as non-noble transition metals. Early reports of innate C(sp2)–H amination were limited in scope, partly because the aromatic substrate was used in excess.12 Further development unlocked the synthesis of free primary anilines under mild conditions. In addition to innate C–H aminations, methodologies have also emerged that target the functionalization of arenes at the ortho-, meta- and para-position.
2.2.1 Noble metal-catalysed aromatic C–H amination.
A seminal report in the area of innate C–H amination with only 1 equivalent of arene was disclosed by the Ritter group in 2013.122 They employed an amine-N-oxide-ligated palladium complex, combined with a Ag co-catalyst, for the generation of a wide range of sulfonamides. Glorius and co-workers realised the Rh(III) catalysed amination of arenes possessing a pyridine or O-methyl hydroxamic acid directing group by employing an N-Boc-protected hydroxylamine-derived aminating reagent.123 In 2016, Kürti and Falck reported the generation of free primary and N-alkyl arylamines catalysed by a rhodium complex. This reaction is proposed to proceed via a metal–nitrenium intermediate.124 An example of the Rh catalysed, para-selective C–H amination of alkoxyarenes was reported by the Kawabata group in 2018.125
2.2.2 Earth-abundant metal-catalysed aromatic C–H amination.
Earth-abundant metals such as Na, Al, Ti, Fe, Ni, Cu, or Hg have been known to promote innate arene C–H amination for several decades. One of the first amination strategies employing an N–X reagent was the reaction of hydroxylamine O-sulfonic acid (HOSA, R1) with arenes in the presence of aluminium chloride, developed by Keller in 1944 and Bennett in 1961 (Scheme 5).126,127 Other early examples of innate aromatic C–H amination with hydroxylamine-derived reagents include homolytic aromatic amination reactions involving amino radical cations generated from N–Cl substrates in a strongly acidic environment (H2SO4) or mediated by Na(I), Ti(IV), Fe(II), Cu(I), Ni(II), and Hg(I) salts, as reported by Bock and Minisci.128–131 The utility of these early reports remained limited due to the excess of arene employed, and the need of Brønsted or Lewis acids.
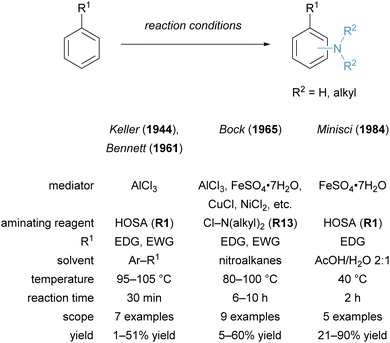 |
| Scheme 5 Early examples of innate C–H arene amination. | |
In recent years, significant progress has been made in Fe catalysed amination reactions, employing hydroxylamine-derived aminating reagents and using arenes as the limiting reagent. A mild procedure for the imidation of (hetero)arenes, catalysed by ferrocene and employing N-succinimidyl perester (NSP, R17) as the aminating reagent, was reported by Baran and co-workers in 2014.34 This strategy proceeding via a radical pathway enabled the reaction to work on a wider range of substrates (including various heteroarenes) than previously reported methods, yet it still required a deprotection step of the succinimide to unmask the free amine. The innate Fe catalysed arene C–H amination to generate free anilines was published by our group in 2016 using the hydroxylamine-derived reagent MsONH3OTf (R4) (Scheme 6A).20 The use of an earth-abundant metal under mild conditions enabled the direct access to a plethora of primary anilines and is a more cost-efficient method for analogous Rh catalysed transformations. This amination strategy was also subsequently applied in the total synthesis of di- and sesquiterpenoids by the Dong group in 2020.132 We also reported the Fe catalysed C–H amination for the synthesis of N-methylanilines from simple arenes using electrophilic aminating reagent NsONH2MeOTf (R5-Me).133 More recently, the Xu and Huang groups reported the C–H amination of (hetero)arenes under photoredox catalysis to form protected trifluoromethylamines.134
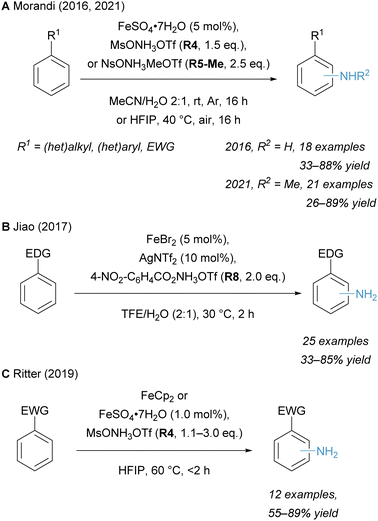 |
| Scheme 6 Fe catalysed C–H amination of arenes. | |
With the development of new Fe catalysed innate C–H amination reactions a variety of new aminating reagents was introduced, each tailored for different kinds of transformations. In 2017, Jiao and co-workers introduced a new aminating reagent 4-NO2–C6H4CO2NH3OTf (R8), which enabled the Fe catalysed C–H amination of electron-rich and -neutral arenes as well as heteroarenes (Scheme 6B).21 Mechanistic studies suggest that the transformation is enabled via the formation of an amino radical species. While only electron-rich or -neutral substrates were suitable for the generation of primary anilines, Ritter and co-workers realised the amination of electron-poor arenes in 2019 by modifying our previously reported conditions.16 Hexafluoroisopropanol (HFIP), which disrupts ion pairing through hydrogen bonding interactions, proved to be essential for the successful C–H amination of these more inert substrates (Scheme 6C).135
Notable aminating strategies utilising non-noble transition metals, other than iron, have been developed in recent years. In 2019, the group of Falck employed catalytic Cu(OTf)2 for the synthesis of free primary and secondary anilines (Scheme 7A).49 This offers a cost-effective alternative to the established methodologies with Rh catalysts. An alternative TiCl3-mediated amination strategy for the synthesis of free primary amines from arenes was reported by Sanford and co-workers in 2020 (Scheme 7B).136 This protocol complements Minisci's originally reported conditions by using the arene as the limiting reagent and provides an expanded substrate scope.
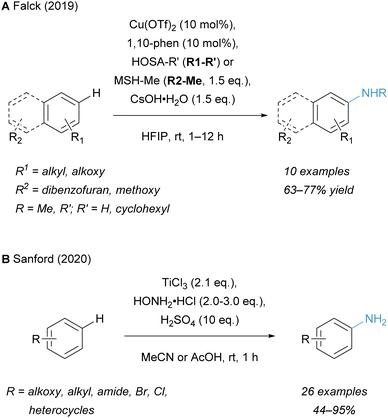 |
| Scheme 7 Arene C–H amination reactions using Cu(II) and Ti(III) salts. | |
2.2.3 Regioselective aromatic C–H amination.
A challenge that has been partially addressed in recent years is the regioselective C–H amination of arenes. A highly meta-selective arene C–H amination strategy, assisted by a picolinate directing group, was reported by Falck and co-workers in 2021 (Scheme 8A).137 One of the most recent advances in generating ortho-substituted primary amines over meta- or para-substituted ones is the regioselective arene ortho-amination of sulfamate-protected anilines developed by Phipps and co-workers (Scheme 8B). This directing group can easily be deprotected under acidic conditions.25 This work makes use of electrostatic and hydrogen bonding interactions between the aminium radical cation generated from a wide range of known and novel reagents (R4, R7–R9) and the anionic arene sulfamate substrate in HFIP.
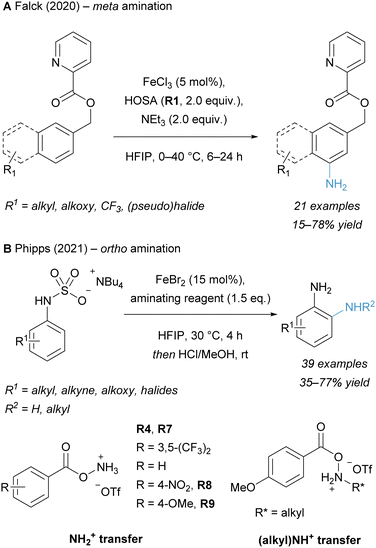 |
| Scheme 8 Fe catalysed regioselective arene C–H amination at the ortho- and para-position. | |
Several reports have been published to achieve the para-selective C–H amination of arenes using photoredox catalysis. These reactions, proceeding via the formation of highly electrophilic aminium radicals from N-haloamines, have been reported by the groups of Ritter (Scheme 9A)138 and Leonori (Scheme 9B)139 to generate para-substituted aryl piperazines and other tertiary amines. In the former report, selectfluor is used and thus necessitates a reduction step to unmask the diazoniabicyclo[2.2.2]octane salts into free piperazines. Therefore, a general protocol for the para-selective synthesis of free primary anilines via C–H amination with a broad substrate scope is still lacking.
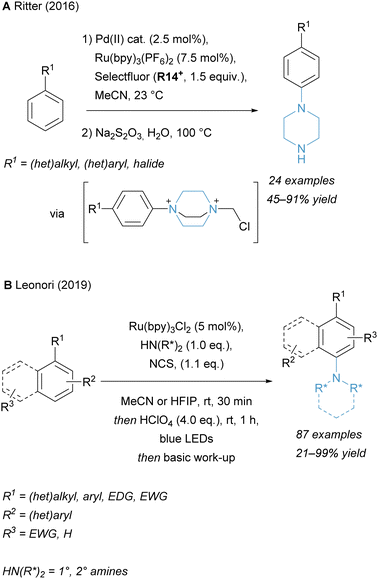 |
| Scheme 9 Regioselective, photocatalysed arene C–H amination in para-position. | |
2.2.4 Arylpyridinium ions as reactive intermediates in innate C–H amination reactions.
The direct access of unprotected primary amines via arylpyridinium intermediates has also attracted the attention of several research groups. A photocatalytic approach was independently developed by the Carreira and Ritter groups in 2019, in which the direct C–H amination of arenes is enabled via photocatalytically generated pyridyl radical cations (Scheme 10).35,36
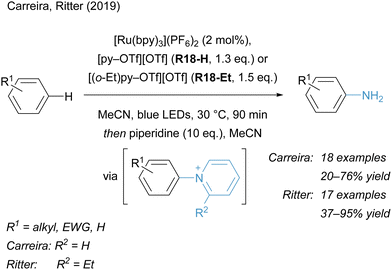 |
| Scheme 10 C–H amination of arenes via arylpyridinium intermediates. | |
2.2.5 Intramolecular aromatic C–H amination.
Heterocyclic compounds are extremely relevant in the pharmaceutical and agrochemical industry.140 Several procedures for synthesizing indoles,141,142 carbazoles,143,144 indolines,145,146 and benzazetidines147 have been developed in the last decades via C–H arene amination. Next to these and the ones mentioned in the previous sections, another building block present in a wide range of biologically active natural and unnatural products is the tetrahydroquinoline motif.148,149 The group of Minisci reported the intramolecular homolytic C–H amination by N-chloroamines (R14) in the presence of ferrous sulfate in concentrated sulphuric acid to afford indolines and tetrahydroquinolines already in 1966 (Scheme 11A).129 The group of Kikugawa applied the AlCl3-mediated intramolecular C–H arene amination to the synthesis of indolines and tetrahydroquinolines from alkylhydroxylamine derivatives in 1981 (Scheme 11B).150
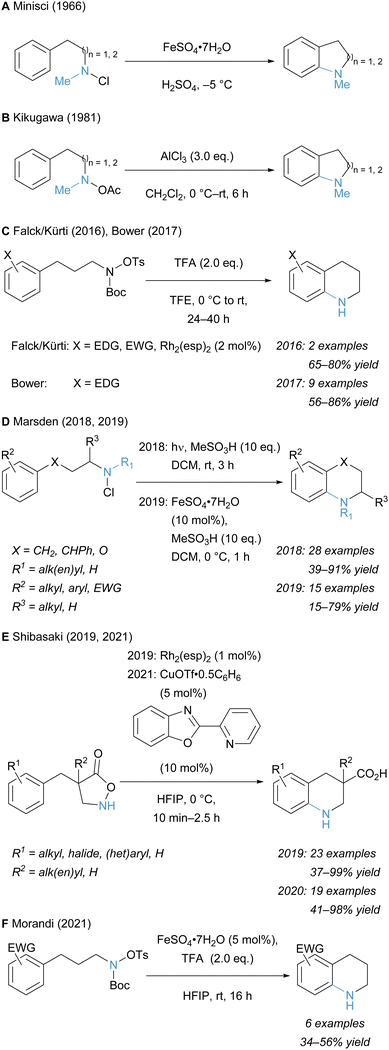 |
| Scheme 11 Synthesis of tetrahydroquinolines. | |
These early examples laid the foundation for later advances in the generation of tetrahydroquinolines via C–H amination. In 2016, the Falck and Kürti groups developed an intramolecular Rh catalysed C–H amination of electron-rich and -poor arenes to afford several free tetrahydroquinolines. Thereafter, a metal-free procedure for the intramolecular C–H amination of electron-neutral as well as electron-rich arenes was developed by the Bower group (Scheme 11C). They propose that these substrates maintain sufficient nucleophilicity to approach the electrophilic amine moiety in an SEAr fashion.151 The Fe catalysed intramolecular C–H amidation of arenes for the generation of arylcarbamates was reported by Singh and co-workers in 2018. Mechanistic investigations and DFT calculations suggest a reaction pathway that likely proceeds via nitrene formation upon N–O bond cleavage.152 The Marsden group reported the synthesis of tetrahydroquinolines from secondary alkylamines through the in situ formation of N-chloroamines with N-chlorosuccinimide (Scheme 11D). In the presence of methanesulfonic acid, these can undergo a light-mediated cyclization.153 Their methodology was also applied in the synthesis of polycyclic substrates, whose skeleton is present in various complex alkaloids. Furthermore, continuous flow conditions drastically improved the efficiency of the reaction.154 The same group reported the Fe catalysed intramolecular aromatic C–H amination of N-chloroamines with methanesulfonic acid to generate tertiary tetrahydroquinolines.155
The group of Shibasaki exploited the labile N–O bond of hydroxylamine-derived compounds and developed the Rh catalysed synthesis of novel β-amino acids, which were generated from initial N–O bond cleavage in the substrate, followed by intramolecular C–H arene amination of the liberated amine moiety (Scheme 11E).156 With this method a variety of tetrahydroquinoline derivatives and spirocyclic β-amino acids could be prepared. An analogous protocol with a Cu catalyst was developed, thus enabling higher chemoselectivity than with the more reactive Rh catalyst. The reaction is proposed to proceed via a dicopper alkyl nitrene.157
The groups of Zhang, Chai, and Zhang developed the electrochemical cyclization of aryl sulfonamides to benzosultams via intramolecular aromatic C–H amination.158
In our recent publication on the Fe-mediated N-methylamination of arenes, we also reported the synthesis of electron-poor tetrahydroquinolines in the presence of trifluoroacetic acid in HFIP (Scheme 11F).133 This procedure unlocks the functionalization of electron-poor arenes in a more cost-effective fashion compared to methods relying on the less abundant rhodium metal.159
2.3 Amination of sulfur compounds
In addition to carbon nucleophiles, sulfur-bearing functionalities can be transformed with hydroxylamine-derived reagents to generate a variety of value-added aminated sulfur compounds.160,161
2.3.1 Sulfoximines.
A prominent example of this transformation is the generation of sulfoximines from sulfoxides (Scheme 12).162,163 In search of a safer alternative to hazardous reagents (e.g.R2 (MSH)164) the group of Richards and Ge demonstrated the use of R10 (DPH) in a Rh catalysed transformation (Scheme 12A) in 2014.165 (Hetero)aromatic and aliphatic thioethers afforded the desired product in high yields, whereas steric bulk at the ortho-position hampered the reaction.
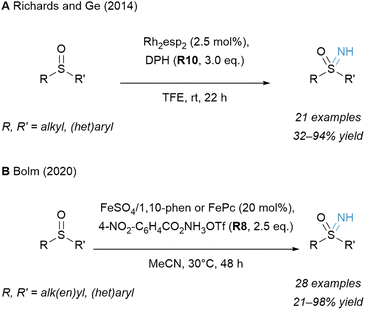 |
| Scheme 12 Amination of sulfoxides. | |
A more cost-effective method was disclosed a few years later by Bolm and co-workers (Scheme 12B).166 An Fe based catalytic system with a phenanthroline (phen) or a phthalocyanine (Pc) ligand structure performed effectively. Though O-pivaloyl-substituted hydroxylammonium reagent PONT (R7) gave the desired product in good yield, the reaction could be further improved by switching to an O-benzoyl congener R8 (4-NO2–C6H4CO2NH3OTf).
2.3.2 Primary sulfinamides.
Traditionally, the conversion of free thiols to sulfinamides can be achieved by a two-step protocol that used either sulfenamides167 or sulfinates168,169 as intermediates. A direct oxidative coupling approach with primary and secondary amines showed some over-oxidation of the sulfinamide group.170
Recently, our group reported that unprotected, primary sulfinamides could be selectively obtained in a mild Fe catalysed process under ambient conditions from thiols by employing PONT (R7) (Scheme 13).171 This process allowed the use of a wide range of thiols: not only were thiophenols transformed in excellent yields, but also primary, secondary, tertiary as well as benzylic thiols all reacted to give the respective sulfinamide product without any overoxidation to sulfonamides. Considering related Fe catalysed transformations with this reagent (see Section 2.1), it is remarkable that alkene groups remained intact using this protocol.
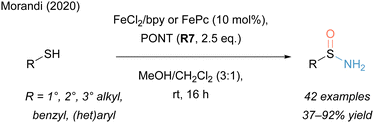 |
| Scheme 13 Aminooxygenation of thiols. NCS: N-chlorosuccinimide; bpy: 2,2′-bipyridine. | |
A key mechanistic finding was that the aminating reagent acted beyond its usual function as a nitrogen source by also serving as an oxidant. The oxygen source could be traced back to the methanolic solvent system.
2.3.3 N–O reagents for C–S bond formation.
Though various methods have been reported to transform thiols into primary, unprotected sulfonamides,172–175 similar protocols employing a hydroxylamine-derived reagent are less known.
Recently, the laboratory of Willis has reported an example with a novel N-sulfinylhydroxylamine reagent that exhibits an N–O connectivity (Scheme 14A).176 This electrophilic O-tBu reagent (tBuONSO) already contained all the necessary atomic building blocks to generate a sulfonamide, including the sulfur core. The remaining C–S bond was then formed upon reaction with a strong carbon nucleophile such as a Grignard or organolithium species. Similarly, a novel N-silyl analogue was used to synthesise primary sulfinamides.177
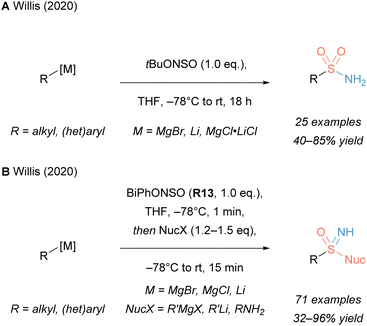 |
| Scheme 14 Application of hydroxylamine-derived reagents for the construction of C–S bonds. | |
O-Aryl reagent R13 by contrast exhibits a weaker N–O bond and thus differs in reactivity (Scheme 14B).30 The mechanistic experiments conducted by the group of Willis indicate that, upon attack of the first equivalent of an organometallic carbon nucleophile and loss of a phenolate, a sulfinyl nitrene species is formed. This could subsequently react further on the electrophilic sulfur with a different carbon or nitrogen nucleophile to yield unprotected sulfoximines and sulfonimidamides, respectively.
3 Conclusions and outlook
The reports discussed above clearly show that hydroxylamine-based reagents are a privileged class of electrophilic aminating species for the preparation of important amine building blocks. They are particularly attractive reagents enabling the direct synthesis of unprotected amines.
However, this should not discourage to envision new reagents which use the hydroxylamine handle to introduce transiently protected amines which can easily be deprotected. Recent examples are Fier's reagent (R12) for alpha-carbamation of pyridines29 and an improved version of NFSI by the Hashimoto group (NFC, R16).178
In most cases, hydroxylamine-derived reagents exhibit scalable syntheses, air and temperature stability and a broad range of applications. Their practicality is further increased as they tend to be easy-to-handle solids with an extended shelf life. In contrast, N-chloro and N-bromo activated reagents suffer from low stability and availability. While methods directly using simple amines in photo-179 or electrochemical180,181 reactions have recently emerged for the formation of C–N bonds, solid hydroxylamine-derived reagents may represent a more convenient alternative for various applications. Especially in the case of low-molecular amino functions, hydroxylamine-derived reagents are less volatile and hazardous than their free amine and azide counterparts, making them ideal reagents for discovery projects and late-stage functionalisations. Moreover, their weak N–O bond enables facile and selective functionalisation under mild reaction conditions.
For all these reasons, it is important to further investigate methods to synthesise these reagents, especially with regard to developing new versatile methods for the construction of the N–O connectivity.182 This, in turn, will provide access to more functionalised reagents which will bring about the design of new catalytic reactions.
A common limitation of several of the presented amination reactions employing N–O species is the use of perfluorinated reagents and/or solvents to achieve enhanced reactivity. It would be very desirable to find cheaper and environmentally more benign alternatives which mimic the special reactivity of e.g. hexafluoroisopropanol183 to facilitate the application of these reactions on larger scales.
Another challenge in the field is that several synthetically useful transformations have remained elusive. For example, asymmetric transformations enabling the direct introduction of unprotected amines remain scarce, probably due to the additional challenges of controlling enantioselectivity in reactions that often involve radical intermediates.184,185
Finally, there is a clear need for additional mechanistic studies, particularly for reactions employing first-row transition metals. A deeper understanding of these reactions could serve to improve their scope, as well as unlock completely new transformations.
In conclusion, we hope this feature article will serve as an inspiration for the future development of new transformations that will streamline the discovery and synthesis of important amines. Seeing the recent increase of reports in this research area, we are confident that the challenges mentioned above will be swiftly addressed by the synthetic community.
Conflicts of interest
There are no conflicts of interest to declare.
Acknowledgements
Financial support from the Swiss National Science Foundation (SNSF 184658) and ETH Zürich is gratefully acknowledged.
Notes and references
- N. A. McGrath, M. Brichacek and J. T. Njardarson, J. Chem. Educ., 2010, 87, 1348–1349 CrossRef CAS.
- L. Legnani, B. N. Bhawal and B. Morandi, Synthesis, 2016, 776–789 Search PubMed.
- S. Makai and B. Morandi, Encycl. Reagents Org. Synth., 2021, 1–3 Search PubMed.
- N. Guimond, S. I. Gorelsky and K. Fagnou, J. Am. Chem. Soc., 2011, 133, 6449–6457 CrossRef CAS PubMed.
- G. Zinner, Arch. Pharm., 1960, 293, 657–661 CrossRef CAS.
- S. Makai, E. Falk and B. Morandi, Org. Synth., 2020, 97, 207–216 CrossRef CAS.
- L. G. O’Neil and J. F. Bower, Angew. Chem., Int. Ed., 2021, 60, 25640–25666 CrossRef PubMed.
- S. Sabir, G. Kumar and J. L. Jat, Org. Biomol. Chem., 2018, 16, 3314–3327 RSC.
- J. Davies, S. P. Morcillo, J. J. Douglas and D. Leonori, Chem. – Eur. J., 2018, 24, 12154–12163 CrossRef CAS PubMed.
- D. Jinan, P. P. Mondal, A. V. Nair and B. Sahoo, Chem. Commun., 2021, 57, 13495–13505 RSC.
- K. Hirano and M. Miura, J. Am. Chem. Soc., 2022, 144, 648–661 CrossRef CAS PubMed.
- Y. Park, Y. Kim and S. Chang, Chem. Rev., 2017, 117, 9247–9301 CrossRef CAS PubMed.
- J. Jiao, K. Murakami and K. Itami, ACS Catal., 2016, 6, 610–633 CrossRef CAS.
- C. Pratley, S. Fenner and J. A. Murphy, Chem. Rev., 2022, 122, 8181–8260 CrossRef CAS PubMed.
-
A. Ricci, Methodologies in Amine Synthesis: Challenges and Applications, Wiley-VCH, Weinheim, Germany, 2021 Search PubMed.
- T. Xiong and Q. Zhang, Chem. Soc. Rev., 2016, 45, 3069–3087 RSC.
- A. Trowbridge, S. M. Walton and M. J. Gaunt, Chem. Rev., 2020, 120, 2613–2692 CrossRef CAS PubMed.
- R. N. Keller and P. A. S. Smith, J. Am. Chem. Soc., 1944, 66, 1122–1124 CrossRef CAS.
- L. A. Carpino, J. Am. Chem. Soc., 1960, 82, 3133–3135 CrossRef CAS.
- L. Legnani, G. P. Cerai and B. Morandi, ACS Catal., 2016, 6, 8162–8165 CrossRef CAS.
- J. Liu, K. Wu, T. Shen, Y. Liang, M. Zou, Y. Zhu, X. Li, X. Li and N. Jiao, Chem. – Eur. J., 2017, 23, 563–567 CrossRef CAS PubMed.
- A. M. Berman and J. S. Johnson, J. Am. Chem. Soc., 2004, 126, 5680–5681 CrossRef CAS PubMed.
- C. Grohmann, H. Wang and F. Glorius, Org. Lett., 2013, 15, 3014–3017 CrossRef CAS PubMed.
- A. S. Radhakrishna, G. M. Loudon and M. J. Miller, J. Org. Chem., 1979, 44, 4836–4841 CrossRef CAS.
- J. E. Gillespie, C. Morrill and R. J. Phipps, J. Am. Chem. Soc., 2021, 143, 9355–9360 CrossRef CAS PubMed.
- L. Parlanti, R. P. Discordia, J. Hynes, M. M. Miller, H. R. O’Grady and Z. Shi, Org. Lett., 2007, 9, 3821–3824 CrossRef CAS PubMed.
- T. Sheradsky, G. Salemnick and Z. Nir, Tetrahedron, 1972, 28, 3833–3843 CrossRef CAS.
- S. Guo, J. C. Yang and S. L. Buchwald, J. Am. Chem. Soc., 2018, 140, 15976–15984 CrossRef CAS PubMed.
- P. S. Fier, S. Kim and R. D. Cohen, J. Am. Chem. Soc., 2020, 142, 8614–8618 CrossRef CAS PubMed.
- T. Q. Davies, M. J. Tilby, J. Ren, N. A. Parker, D. Skolc, A. Hall, F. Duarte and M. C. Willis, J. Am. Chem. Soc., 2020, 142, 15445–15453 CrossRef CAS PubMed.
- G. H. Coleman, J. Am. Chem. Soc., 1933, 55, 3001–3005 CrossRef CAS.
- P. A. Sibbald and F. E. Michael, Org. Lett., 2009, 11, 1147–1149 CrossRef CAS PubMed.
- Y. Oe, R. Yoshida, A. Tanaka, A. Adachi, Y. Ishibashi, T. Okazoe, K. Aikawa and T. Hashimoto, J. Am. Chem. Soc., 2022, 144, 2107–2113 CrossRef CAS PubMed.
- K. Foo, E. Sella, I. Thomé, M. D. Eastgate and P. S. Baran, J. Am. Chem. Soc., 2014, 136, 5279–5282 CrossRef CAS PubMed.
- W. S. Ham, J. Hillenbrand, J. Jacq, C. Genicot and T. Ritter, Angew. Chem., Int. Ed., 2019, 58, 532–536 CrossRef CAS PubMed.
- S. L. Rössler, B. J. Jelier, P. F. Tripet, A. Shemet, G. Jeschke, A. Togni and E. M. Carreira, Angew. Chem., Int. Ed., 2019, 58, 526–531 CrossRef PubMed.
- S. Sabir, G. Kumar and J. L. Jat, Asian J. Org. Chem., 2017, 6, 782–793 CrossRef CAS.
- Z. Wu, M. Hu, J. Li, W. Wu and H. Jiang, Org. Biomol. Chem., 2021, 19, 3036–3054 RSC.
- S. Zhu, N. Niljianskul and S. L. Buchwald, J. Am. Chem. Soc., 2013, 135, 15746–15749 CrossRef CAS PubMed.
- R. Y. Liu and S. L. Buchwald, Acc. Chem. Res., 2020, 53, 1229–1243 CrossRef CAS PubMed.
- Y. Yang, S. L. Shi, D. Niu, P. Liu and S. L. Buchwald, Science, 2015, 349, 62–66 CrossRef CAS PubMed.
- Y. Miki, K. Hirano, T. Satoh, M. Miura, Y. Miki, K. Hirano, T. Satoh and M. Miura, Angew. Chem., Int. Ed., 2013, 52, 10830–10834 CrossRef CAS PubMed.
- P. Métra and J. Hamelin, J. Chem. Soc., Chem. Commun., 1980, 1038–1039 RSC.
- J. C. Bottaro, J. Chem. Soc., Chem. Commun., 1980, 560–561 RSC.
- J. Mendiola, J. A. Rincon, C. Mateos, J. F. Soriano, O. De Frutos, J. K. Niemeier and E. M. Davis, Org. Process Res. Dev., 2009, 13, 263–267 CrossRef CAS.
- J. L. Jat, M. P. Paudyal, H. Gao, Q. L. Xu, M. Yousufuddin, D. Devarajan, D. H. Ess, L.
urti and J. R. Falck, Science, 2014, 343, 61–65 CrossRef CAS PubMed.
- Z. Ma, Z. Zhou and L. Kürti, Angew. Chem., Int. Ed., 2017, 56, 9886–9890 CrossRef CAS PubMed.
- S. Sabir, C. B. Pandey, A. K. Yadav, B. Tiwari and J. L. Jat, J. Org. Chem., 2018, 83, 12255–12260 CrossRef CAS PubMed.
- S. Munnuri, R. R. Anugu and J. R. Falck, Org. Lett., 2019, 21, 1926–1929 CrossRef CAS PubMed.
- D. Chandra, A. K. Yadav, V. Singh, B. Tiwari and J. L. Jat, ChemistrySelect, 2021, 6, 10524–10526 CrossRef CAS.
- Q. Q. Cheng, Z. Zhou, H. Jiang, J. H. Siitonen, D. H. Ess, X. Zhang and L. Kürti, Nat. Catal., 2020, 3, 386–392 CrossRef CAS.
- Y. Zhu, Q. Wang, R. G. Cornwall and Y. Shi, Chem. Rev., 2014, 114, 8199–8256 CrossRef CAS PubMed.
- J. J. Farndon, T. A. Young and J. F. Bower, J. Am. Chem. Soc., 2018, 140, 17846–17850 CrossRef CAS PubMed.
- R. Liu, S. R. Herron and S. A. Fleming, J. Org. Chem., 2007, 72, 5587–5591 CrossRef CAS PubMed.
- H. Lebel, S. Lectard and M. Parmentier, Org. Lett., 2007, 9, 4797–4800 CrossRef CAS PubMed.
- H. Lebel, K. Huard and S. Lectard, J. Am. Chem. Soc., 2005, 127, 14198–14199 CrossRef CAS PubMed.
- E. Azek, C. Spitz, M. Ernzerhof and H. Lebel, Adv. Synth. Catal., 2020, 362, 384–397 CrossRef CAS.
- H. Lebel, M. Parmentier, O. Leogane, K. Ross and C. Spitz, Tetrahedron, 2012, 68, 3396–3409 CrossRef CAS.
- S. Fioravanti, A. Morreale, L. Pellacani and P. A. Tardella, C. R. Chim., 2005, 8, 845–847 CrossRef CAS.
- A. Koohang, C. L. Stanchina and R. M. Coates, Tetrahedron, 1999, 55, 9669–9686 CrossRef CAS.
- S. Lee, H. Lei and T. Rovis, J. Am. Chem. Soc., 2019, 141, 12536–12540 CrossRef CAS PubMed.
- G. Kirby, L. Grimaud, M. R. Vitale, G. Prestat and F. Berhal, Green Chem., 2021, 23, 9428–9432 RSC.
- F. Pesciaioli, F. De Vincentiis, P. Galzerano, G. Bencivenni, G. Bartoli, A. Mazzanti, P. Melchiorre, F. Pesciaioli, F. De Vincentiis, P. Galzerano, G. Bencivenni, G. Bartoli, A. Mazzanti and P. Melchiorre, Angew. Chem., Int. Ed., 2008, 47, 8703–8706 CrossRef CAS PubMed.
- A. Armstrong, C. A. Baxter, S. G. Lamont, A. R. Pape and R. Wincewicz, Org. Lett., 2007, 9, 351–353 CrossRef CAS PubMed.
- G. Herbert, J. Laboratory, M. S. Kharasch and H. M. Priestley, J. Am. Chem. Soc., 2002, 61, 3425–3432 Search PubMed.
- K. Schrage, Tetrahedron Lett., 1966, 7, 5795–5799 CrossRef.
- F. Minisci, R. Galli and M. Cecere, Tetrahedron Lett., 1966, 7, 3163–3166 CrossRef.
- S. R. Chemler and M. T. Bovino, ACS Catal., 2013, 3, 1076–1091 CrossRef CAS PubMed.
- J. Davies, N. S. Sheikh and D. Leonori, Angew. Chem., Int. Ed., 2017, 56, 13361–13365 CrossRef CAS PubMed.
- T. Bach, B. Schlummer and K. Harms, Chem. Commun., 2000, 287–288 RSC.
- H. Zhang, Y. Song, J. Zhao, J. Zhang, Q. Zhang, H. Zhang, Y. C. Song, J. B. Zhao, J. P. Zhang and Q. Zhang, Angew. Chem., Int. Ed., 2014, 53, 11079–11083 CrossRef CAS PubMed.
- G. C. Arteaga, J. Saavedra-Olavarría, S. Almendras, P. Hermosilla-Ibáñez, I. Almodovar and E. G. Pérez, Tetrahedron Lett., 2018, 59, 1091–1093 CrossRef CAS.
- S. Engl and O. Reiser, Org. Lett., 2021, 23, 5581–5586 CrossRef CAS PubMed.
- D. F. Lu, C. L. Zhu, J. D. Sears and H. Xu, J. Am. Chem. Soc., 2016, 138, 11360–11367 CrossRef CAS PubMed.
- C. L. Zhu, J. S. Tian, Z. Y. Gu, G. W. Xing and H. Xu, Chem. Sci., 2015, 6, 3044–3050 RSC.
- L. Legnani, G. Prina-Cerai, T. Delcaillau, S. Willems and B. Morandi, Science, 2018, 362, 434–439 CrossRef CAS PubMed.
- E. Falk, S. Makai, T. Delcaillau, L. Gürtler and B. Morandi, Angew. Chem., Int. Ed., 2020, 59, 21064–21071 CrossRef CAS PubMed.
- H. Jiang and A. Studer, Angew. Chem., Int. Ed., 2018, 57, 10707–10711 CrossRef CAS PubMed.
- D. F. Lu, C. L. Zhu, J. D. Sears and H. Xu, J. Am. Chem. Soc., 2016, 138, 11360–11367 CrossRef CAS PubMed.
- Y. Li, J. Bao, Y. Zhang, X. Peng, W. Yu, T. Wang, D. Yang, Q. Liu, Q. Zhang and J. Fu, Chem, 2022, 8, 1147–1163 CAS.
- Y. Li, Y. Liang, J. Dong, Y. Deng, C. Zhao, Z. Su, W. Guan, X. Bi, Q. Liu and J. Fu, J. Am. Chem. Soc., 2019, 141, 18475–18485 CrossRef CAS PubMed.
- S. Govaerts, L. Angelini, C. Hampton, L. Malet-Sanz, A. Ruffoni and D. Leonori, Angew. Chem., Int. Ed., 2020, 132, 15131–15138 CrossRef.
- S. Lee, Y. J. Jang, E. J. T. Phipps, H. Lei and T. Rovis, Synthesis, 2019, 1247–1252 Search PubMed.
- F. Béke, Á. Mészáros, Á. Tóth, B. B. Botlik and Z. Novák, Nat. Commun., 2020, 11, 5924 CrossRef PubMed.
- Y. Li, A. Ali, J. Dong, Y. Zhang, L. Shi, Q. Liu and J. Fu, Org. Lett., 2021, 23, 4072–4077 CrossRef CAS PubMed.
- S. R. S. Saibabu Kotti, C. Timmons and G. Li, Chem. Biol. Drug Des., 2006, 67, 101–114 CrossRef CAS PubMed.
- D. Wu, S. S. Cui, Y. Lin, L. Li and W. Yu, J. Org. Chem., 2019, 84, 10978–10989 CrossRef CAS PubMed.
- P. Wang, Y. Luo, S. Zhu, D. Lu and Y. Gong, Adv. Synth. Catal., 2019, 361, 5565–5575 CrossRef CAS.
- S. Minakata, H. Miwa, K. Yamamoto, A. Hirayama and S. Okumura, J. Am. Chem. Soc., 2021, 143, 4112–4118 CrossRef CAS PubMed.
- Á. Iglesias, E. G. Pérez and K. Muñiz, Angew. Chem., Int. Ed., 2010, 49, 8109–8111 CrossRef PubMed.
- D. E. Olson, J. Y. Su, D. A. Roberts and J. Du Bois, J. Am. Chem. Soc., 2014, 136, 13506–13509 CrossRef CAS PubMed.
- W. Guo, Q. Wang and J. Zhu, Angew. Chem., Int. Ed., 2021, 60, 4085–4089 CrossRef CAS PubMed.
- N. Fu, G. S. Sauer, A. Saha, A. Loo and S. Lin, Science, 2017, 357, 575–579 CrossRef CAS PubMed.
- Y.-A. Yuan, D.-F. Lu, Y.-R. Chen and H. Xu, Angew. Chem., Int. Ed., 2016, 128, 544–548 CrossRef.
- H. Zhou, W. Jian, B. Qian, C. Ye, D. Li, J. Zhou and H. Bao, Org. Lett., 2017, 19, 6120–6123 CrossRef CAS PubMed.
- C. Röben, J. A. Souto, Y. González, A. Lishchynskyi and K. Muñiz, Angew. Chem., Int. Ed., 2011, 50, 9478–9482 CrossRef PubMed.
- P. T. Nyffeler, C. H. Liang, K. M. Koeller and C. H. Wong, J. Am. Chem. Soc., 2002, 124, 10773–10778 CrossRef CAS PubMed.
- F. C. Sequeira, B. W. Turnpenny and S. R. Chemler, Angew. Chem., Int. Ed., 2010, 49, 6365–6368 CrossRef CAS PubMed.
- K. Shen and Q. Wang, J. Am. Chem. Soc., 2017, 139, 13110–13116 CrossRef CAS PubMed.
- S. A. Fayssal, A. Giungi, F. Berhal and G. Prestat, Org. Process Res. Dev., 2020, 24, 695–703 CrossRef CAS.
- F. L. Wang, X. Y. Dong, J. S. Lin, Y. Zeng, G. Y. Jiao, Q. S. Gu, X. Q. Guo, C. L. Ma and X. Y. Liu, Chem, 2017, 3, 979–990 CAS.
- B. Lei, X. Wang, L. Ma, Y. Li and Z. Li, Org. Biomol. Chem., 2018, 16, 3109–3113 RSC.
- B. Zhang and A. Studer, Org. Lett., 2014, 16, 1790–1793 CrossRef CAS PubMed.
- D. Lv, Q. Sun, H. Zhou, L. Ge, Y. Qu, T. Li, X. Ma, Y. Li and H. Bao, Angew. Chem., Int. Ed., 2021, 60, 12455–12460 CrossRef CAS PubMed.
- D. Förster, W. Guo, Q. Wang and J. Zhu, ACS Catal., 2021, 11, 10871–10877 CrossRef.
- S. Makai, E. Falk and B. Morandi, J. Am. Chem. Soc., 2020, 142, 21548–21555 CrossRef CAS PubMed.
- S. C. Bergmeier, Tetrahedron, 2000, 56, 2561–2576 CrossRef CAS.
- G. Li, H.-T. Chang and K. B. Sharpless, Angew. Chem., Int. Ed. Engl., 1996, 35, 451–454 CrossRef CAS.
- K. S. Williamson and T. P. Yoon, J. Am. Chem. Soc., 2012, 134, 12370–12373 CrossRef CAS PubMed.
- D. J. Michaelis, C. J. Shaffer and T. P. Yoon, J. Am. Chem. Soc., 2007, 129, 1866–1867 CrossRef CAS PubMed.
- K. S. Williamson and T. P. Yoon, J. Am. Chem. Soc., 2010, 132, 4570–4571 CrossRef CAS PubMed.
- G. S. Liu, Y. Q. Zhang, Y. A. Yuan and H. Xu, J. Am. Chem. Soc., 2013, 135, 3343–3346 CrossRef CAS PubMed.
- D. F. Lu, C. L. Zhu, Z. X. Jia and H. Xu, J. Am. Chem. Soc., 2014, 136, 13186–13189 CrossRef CAS PubMed.
- S. Ren, S. Song, L. Ye, C. Feng and T. P. Loh, Chem. Commun., 2016, 52, 10373–10376 RSC.
- B. N. Hemric, K. Shen and Q. Wang, J. Am. Chem. Soc., 2016, 138, 5813–5816 CrossRef CAS PubMed.
- B. N. Hemric and Q. Wang, Beilstein J. Org. Chem., 2016, 12, 22–28 CrossRef CAS PubMed.
- L. Legnani and B. Morandi, Angew. Chem., Int. Ed., 2016, 55, 2248–2251 CrossRef CAS PubMed.
- M. K. Jackl, L. Legnani, B. Morandi and J. W. Bode, Org. Lett., 2017, 19, 4696–4699 CrossRef CAS PubMed.
- Y. Shi, P. C. J. Kamer and D. J. Cole-Hamilton, Green Chem., 2019, 21, 1043–1053 RSC.
- S. Chatterjee, I. Harden, G. Bistoni, R. G. Castillo, S. Chabbra, M. van Gastel, A. Schnegg, E. Bill, J. A. Birrell, B. Morandi, F. Neese and S. DeBeer, J. Am. Chem. Soc., 2022, jacs.1c11083 Search PubMed.
- I. Cho, C. K. Prier, Z. Jia, R. K. Zhang, T. Görbe and F. H. Arnold, Angew. Chem., Int. Ed., 2019, 131, 3170–3174 CrossRef.
- G. B. Boursalian, M.-Y. Ngai, K. N. Hojczyk and T. Ritter, J. Am. Chem. Soc., 2013, 135, 13278–13281 CrossRef CAS PubMed.
- C. Grohmann, H. Wang and F. Glorius, Org. Lett., 2013, 15, 3014–3017 CrossRef CAS PubMed.
- M. P. Paudyal, A. M. Adebesin, S. R. Burt, D. H. Ess, Z. Ma, L. Kürti and J. R. Falck, Science, 2016, 353, 1144–1147 CrossRef CAS PubMed.
- K. Arai, Y. Ueda, K. Morisaki, T. Furuta, T. Sasamori, N. Tokitoh and T. Kawabata, Chem. Commun., 2018, 54, 2264–2267 RSC.
- R. N. Keller and P. A. S. Smith, J. Am. Chem. Soc., 1944, 66, 1122–1124 CrossRef CAS.
- P. Kovacic and R. P. Bennett, J. Am. Chem. Soc., 1961, 83, 221–224 CrossRef CAS.
- H. Bock and K.-L. Kompa, Angew. Chem., Int. Ed. Engl., 1965, 4, 783 CrossRef.
- F. Minisci and R. Galli, Tetrahedron Lett., 1966, 7, 2531–2533 CrossRef.
- F. Minisci, Synthesis, 2002, 1–24 CrossRef.
- A. Citterio, A. Gentile, F. Minisci, W. Navarrini, M. Serravalle and S. Ventura, J. Org. Chem., 1984, 49, 4479–4482 CrossRef CAS.
- S.-H. Hou, A. Y. Prichina, M. Zhang and G. Dong, Angew. Chem., Int. Ed., 2020, 59, 7848–7856 CrossRef CAS PubMed.
- E. Falk, V. C. M. Gasser and B. Morandi, Org. Lett., 2021, 23, 1422–1426 CrossRef CAS PubMed.
- S. Liu, Y. Huang, J. Wang, F.-L. Qing and X.-H. Xu, J. Am. Chem. Soc., 2022, 144, 1962–1970 CrossRef CAS PubMed.
- E. M. D’Amato, J. Börgel and T. Ritter, Chem. Sci., 2019, 10, 2424–2428 RSC.
- Y. Y. See and M. S. Sanford, Org. Lett., 2020, 22, 2931–2934 CrossRef CAS PubMed.
- R. R. Anugu, S. Munnuri and J. R. Falck, J. Am. Chem. Soc., 2020, 142, 5266–5271 CrossRef CAS PubMed.
- G. B. Boursalian, W. S. Ham, A. R. Mazzotti and T. Ritter, Nat. Chem., 2016, 8, 810–815 CrossRef CAS PubMed.
- A. Ruffoni, F. Juliá, T. D. Svejstrup, A. J. McMillan, J. J. Douglas and D. Leonori, Nat. Chem., 2019, 11, 426–433 CrossRef CAS PubMed.
- E. Vitaku, D. T. Smith and J. T. Njardarson, J. Med. Chem., 2014, 57, 10257–10274 CrossRef CAS PubMed.
- A. S. Abreu, P. M. T. Ferreira, M.-J. R. P. Queiroz, I. C. F. R. Ferreira, R. C. Calhelha and L. M. Estevinho, Eur. J. Org. Chem., 2005, 2951–2957 CrossRef CAS.
- C. Yamamoto, K. Takamatsu, K. Hirano and M. Miura, J. Org. Chem., 2017, 82, 9112–9118 CrossRef CAS PubMed.
- S. H. Cho, J. Yoon and S. Chang, J. Am. Chem. Soc., 2011, 133, 5996–6005 CrossRef CAS PubMed.
- K. Takamatsu, K. Hirano, T. Satoh and M. Miura, Org. Lett., 2014, 16, 2892–2895 CrossRef CAS PubMed.
- K. Takamatsu, K. Hirano, T. Satoh and M. Miura, J. Org. Chem., 2015, 80, 3242–3249 CrossRef CAS PubMed.
- M. C. Henry, H. M. Senn and A. Sutherland, J. Org. Chem., 2019, 84, 346–364 CrossRef CAS PubMed.
- G. He, G. Lu, Z. Guo, P. Liu and G. Chen, Nat. Chem., 2016, 8, 1131–1136 CrossRef CAS.
- V. Sridharan, P. A. Suryavanshi and J. C. Menéndez, Chem. Rev., 2011, 111, 7157–7259 CrossRef CAS PubMed.
- I. Muthukrishnan, V. Sridharan and J. C. Menéndez, Chem. Rev., 2019, 119, 5057–5191 CrossRef CAS PubMed.
- M. Kawase and Y. Kikugawa, Chem. Pharm. Bull., 1981, 29, 1615–1623 CrossRef CAS.
- J. J. Farndon, X. Ma and J. F. Bower, J. Am. Chem. Soc., 2017, 139, 14005–14008 CrossRef CAS PubMed.
- A. V. G. Prasanthi, S. Begum, H. K. Srivastava, S. K. Tiwari and R. Singh, ACS Catal., 2018, 8, 8369–8375 CrossRef CAS.
- S. C. Cosgrove, J. M. C. Plane and S. P. Marsden, Chem. Sci., 2018, 9, 6647–6652 RSC.
- S. C. Cosgrove, G. E. Douglas, S. A. Raw and S. P. Marsden, ChemPhotoChem, 2018, 2, 851–854 CrossRef CAS.
- G. E. Douglas, S. A. Raw and S. P. Marsden, Eur. J. Org. Chem., 2019, 5508–5514 CrossRef CAS.
- J. S. Yu, M. Espinosa, H. Noda and M. Shibasaki, J. Am. Chem. Soc., 2019, 141, 10530–10537 CrossRef CAS PubMed.
- R. K. Tak, F. Amemiya, H. Noda and M. Shibasaki, Chem. Sci., 2021, 12, 7809–7817 RSC.
- A. Liu, T. Guo, S. Zhang, H. Yang, Q. Zhang, Y. Chai and S. Zhang, Org. Lett., 2021, 23, 6326–6331 CrossRef CAS PubMed.
- Y. Feng and N. Alonso-Vante, Phys. Status Solidi, 2008, 245, 1792–1806 CrossRef CAS.
- C. Lamberth, J. Sulphur Chem., 2010, 25, 39–62 CrossRef.
- U. Lücking, Angew. Chem., Int. Ed., 2013, 52, 9399–9408 CrossRef PubMed.
- V. Bizet, C. M. M. Hendriks and C. Bolm, Chem. Soc. Rev., 2015, 44, 3378–3390 RSC.
- M. Andresini, A. Tota, L. Degennaro, J. A. Bull and R. Luisi, Chem. – Eur. J., 2021, 27, 1–30 CrossRef PubMed.
- Y. Tamura, K. Sumoto, J. Minamikawa and M. Ikeda, Tetrahedron Lett., 1972, 13, 4137–4140 CrossRef.
- J. Miao, N. G. J. Richards and H. Ge, Chem. Commun., 2014, 50, 9687–9689 RSC.
- H. Yu, Z. Li and C. Bolm, Angew. Chem., Int. Ed., 2018, 130, 330–333 CrossRef.
- D. V. Sudarikov, Y. V. Krymskaya, N. O. Il’chenko, P. A. Slepukhin, S. A. Rubtsova and A. V. Kutchin, Russ. Chem. Bull., 2018, 67(4), 731–742 CrossRef CAS.
- B. M. Trost and J. R. Parquette, J. Org. Chem., 1993, 58, 1579–1581 CrossRef CAS.
- F. A. Davis, R. E. Reddy, J. M. Szewczyk, G. V. Reddy, P. S. Portonovo, H. Zhang, D. Fanelli, R. T. Reddy, P. Zhou and P. J. Carroll, J. Org. Chem., 1997, 62, 2555–2563 CrossRef CAS PubMed.
- N. Taniguchi, Eur. J. Org. Chem., 2016, 2157–2162 CrossRef CAS.
- S. Chatterjee, S. Makai and B. Morandi, Angew. Chem., Int. Ed., 2021, 60, 758–765 CrossRef CAS PubMed.
- A. Tota, S. St John-Campbell, E. L. Briggs, G. O. Estévez, M. Afonso, L. Degennaro, R. Luisi and J. A. Bull, Org. Lett., 2018, 20, 2599–2602 CrossRef CAS PubMed.
- E. Hayashi, Y. Yamaguchi, Y. Kita, K. Kamata and M. Hara, Chem. Commun., 2020, 56, 2095–2098 RSC.
- J. B. Feng and X. F. Wu, Org. Biomol. Chem., 2016, 14, 6951–6954 RSC.
- G. Laudadio, E. Barmpoutsis, C. Schotten, L. Struik, S. Govaerts, D. L. Browne and T. Noël, J. Am. Chem. Soc., 2019, 141, 5664–5668 CrossRef CAS PubMed.
- T. Q. Davies, M. J. Tilby, D. Skolc, A. Hall and M. C. Willis, Org. Lett., 2020, 22, 9495–9499 CrossRef CAS PubMed.
- M. Ding, Z.-X. Zhang, T. Q. Davies and M. C. Willis, Org. Lett., 2022, 24, 1711–1715 CrossRef CAS PubMed.
- Y. Oe, R. Yoshida, A. Tanaka, A. Adachi, Y. Ishibashi, T. Okazoe, K. Aikawa and T. Hashimoto, J. Am. Chem. Soc., 2022, 144, 2107–2113 CrossRef CAS PubMed.
- A. J. Musacchio, B. C. Lainhart, X. Zhang, S. G. Naguib, T. C. Sherwood and R. R. Knowles, Science, 2017, 355, 727–730 CrossRef CAS PubMed.
- M. Ošeka, G. Laudadio, N. P. van Leest, M. Dyga, A. de, A. Bartolomeu, L. J. Gooßen, B. de Bruin, K. T. de Oliveira and T. Noël, Chem, 2021, 7, 255–266 Search PubMed.
- D. E. Holst, D. J. Wang, M. J. Kim, I. A. Guzei and Z. K. Wickens, Nature, 2021, 596, 74–79 CrossRef CAS PubMed.
- A. Banerjee and H. Yamamoto, Chem. Sci., 2019, 10, 2124–2129 RSC.
- I. Colomer, A. E. R. Chamberlain, M. B. Haughey and T. J. Donohoe, Nat. Rev. Chem., 2017, 1, 1–12 CrossRef.
- S. Mondal, F. Dumur, D. Gigmes, M. P. Sibi, M. P. Bertrand and M. Nechab, Chem. Rev., 2022, 122, 5842–5976 CrossRef CAS PubMed.
- R. S. J. Proctor, A. C. Colgan and R. J. Phipps, Nat. Chem., 2020, 12, 990–1004 CrossRef PubMed.
Footnote |
† These authors contributed equally to this work. |
|
This journal is © The Royal Society of Chemistry 2022 |
Click here to see how this site uses Cookies. View our privacy policy here.