DOI:
10.1039/D1BM01756J
(Review Article)
Biomater. Sci., 2022,
10, 2734-2758
Advancing bone tissue engineering one layer at a time: a layer-by-layer assembly approach to 3D bone scaffold materials
Received
16th November 2021
, Accepted 19th March 2022
First published on 30th March 2022
Abstract
The layer-by-layer (LbL) assembly technique has shown excellent potential in tissue engineering applications. The technique is mainly based on electrostatic attraction and involves the sequential adsorption of oppositely charged electrolyte complexes onto a substrate, resulting in uniform single layers that can be rapidly deposited to form nanolayer films. LbL has attracted significant attention as a coating technique due to it being a convenient and affordable fabrication method capable of achieving a wide range of biomaterial coatings while keeping the main biofunctionality of the substrate materials. One promising application is the use of nanolayer films fabricated by LbL assembly in the development of 3-dimensional (3D) bone scaffolds for bone repair and regeneration. Due to their versatility, nanoscale films offer an exciting opportunity for tailoring surface and bulk property modification of implants for osseous defect therapies. This review article discusses the state of the art of the LbL assembly technique, and the properties and functions of LbL-assembled films for engineered bone scaffold application, combination of multilayers for multifunctional coatings and recent advancements in the application of LbL assembly in bone tissue engineering. The recent decade has seen tremendous advances in the promising developments of LbL film systems and their impact on cell interaction and tissue repair. A deep understanding of the cell behaviour and biomaterial interaction for the further development of new generations of LbL films for tissue engineering are the most important targets for biomaterial research in the field. While there is still much to learn about the biological and physicochemical interactions at the interface of nano-surface coated scaffolds and biological systems, we provide a conceptual review to further progress in the LbL approach to 3D bone scaffold materials and inform the future of LbL development in bone tissue engineering.
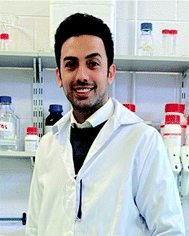 MohammadAli Sahebalzamani | MohammadAli Sahebalzamani is a PhD researcher at Dublin City University. His research, which is funded via the Irish Research Council – Government of Ireland PhD scholarship scheme (GOIPG/2020/1344), focusses on the development and characterisation of multifunctional bone scaffold-based materials fabricated by layer by layer assembly. |
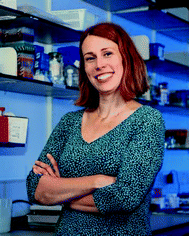 Monika Ziminska | Dr Monika Ziminska is a Postdoctoral Fellow in the School of Pharmacy at the Queen's University of Belfast. Dr Ziminska's research interests include understanding the deformation and failure of mechanically adaptive cellular materials. |
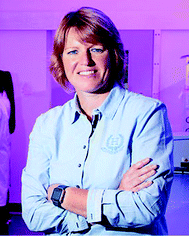 Helen O. McCarthy | Professor Helen O. McCarthy is a Full Professor and Chair of Nanomedicine in the School of Pharmacy at the Queen's University of Belfast. Prof McCarthy's lab works on the development and clinical translation of non-viral delivery systems for nanomedicine applications. |
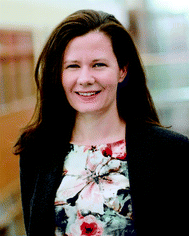 Tanya J. Levingstone | Dr Tanya Levingstone is an Assistant Professor in the School of Mechanical and Manufacturing Engineering at Dublin City University. Dr Levingstone's current research focusses on the development of novel biomaterials based approaches for bone and cartilage repair. |
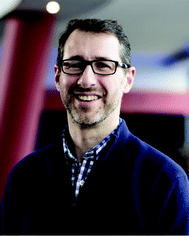 Nicholas J. Dunne | Professor Nicholas Dunne is a Full Professor and Chair of Biomaterials Engineering in the School of Mechanical and Manufacturing Engineering at Dublin City University. Prof Dunne leads a multidisciplinary research group focussed on the development of a nanomedicine-based medical device technology for the treatment and repair of diseased or damaged soft and hard tissue. |
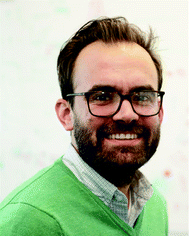 Andrew R. Hamilton | Dr Andrew R. Hamilton is an Associate Professor in Engineering and Physical Sciences at the University of Southampton. Dr Hamilton's research interests include the processing and characterisation of multifunctional and lightweight structural materials. |
1 Introduction
Bone tissue has a complex, hierarchical, three dimensional (3D) porous structure and performs a range of unique mechanical, biological and chemical functions. Bone grafting material, the gold standard for the treatment of these injuries, has limitations relating to harvesting the tissues, lack of availability, etc. Thus, it is imperative to use synthetic bone grafting materials.1–3 The use of bone grafts and substitutes during orthopaedic surgery is increasing significantly, and the global market was valued at USD 2.98 billion in 2019 and is projected to reach USD 4.15 billion by 2026.4 Bone tissue engineering aims to replace injured bone tissue in order to restore functionality and promote healing. This engineering approach requires osteogenic cells, biochemical growth factors, and a porous, bioactive and biodegradable bone scaffold that is capable of accelerating bone formation and providing mechanical support during bone regeneration.5,6 The mechanical properties of an engineered bone scaffold should match those of the host bone tissue, with appropriate strength to prevent failure and sufficient stiffness to avoid bone tissue resorption.7,8 Bone scaffolds should also have sufficient mechanical strength to withstand the hydrostatic pressures in vivo and to maintain the pore space required for cell and blood vessel in-growth.5,6,9,10 An interconnected, highly porous microarchitecture with suitable porosity (i.e., 75–95 vol%) is a prominent feature in this hierarchy and plays an important role in cancellous bone scaffold design and for functional performance both in vitro and in vivo.11 The degradation rate also must be closely controlled and matched with the growth rate of new bone (e.g. 9 months for spinal fusion scaffold or between 3 and 6 months for craniomaxillofacial applications) to gradually create space for new bone tissue formation and to eventually transfer the load to the new bone.6,12,13 By the time the injury site has regenerated, the scaffold should be completely degraded.14 The interface between the bone substitute material and the biological environment can trigger a wide variety of processes, from an initial inflammatory reaction to the ultimate bone tissue remodelling.15 The considerations for scaffold design are therefore numerous and complex, and furthermore are unique to individual sites of repair in patients.16,17
The application of surface modification treatments like using nanoscale coatings can enhance the performance of the scaffolds and improve the cellular responses for tissue repair.18,19 Nanoscale coatings offer an exciting opportunity for tailoring the surface and bulk property modification of bone tissue engineering scaffolds for specific applications.20,21 The biological properties, mechanical properties and degradation of bone scaffolds can be controlled by the application of nano- and microscale coatings using various deposition methods, such as atomic layer deposition, layer-by-layer (LbL) assembly, plasma spraying, electrophoretic deposition or sol–gel processes.22–25 These coatings can traverse material scales by affecting macroscale properties through structural features that extend to the nanoscale. In particular, the LbL assembly technique shows promise in providing an environment that resembles native bone by mimicking the structures and functions of the native ECM, and promoting cell adhesion, proliferation, and migration.26–28 This technique has been shown to be a very simple, efficient, and versatile technique for surface modification in fabricating tissue engineering scaffolds.29
Numerous studies have reported the use of LbL assembly to incorporate proteins, growth factors, polysaccharides, nucleic acids, and functional peptides into biomaterials, and to deposit coatings with controlled degradation rates.30–34 In addition, the physicochemical properties of multilayer films such as topography, stiffness and strength, and hydrophobicity can be optimized by altering the coating composition and processing parameters, which in turn enables control over biological cellular responses.35,36
The bone tissue engineering field faces numerous challenges including difficulties of in vitro cell culture, mimicking osteogenesis in native tissue, the cost and complexity of manufacturing, and achieving biomaterial scaffolds that satisfy the diverse structural, mechanical and biological properties required for bone tissue engineering applications.37–39 Deposition of multifunctional coatings using LbL assembly is a potential route to addressing these challenges due to the low cost and simplicity of the technique, the fine control over composition and structure, the ability to achieve a tailored hierarchical structure and the potential for the high loading capability of bioactive molecules.29 Furthermore, the fabrication of coatings using LbL assembly is of interest in the development of biocompatible implants for bone tissue repair and replacement applications.40,41 Over the last decade, research studies conducted on the LbL assembly technique applied to bone tissue engineering and bone regeneration have significantly increased by nearly five times in the number of publications, as shown by a keyword search on the ISI Web of Knowledge database of topic terms “layer-by-layer assembly for bone regeneration” that reveals an increase from 100 papers in 2010 to more than 500 papers in 2020.
Herein, a brief overview of LbL-assembled multifunctional films is presented with a focus on coatings with tailored mechanical and bulk properties, coatings on porous 3D templates, biocompatible coatings that promote cell and protein adhesion, biodegradable coatings, and coatings containing bioactive molecules and drug delivery systems. An overview of the LbL assembly fabrication technique is followed by a review of the properties and functionalities of LbL-assembled coatings that are relevant for producing 3D constructs that satisfy the diverse requirements for bone tissue engineering. Finally, a perspective on the broad potential afforded by combinations of LbL-assembled coatings in bone repair and bone tissue engineering is given.
2 Overview of the LbL assembly technique
2.1 Deposition process and mechanism
LbL assembly is a form of template-assisted manufacturing that is used to fabricate thin film coating layers and functionalize surfaces.42,43 The LbL technique is a simple and effective surface modification strategy.44 The technique is based mainly on electrostatic attraction and involves the adsorption of oppositely charged electrolyte complexes onto a substrate, resulting in uniform single layers that can be sequentially deposited to form multilayer films.29 In addition to electrostatic attraction, the creation of multilayer films can also be driven by covalent bonding, van der Waals forces, biological recognition and hydrophobic interactions, or a combination of these forces.45 As illustrated in Fig. 1a, during a typical deposition sequence, a substrate is subjected to a negatively charged electrolyte (e.g. polyanion, or micro-/nanoparticles) and a negatively charged monolayer absorbs onto the substrate surface. The substrate is next immersed in a positively charged electrolyte (e.g. polycation); the charge of the adsorbed cations is sufficient to neutralise the previous layer, invert the surface charge and create a positively charged monolayer on the surface. In order to remove unattached or weakly attached electrolytes and to prevent the intermingling of oppositely charged electrolytes, the substrate is usually rinsed with water or a suitable solvent between deposition steps.46
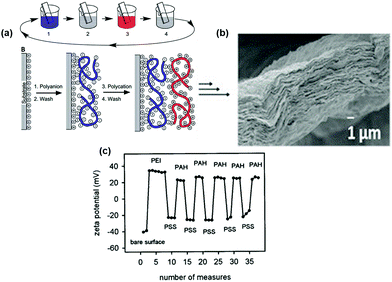 |
| Fig. 1 (a) Schematic of LbL assembly using two oppositely charged polyelectrolytes where step 1 is a polyanion, step 2 and step 4 are water washes, and step 3 is a polycation. (b) Cross-sectional micrograph showing 60 quadlayers of polyethyleneimine (PEI), polyacrylic acid, nanoclay (PEI/PAA/PEI/nanoclay) film fabricated with LbL assembly.47 Reprinted with permission from J. Mater. Chem., 16, 2006. Copyright 2006 Royal Society of Chemistry. (c) Typical change in zeta potential during the build-up of PSS/PAH bilayers using LbL assembly.48 Reprinted with permission from Langmuir, 16, 2000. Copyright 2000 American Chemical Society. | |
As shown in Fig. 1a and b the deposition of electrolytes onto the surface does not result in the formation of 1
:
1 stoichiometric complexes, but rather there is an overcompensation of charge at the surface that allows deposition of further layers via electrostatic interactions.49,50 This process can be repeated until the desired thickness or material coating is obtained. Examples of a multilayer film fabricated in this way are shown in Fig. 1b. The LbL assembly process enables precise control (theoretically down to a single nanoscale layer) over the multilayered structure, composition, and thickness of the resulting films, as a wide range of species can be deposited in a predetermined sequence.21 The potential of LbL assembly to deposit a wide range of functional constituents within multilayer-structured coatings makes the approach of LbL assembly a strong procedure for fabricating engineered tissue scaffolds that can be applied onto various porous template materials to achieve a wide range of properties, pore structures, and multifunctionality.51 The alternating change in surface charge after deposition of each layer is illustrated in Fig. 1c for a substrate coated with polycationic polystyrenesulfonate (PSS) and polyanionic polyallylamine (PAH) bilayers with an initial layer of PEI on a glass substrate.
2.2 Deposition methods
LbL-assembled films can be deposited by dipping a substrate into oppositely charged solutions, spraying solutions onto the substrate, or by spin-assisted LbL assembly, as illustrated in Fig. 2.52–54 There are advantages and disadvantages to all three methods of deposition. The traditional dipping assembly of multilayers is based on cyclic dipping of the substrate in solutions. It is the most studied and well-known and is a convenient and cost-effective technique. However, it can be time-consuming and can require long solution–substrate contact times.40 The spray- and spin-assisted assembly methods were developed to accelerate the deposition process. In spray deposition, a multilayer film is created by spraying solutions perpendicular to the substrates and the removal of the excess solution is driven by gravity. The deposition process is faster, and the resulting films are smoother compared to the dipping method, however, films are often inhomogeneous due to incomplete wetting of the substrate edges. Spin-assisted deposition is also faster than the traditional dipping method, with the deposition of an individual layer taking seconds. This method can also be used with uncharged polymers, but the main limitation is that it is challenging to coat large areas or 3D structures.55,56
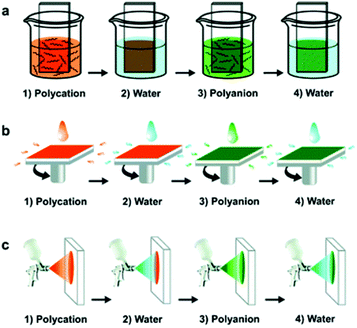 |
| Fig. 2 Schematics of the LbL assembly fabrication process. From top: dipping method, spray-assisted method, and spin-assisted method.57 Reprinted with permission from Chem. Soc. Rev., 41, 2012. Copyright 2012 Royal Society of Chemistry. | |
Deposition of LbL multilayer films as coatings onto open-cell porous substrates for mechanical reinforcement, controlled porosity, or added functionality such as biocompatibility or bioactivity, is a potential route for addressing the design challenges associated with the currently available bone tissue scaffolds.58,59 The strategy is to use this simple, inexpensive and environmentally friendly method to produce a suitable coating for bone scaffolds with relevant characteristics including (a) high microscale thickness, (b) tailored mechanical properties, (c) controllable porosity, and (d) surface modification that allows for the desired physical, and (e) chemical and biological functionality.
The ability to fabricate LbL assembly coatings in a time- and cost-effective manner is a potential obstacle to the clinical translation of the technique. The processing times associated with LbL assembly are likely to be prohibitively slow for producing bone tissue scaffolds or customised porous materials for other applications. However, forced drying of specimens could significantly reduce the processing times to the required deposition time. Furthermore, the implementation of LbL assembly can be easily automated and improved for commercial production.60–62 Considerable work has focused on reducing the LbL assembly processing times by the incorporation of automated systems and faster deposition kinetics. For example, the utilisation of a rotating slide holder reduced the solution contact time up to 10-fold and facilitated the fabrication of coatings with increased thicknesses.63 Electrophoretic deposition used to speed up the deposition of the LbL-assembled PEI/PAA/PEI/nanoclay coatings, resulted in nearly an order of magnitude increase in thickness (compared to coatings fabricated without an electrical current) and achieved controlled coating thicknesses in a much shorter time than the conventional LbL assembly dipping method.21,64
2.3 Constituents and substrates
LbL assembly has been successfully implemented using a wide range of constituents including proteins, polymers, inorganic nanoparticles, clay nanosheets, organic dyes, dendrites, viruses and cells, nanotubes and nanofibers. These constituents can be deposited onto a wide range of substrates to form large area films and coatings on substrates of almost any shape.65 Substrates reported in the literature include glass slides, silicon wafers, paper, quartz crystals, silicone rubber, titanium implants, 3D porous scaffolds, and biological cells.29,46,66 The thickness, roughness, and morphology of LbL-assembled films built from weak polyelectrolytes can be tailored through simple adjustments of assembly parameters such as the pH of solutions, salt concentration, the molecular weight of polyelectrolytes, time of rinsing, dipping times, and temperature.28,67,67,68 Due to this versatility, simplicity and variety of constituents, LbL assembly has been utilised in a wide-ranging number of biomedical applications, such as coating of implantable materials, tissue engineering, biosensors and drug delivery systems.41,52,69–71
2.4 Film growth and thickness
The total thickness of a multilayer film is determined by the number of times a deposition cycle is repeated. Typically, there is a linear relationship between the number of deposition cycles and total thickness. The slope of this linear relationship is the average thickness of the repeating multilayer unit and the film build-up is usually proportional to the dimensions of the constituents deposited.57,68 Linear growth occurs when strong polyelectrolytes are used. Alternatively, some pairs of weak polyelectrolytes, such as poly-L-lysine (PLL)/polyglutamic acid (PGA) or polyacrylic acid (PAA)/PEI, exhibit a more rapid “exponential” growth of thickness. The thickness increase of these exponential films is significantly faster compared to the linearly growing films and is not limited to the dimensions of the incorporated polymers or particles. The thickness and growth rate typically accelerate with the number of layers previously deposited and can reach several microns after fewer than 10 deposition cycles, whereas linearly growing films are typically on the order of several nanometres thick per deposition cycle.41,52,72
In addition to the differences in growth and thickness, linearly and exponentially grown films exhibit different characteristics and properties. Typically, exponentially growing films are softer, have higher water content (up to 80% of volume) and are permeable to water, and large ions and molecules compared to films grown linearly.29 Some exponentially grown polymer–nanoclay films (e.g. films consisting of PEI/PAA/PEI/montmorillonite (MTM) nanoclay) are unusual in that they exhibit a higher stiffness and hardness compared to films grown in a linear manner. This has been attributed to the dynamic structure of exponential films that is enabled by electrolyte mobility and allows polyelectrolytes to adjust and more closely interface with the nanoscale topography of clay sheets.73,74 The high modulus of exponentially grown films without nanoclays (PEI/PAA) has also been attributed to assembly conditions that result in highly cross-linked, interpenetrating polymer networks.44,75
The exponential growth rate, permeability, stability and thickness per multilayer can be controlled and enhanced by altering the ionic strength or the pH of the polymer solutions.76,77 Adding salt to a weak polyelectrolyte results in film swelling, softening and smoothing as the amount of salt added is increased. By changing the pH of weak polyelectrolytes the charge on the functional groups is reduced and as a result, a higher growth rate and thicker films are achieved.73,78 The characteristics of films grown exponentially are contrasted with films grown linearly in Table 1. A key limitation of LbL assembly is that the nano- and microscale thicknesses, together with the slow rate of deposition,21,79 can restrict macroscale applications requiring thicker films.11,73,80 Fabrication of thick films using exponentially grown films can help increase the deposition rate, but typically remains limited to the order of ∼100 µm total thickness in most practical cases.
Table 1 Exponential vs. linear growth
Film properties |
Linear growth |
Exponential growth |
Ref. |
Film thickness |
Mass and thickness increase linearly as the number of deposited bilayers increases. |
Thickness of dried films increased exponentially with the number of deposited layers |
81
|
Polyelectrolytes during deposition |
Usually observed in highly charged polyelectrolytes, or polyelectrolytes dissolved in solutions of low ionic strength |
Caused by “in-and-out” diffusion of particular combinations of polyelectrolytes |
68
|
Permeability |
H2O, small ions, small molecules |
H2O, large ions and large molecules |
52
|
Mechanical properties |
Elastic solids |
Liquids or weak gels |
52
|
Water content |
Low (30% of volume) |
High (up to 80% of volume) |
|
Ions from electrolyte |
Very small |
Usually high |
52
|
Drug delivery |
Limited |
Can be loaded with biological molecules and act as reservoirs to deliver such molecules to cells |
81
|
3 Properties and functions of LbL-assembled films for bone biomaterials
A fundamental factor in the design of an engineered bone scaffold is the choice of suitable materials, which depends on the specific scaffold application and plays a key role in the overall performance of the implant. The design and manufacture of multi-scale scaffold materials that satisfy the biological requirements (biocompatibility, biodegradability and promotion of cell and protein attachment) while combining the required mechanical properties and porosity is a key objective to their successful implementation in bone tissue engineering.6,27,51 A high level of interconnected porosity is required to stimulate bone growth into the scaffold, however, high porosity reduces the mechanical properties. One of the possible novel approaches to this ongoing challenge is to deposit a stiff and strong polymer-nanocomposite coating onto porous template scaffolds using LbL assembly.40,51,82 Deposition of nanocomposite coatings using LbL onto resorbable scaffolds would allow for a scaffold material with tailored biomechanical properties, which will be absorbed by the body over time.53,68,82,83
Deposition of multifunctional thin films as coatings using LbL assembly for mechanical reinforcement, controlled porosity, and added functionality such as bioactivity is a potential route for addressing these complex design requirements. In this section, an overview of the properties and functions of LbL-assembled films are presented based on their architecture and performance with an emphasis on tailoring surface and bulk mechanical properties, adjusting biodegradability, achieving biocompatibility and the delivery of bioactive molecules and therapeutic drugs, and promoting cell and protein adhesion for bone tissue engineering applications.
3.1 Bulk mechanical properties
A successful bone scaffold should maintain mechanical stability under variable loading conditions while transferring sufficient force to newly formed tissue in order to stimulate osteoblasts.11,84,85 In order to achieve this, it is widely accepted that the mechanical properties of a bone scaffold should ideally match that of the host bone tissue.8 Compared with conventionally fabricated composite coatings (e.g., via in situ polymerisation, melt intercalation, sintering, and vacuum evaporation)10,86–88 films prepared by LbL assembly have the advantages of improved mechanical properties owing to the capacity for high loadings of well-dispersed nanofillers, as well as the ability to tailor material structure and composition at the nano- and micro-scales and to easily add multifunctionality.57,73,89 Polymer nanocomposites with a hard, reinforcing phase of carbon nanotubes, carbon nanofibers, synthetic oxide nanoparticles, and natural nanoclays have been produced via LbL assembly. Several studies have investigated the utilisation of negatively charged MTM nanoclay for mechanical reinforcement of polymer films fabricated via LbL assembly.51,73,90
Although a range of LbL-assembled films with good mechanical properties have been reported, the micro-scale thickness of these films is a challenge to macroscale applications requiring bulk materials, such as bone tissue scaffolds. The LbL assembly of conformal coatings deposited onto highly porous 3D templates can overcome this dimensional limitation and has been reported as a strategy for translating the mechanical properties of microscale coatings to the bulk scale.47 Open cell porous foam templates are shown before and after coating with PEI/PAA/PEI/nanoclay quadlayers in Fig. 3a and b. As the thickness of the coatings increased, the bulk compressive modulus and density increased and the porosity of coated foams decreased. The compressive modulus is plotted as a function of density for these coated foams in Fig. 3c (black/white markers), and the predicted properties (dashed lines) were calculated using scaling laws for coated porous materials with varying coating thicknesses (increases along the dashed lines) and coating materials with varying elastic moduli (increases across the dashed lines). As shown in Fig. 3c, the predicted range of achievable moduli for these coated foams overlaps the range for cancellous bone.
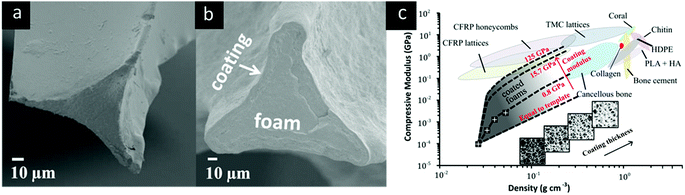 |
| Fig. 3 (a) Cross-section of uncoated polyurethane foam strut and (b) nanocomposite-coated polyurethane foam strut47 (c) Ashby plot of compressive modulus and density, including ranges for common engineering materials, bone scaffold materials, and cancellous bone. Experimental data (markers) and theoretical predictions (dashed lines) for nanocomposite-coated foams are included for coatings with elastic modulus ranging from 0.8 to 125 GPa.47 Inserted images illustrate the change in foam architecture with increasing coating thickness. Reprinted with permission from ACS Appl. Mater. Interfaces, 8, 2016. Copyright 2016 American Chemical Society. | |
3.2 Local surface stiffness
Bone healing is highly sensitive to mechanical loading since mechanical stimulus is a critical regulator of bone structure and function.95,96 Many bone tissue engineering strategies rely upon seeding mesenchymal stem cells (MSCs), which have the potential to differentiate into various cell types.97 MSCs are sensitive to the composition and structure of the materials onto which they are seeded.98 The mechanical properties of 2D surfaces and 3D scaffolds are known to regulate the behaviour of attached cells,99 influence the lineage specification of MSCs, and stimulate osteogenesis of MSCs both in vitro and in vivo.100,101 The stiffness of the microenvironment of pre-calcified collagenous bone tissue is above 30 kPa,102 and matrices with this approximate stiffness have been shown to be osteogenic. For example, Engler et al. demonstrated that MSCs can commit to osteoblasts when cultured on collagen-coated polyacrylamide (PAM) hydrogels with a stiffness of 25–40 kPa.101 Regulating differentiation of bone progenitor cells by mechanical stimuli and committing MSCs to an osteogenic lineage by using scaffold materials with a suitable stiffness in vitro has the potential to reduce the culture time required to pre-differentiate cells and eliminate the need for osteogenic induction supplements.3,103–105 The mechanical properties of the LbL-assembled films can be controlled in several ways: by tailoring assembly conditions (e.g. pH, ionic strength, incorporation of reinforcing phase) or by using covalent crosslinking agents (e.g. glutaraldehyde (GA), genipin).51,106,107 The multilayered coatings are therefore a promising route for the regulation of MSC osteointegration via mechanical cues and studying cell behaviour on substrates with a wide range of mechanical properties achieved by simply varying the assembly conditions or the concentration of the crosslinkers. Examples of how the incorporation of the reinforcing phase results in mechanical reinforcement of multi-layered films and coatings are shown in Table 2. The remaining part of this section will focus on summarising how the cell behaviour can be controlled by modulating the assembly conditions or incorporating crosslinking methods.
Table 2 Examples of mechanical reinforced LbL assembly films
LbL assembly components |
Substrate |
Mechanical properties |
Thickness |
Additional notes |
Ref. |
Polydiallyldimethylammonium chloride (PDDA)/MTM |
Free-standing film |
Strength 109 MPa |
4.9 µm for 200 bilayers |
N/A |
90
|
|
|
Elastic modulus |
|
|
|
|
|
13 GPa (200 bilayers) |
|
|
|
PVA/MTM (GA crosslinked) |
Free-standing film |
GA crosslinked: |
1.5 µm for 300 bilayers |
GA crosslinking improved load transfer between polymer and nanoclay. |
91
|
|
|
Strength 400 MPa |
|
|
|
|
|
Elastic modulus 125 GPa |
|
|
|
|
|
Non-crosslinked: |
|
|
|
|
|
Strength 150 MPa |
|
|
|
|
|
Elastic modulus 13 GPa |
|
|
|
PEI/PAA/PEI/MTM |
Glass slide |
Elastic modulus 15.7 GPa (100 quadlayers) |
100 µm for 100 quadlayers |
Exhibited exponential growth during LbL assembly. |
92
|
PEI/PAA/PEI/MTM covalently cross-linked |
Free-standing film |
Elastic modulus 45 GPa (20 quadlayers), 25 GPa (100 quadlayers) |
920 nm for 10 quadlayers |
Electrophoretic deposition used to enhance deposition rate during LbL assembly. |
64
|
PEI/PAA/PEI/single wall carbon nanotubes (SWNT) |
Free-standing film |
Elastic modulus 15 GPa for 8 quadlayers |
1 µm for 8 quadlayers |
N/A |
93
|
PDDA/MTM |
Silicon wafers |
Elastic modulus 9.5 GPa |
528 nm for 100 bilayers |
N/A |
94
|
PEI/PAA ionically cross-linked |
Porous stainless steel |
Elastic modulus 23.4 GPa for 10 bilayers |
1 µm for 10 bilayers |
High stiffness was attributed to alternating high/low pH of the solutions, which resulted in high ionic cross-linking and inter-diffusion of PEI and PAA. |
75
|
PEI/PAA |
Free-standing film |
Elastic modulus 0.225 GPa for 40 bilayers |
N/A |
N/A |
93
|
PEI/PAA/PEI/nanoclay |
Silicone substrate or free-standing film |
Elastic modulus 0.601 ± 0.518 GPa (flexible substrate), 1.667 ± 1.057 GPa (free-standing) for 60 quadlayers |
16.07 for 60 quadlayers |
N/A |
47
|
3.2.1 Tailoring deposition conditions.
The thickness, roughness, and morphology of LbL-assembled films can be tailored through simple adjustments of assembly parameters such as the pH of solutions, salt concentration, the molecular weight of polyelectrolytes, time of rinsing, frequency of intermittent drying steps, dipping times, and temperature.
Weak polyelectrolytes in an aqueous solution exhibit a pH-dependant average charge density per unit of functional groups along the polymer chain and thus the extent of interaction between charged polymers can be controlled, whereas in the strong polyelectrolytes the charge density is constant. Yang et al. reported the thickness of 30 bilayers of PEI/PAA bilayers to be 4740, 890, 350 or 90 nm depending on the combinations of pH values of solutions (10/4, 8/6, 4/4 and 7/7, respectively for PEI/PAA).108,109 Kim et al. reported that the thickest PAA/PEI films with the highest elastic modulus were assembled when the pH of PAA was 4, and the pH of PEI was 10, after considering pH values of 6, 8 and 10 (Fig. 4). The 10 bilayers of PEI/PAA were 1 μm thick and had an elastic modulus of 23.4 GPa.75
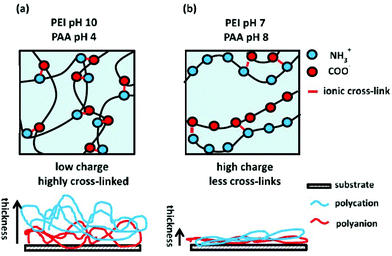 |
| Fig. 4 Schematic representations of the interactions between PEI and PAA at pH that allows for (a) high degree of ionisation and (b) low degree of ionisation in both polyelectrolytes. Below, the film thickness affected by a change in pH is shown.75 Reprinted with permission from Adv. Mater. 2014.26 Copyright 2014 Advanced Materials. | |
The influence of the salt ions on the structural properties of polyelectrolyte multilayers has been reported previously, although there is a discrepancy between the results. The thickness of PAH/PAA films increased with an increase in the concentration of NaCl in an aqueous solution of PAH and PAA.110 In PEI/nanoclay bilayers, increasing salt concentrations (1 mM, 10 mM, 100 mM, 1 M) in the PEI solution with a pH of 8 also resulted in the formation of thicker films, whereas adding salt to unadjusted PEI (pH 10) and clay solution (pH 10.5) did not affect the thickness.109 However, Mjahed et al. observed that when the salt concentration was above 0.48 M, PLL/HA film thickness and mass decreased.111 A similar effect was observed in PAH/PAA films where the thickness decreased with an increased salt concentration.110
3.2.2 Addition of reinforcing layers.
The stiffness of LbL coatings can also be increased through the deposition of a high stiffness reinforcing layer. Biocompatible MTM nanoclay platelets have been successfully incorporated as mechanical reinforcement in PDDA/MTM bilayer coatings and applied onto PAM hydrogels to increase surface roughness and stiffness.112 The compressive modulus of the hydrogel coated with 10 bilayers of PDDA/MTM increased to 190 kPa, resulting in a cell adhesive surface that promoted epithelial cell adhesion and cell migration into the scaffold.91 Various open cell polyurethane foams have been used as models for cancellous bone. Polyurethane foam has a similar morphology to cancellous bone and many bone tissue scaffold like materials. Because of this, polyurethane foam was used as a template to examine the possibilities of tailoring the mechanical properties of highly porous bone tissue scaffolds by the coating LbL process. The LbL assembly of conformal coatings deposited onto porous 3D templates is another example of this strategy using stiff coatings to reinforce softer substrates. Uncoated open-cell polyurethane foam samples with a compressive elastic modulus of 95.33 ± 9.8 kPa increased by up to 1100% to 882 ± 178.1 kPa after deposition of 15 PEI/PAA/PEI/nanoclay multilayers.51,113
3.2.3 Crosslinking.
Crosslinking provides a useful way to modify the mechanical properties of LbL coated substrates. Various chemical (e.g. EDC (1-ethyl-3-(3-dimethylaminopropyl)carbodiimide and genipin) and physical (e.g. photo-crosslinking) crosslinking techniques have been employed to improve the stiffness of LbL-assembled films. EDC is a water-based crosslinker that can be applied after the assembly of polyelectrolytes containing amine and/or carboxyl groups, and leads to the formation of amide cross-linking bonds.106,107 A study by Ren et al. showed that the stiffness of PLL/hyaluronic acid coatings on glass slide substrates was directly proportional to the concentration of EDC used in post-assembly crosslinking. The adhesion of skeletal muscle cells (C2C12) increased as the stiffness increased. Cell differentiation was also found to be dependent on the film stiffness with the stiffest films (>320 kPa) promoting cell proliferation.114 In another study of EDC crosslinking of PLL/hyaluronic acid multilayers, a range of stiffness gradients from 200 to 600 kPa was reported.115 PAA/PAH films have also been crosslinked using EDC, exhibiting stiffness values ranging from 0.5 to 100 MPa.116 In both of these PLL/hyaluronic acid and PAA/PAH material systems, the cells preferentially attached to the stiffer sections of the film and exhibited increased proliferation and cell spreading.117
LbL-assembled PVA/MTM films crosslinked with GA displayed a brick-and-mortar arrangement of organic and inorganic constituents resembling those found in nacre and bone, and exhibited exceptionally high stiffness and strength (tensile modulus of up to 125 GPa and tensile strength up to 480 MPa). Such a highly controlled, layered structure is a unique advantage of the LbL assembly method.90,118 The mechanical properties of the selected films deposited by LbL assembly with and without inorganic reinforcing phases are summarised in Table 2, and demonstrate that several LbL-assembled material systems exhibit mechanical properties comparable to cortical bone (elastic modulus in the range of 7 to 30 GPa (ref. 13) and compressive strength of 100–230 MPa).
Genipin is a natural crosslinking agent derived from the gardenia fruit that has been used to modify the stiffness of bilayers of chondroitin sulfate A (CSA) and PLL deposited onto glass substrates. The resulting crosslinked films had elastic modulus between 170 and 280 kPa, a relevant range for the control of cells, which are capable of detecting small variations in stiffness.119 The LbL assembly films were biocompatible and promoted adhesion, proliferation, and early and late osteogenic differentiation of osteoblast precursor (MC3T3-E1) cells. Because the stiffness and nano-topographical characteristics of the films influence physiological processes in cell behaviour such as adhesion and proliferation. Also, the stiffness and topography of the films were controlled by the amount of the crosslinking agent.83,117,119 The response of local surface stiffness to the cell state has significant implications for cell adhesion and differentiation as well as for tissue repair and regeneration. These results demonstrated the osseointegration of engineered bone substitutes and created multifunctional films capable of finely controlling the morphology and, in turn, influencing the subsequent behaviour of cells.120,121 Overall, the results show that with an increase in substrate stiffness controlled by crosslinking agents, the cells usually exhibit enhanced cell adhesion, enlarged cell spreading with defined actin organization, increased cellular contractility, decreased migration speed, and increased proliferation.117,122,123
In a similar approach, photo-crosslinking of PAA/PAH films was achieved by grafting PAH with a photosensitive benzophenone. LbL-assembled films with a range of uniform stiffness and stiffness gradients were fabricated by varying the time of ultraviolet light exposure and by utilization of gradient density filters which regulated UV light exposure. Films with a stiffness gradient ranging from 55 to 140 MPa were obtained with both steep and shallow gradient slopes. Osteoblast-like cells (U-2 OS) spread and adhered better to the stiffer regions of the films.124
3.3 Biodegradation
Biodegradation of scaffolds for bone tissue engineering applications should be tailored to achieve a gradual transfer of mechanical loads to the newly formed and surrounding bone tissue.13,127,128 In cell-seeded scaffolds, the degradation rate should be adjusted to avoid high levels of scaffold degradation during the initial incubation stage in vitro. The degradation rate of LbL-assembled films can be controlled depending on the type of polyelectrolytes used.27,129 Multi-layered films fabricated with natural polyelectrolytes such as polysaccharides can be degraded by various enzymes like lysozyme and the degradation rate can be tailored via the degree of crosslinking. Schneider et al. demonstrated that in vitro biodegradation of chitosan (CH)/hyaluronic acid and PLL/hyaluronic acid films in contact with hyaluronidase and macrophages can be controlled by altering the concentration of EDC during crosslinking treatments.129 Compared to untreated films, crosslinked films had increased surface roughness, were 10-fold stiffer, and exhibited a slower degradation rate. Garza et al. demonstrated that the degradation rate could also be controlled via the thickness of biodegradable layers. The films were composed of exponentially growing PLL/hyaluronic acid bilayers that acted as reservoir layers and were alternated with biodegradable PLGA barrier layers that were incorporated by spray-casting. Films composed of two PLL/hyaluronan bilayers and one PLGA barrier were rapidly degraded by murine bone marrow cells (within 1 h).130
LbL-assembled coatings can also be used to tailor the degradation rate of the underlying substrate material when deposited onto a biodegradable substrate.61,131 Magnesium is biodegradable, osteoconductive, biocompatible, and has an elastic modulus and compressive strength similar to that of bone and thus is an attractive material for the fabrication of implants and scaffolds for bone repair applications. However, its rapid degradation rate causes the release of high volumes of hydrogen, magnesium and other ions, and the limited bioactivity of magnesium implants in vivo limits their widespread applications.132 Surface modification of magnesium alloys by metal–organic framework-chitosan coatings has recently been presented as a new approach to control the degradation rate of magnesium implants for bone regeneration.133 PLL/alginate multilayer coatings with fibronectin immobilised via EDC crosslinking have been deposited onto magnesium substrates in an effort to increase bioactivity. The coating reduced cytotoxicity in MC3T3-E1 osteoblast cell lines and an in vitro biodegradation study concluded that coated substrates were more resistant to corrosion, however, it did not alter the degradation kinetics of the magnesium alloy substrates.134 LbL-assembled coatings have also been deposited onto AZ91D135 and WE43
136 magnesium alloys for improved corrosion resistance. In both studies, the LbL-assembled coating temporarily reduced the hydrogen evolution of the magnesium substrates and enhanced the corrosion resistance and tailored the degradation rate.
3.4 Electroactive coatings
In addition to mechanical stimuli, the differentiation of MSCs along an osteogenic route can also be achieved with electrical stimulation, which has been reported to determine cell behaviour and help induce bone healing.137 Pulsed electromagnetic fields, capacitive coupling, and direct current have each been used to promote bone regeneration.138,139 Several electroactive components (e.g. metal, carbon nanotube, graphene and conductive polymers) have been utilised in LbL assembly to fabricate functional coatings that can deliver such electrical stimuli.140 Multilayer materials produced by the LbL assembly of PEI and the conductive polymer polyammonium-3-thienyl-ethoxypropanesulfonate (STP) have been reported to improve osteoblast adhesion and proliferation and promote osteogenesis.141 The conducting polymeric system was electroactive, fully erodible, and suitable for adhesion and growth of cells derived from mouse fibroblast (L-929) and skeletal muscle (C2C12).142 Electroactive tetraaniline and degradable multilayered films consisting of PGA-graft-tetraaniline and PLL-graft-tetraaniline have been reported to support osteoblast function and promote osteogenic differentiation. Using this material system, the electrical stimulation of mouse-derived pre-osteoblast MC3T3-E1 cells in vitro resulted in increased expression of osteopontin and runt-related transcription factor 2 (RUNX2), which are markers of osteogenesis.143,144
3.5 Cell interactions and protein adhesion
The surface properties of implants influence tissue and cellular events, including protein adsorption and cell behaviour, both of which are important to bone tissue remodelling.145,146 For this reason, considerable efforts have been devoted towards the surface modification of bone scaffolds for added functionality and the creation of a suitable environment for cell growth and tissue formation.38,147 LbL-assembled multilayer coatings are a promising tool for controlling the attachment behaviour of cells onto surfaces because of the importance of surface properties in cell function.130 Recently, significant efforts have been devoted to optimizing the surface properties of LbL assembly films, such as surface bioactivity, roughness, hydrophilicity, etc.148 In addition, polyelectrolyte multilayer films have been shown to be a powerful tool for the immobilization of biomolecules with preserved bioactivity.
The electrostatic charge of the final layer at the surface of a multilayer film can have a dominant effect on cell attachment; cells may prefer positively or negatively charged terminating layers depending on the cell line.149 Because the ability of the LbL deposition technique in 3D mimetic architectures will be an original means of controlling supra-cellular organization, the reciprocal interactions between active cells and active polyelectrolyte multilayer surfaces offer enormous potential to control cell behaviour.38 Tryoen-Toth et al. observed higher viability and adhesion of SaOS-2 osteoblast-like cells when PEI-(PSS/PAH) coatings on glass slides were terminated with PAH, PGA and PLL layers, whereas the number of attached cells decreased on PSS and PEI terminating layers. A significant downregulation of alkaline phosphatase (ALP, a phenotypic marker for the early stage biomineralisation) was observed in SaOS-2 cells seeded on PEI-terminated layers after 24 h of contact, whereas only a small downregulation was measured for SaOS-2 cells in contact with negatively charged PSS- or PGA-terminated films. Osteocalcin expression (a marker for the mature osteoblast phenotype) in SaOS-2 cells at 24 h was not influenced by any of the polyelectrolyte films. This study concluded that the electrostatic charge of the outer layer of the LbL-assembled films played a major role in early osteoblast phenotype regulation. The LbL-assembled films terminated with PSS, PGA and PLL layers showed good biocompatibility towards SaOS-2 cells and were deemed as biologically inert for bone tissue engineering applications.150
The several concepts for adjusting the chemical, physical, and mechanical properties of PEM films have fostered studies on the influence of these factors on cellular interactions. Adhesion of cells to the scaffold material is an important prerequisite for the successful colonisation of engineered bone substitutes.38,151 To improve the adhesion of pre-osteoblast MC3T3-E1 cells onto nanofibre poly-L-lactic acid (PLLA) scaffolds Liu et al. coated the scaffold surface with gelatin and poly(diallyldimethylammonium chloride) (PDAC). The amount of gelatin was controlled via the number of layers deposited, and the wettability of the scaffold was controlled by changing the final layer. The number of MC3T3-E1 cells on coated scaffolds was significantly higher compared to uncoated scaffolds at 4 h and 24 h time points after cell seeding, and the number of cells increased as the number of PDAC/gelatin bilayers increased. Furthermore, the cells proliferated at a higher rate and were more evenly distributed on coated scaffolds compared to the uncoated scaffolds.152
Recent research has significantly broadened the understanding of the effects of nanocoatings in controlling cell behaviour because the 3D nanostructured surfaces can directly affect intracellular signalling pathways.83,89,153 The LbL assembly of layers or sheets of cells may be utilized to fabricate scaffolds with cell colonization and proliferation extending throughout the scaffold. LbL cell sheets developed for bone regeneration based on interactions between hydroxyapatite (HA) microparticles and pre-osteoblast MC3T3-E1 cells were fabricated by Hu et al. The LbL-assembled film mimicked the natural combination of the inorganic/organic bone matrix. The morphology of the cells in deeper layers was comparable to that of the top layer. The pre-osteoblast cells within the film maintained high osteogenic capacity.154 Therefore, the LbL assembly could be a suitable tool to incorporate cells directly into the porous scaffolds for homogeneous cell distribution and colonisation inside the bone scaffold.
Having robust protein adhesion and absorption into the surface of biomaterial plays a crucial role in stronger cell adhesion for tissue regeneration. Protein adhesion is necessary for the formation of a bio-interface between implanted materials and native bone.155,156 Adsorption of proteins onto LbL-assembled films has been studied widely, and a kinetic model of protein adsorption has been described.157,158 PLL/DNA bilayers have been used to enhance protein adsorption and biomimetic mineralisation on the surface of titanium implants for osteoblast cell culture experiments. PLL is a positively charged polyelectrolyte that is biocompatible and can be easily conjugated with bioactive molecules. DNA has also been used for LbL assembly with layers of PEI, PDDA and PAH.159–161 The high-phosphate content in DNA was found to be beneficial for the deposition of calcium during bone formation due to the high affinity for calcium ions. The LbL assembly of PLL and DNA has also been suggested as a way to improve the bioactivity of titanium for bone tissue repair applications. In vitro investigation has confirmed that osteoblasts isolated from neonatal rat calvarias adhered to coated titanium surfaces and resulted in improved cell/cell and cell/substrate interactions compared to uncoated titanium control samples. Surfaces coated with PLL/DNA/PLL showed a higher number of overlapping and interconnected osteoblasts. The surface roughness of PLL/DNA coatings depended on the terminating layer and was higher with PLL as the last layer. Cell viability, assessed with the Alamar blue assay, demonstrated that tailored surface roughness improved the biocompatibility of titanium implants and cell adhesion.156
To attract osteoblasts, titanium implants have been coated with calcium phosphate (CaP)-containing films. CaPs are bioactive and possess well-known osteoinductive properties that stimulate bone formation.162 Incorporation of biocompatible and antibacterial chitosan (CH) into the multifunctional LbL-assembled film prevented peri-implant infection, and HA particles induced bone mineralisation. The amount of mineralisation increased with increasing numbers of CH/HA bilayers. The coatings were biocompatible with pre-osteoblast MC3T3 cells and effective against infection by Streptococcus gordonii.163
3.6 Drug and growth factor delivery
The functionality of bone scaffold materials can be enhanced by loading biomolecules (i.e., growth factors and/or drugs) to treat bone disorders or promote bone regeneration by stimulating cell adhesion, proliferation and differentiation.164–166 Incorporated drugs should act at the desired location, at an adequate concentration and for a specific period of time.167 Additional surface modification using the LbL approach allowed the fabrication of effective drug delivery carriers with improved targeting effects. LbL-assembled films can help achieve these requirements by providing spatial confinement of the drug, localised delivery to the tissue, and protection from exposure to physiological media.26,29,61
Controlling the number of growth factors delivered to healing sites can help to maximise their efficacy and avoid side effects such as osteolysis, immunological reaction, and tumorigenesis (all of which are associated with the supraphysiological dosage of bone morphogenetic proteins (BMPs)).168,169 Growth factors can be deposited as layers within a multilayer film using electrostatic LbL assembly, or they can be loaded after LbL assembly in crosslinked films that serve as reservoirs for the proteins.170,171 Poly-β-aminoester 2 and PAA coatings have been utilised as delivery systems with tunable control over the delivery of growth factors including vascular endothelial growth factor (VEGF) and BMP-2. BMP-2, an osteoinductive agent, has been widely used to enhance the bioactivity of engineered bone scaffolds or implants and is known to stimulate the proliferation, migration and differentiation of MSCs. In a study by Shah et al., LbL-assembled coatings of poly-β-aminoester 2-PAA/BMP-2/PAA and poly-β-aminoester 2-PAA/VEGF/PAA were deposited onto 3D osteoconductive scaffolds of macroporous PCL/β-tricalcium phosphate (TCP). The delivery of the two growth factors was triggered by a change in pH from 5.0 to 7.4, causing destabilisation and charge imbalance in the coating, and from hydrolytic degradation of the poly-β-aminoester 2. BMP-2 eluted from the coating over 2 weeks, whereas VEGF was released within the first 8 days. Both growth factors retained their efficacy and induced ectopic bone formation in the intramuscular region of a rat femur.168 Different studies have delivered BMP-2 through the use of various alternative LbL-assembled material systems, including employing a chemically crosslinked PLL/hyaluronic acid film deposited onto TCP/HA porous ceramics to deliver rhBMP (recombinant BMP-2);172 poly-β-aminoester 2/BMP-2 deposited onto 3D printed β-TCP/PCL;173 and graphene oxide deposited onto titanium implants, which were evaluated in vivo in a mouse calvarial defect model.174 Deposition of BMP-containing LbL-assembled films onto a substrate allows for a local administration and controlled delivery;169 and has the potential to mitigate the main limitation associated with the current method for clinical delivery of BMP-2 through collagen sponge, i.e. rapid release without spatial control.172,175
The application of the LbL assembly technique to deposit antibiotic coatings onto orthopaedic implants has the potential to reduce implant-related infections and accelerate osteogenesis.176,177 The main advantages of using such a technological approach are its relatively simple procedure and requirement of no special equipment when compared to other coating methods.41,178 Recent studies have focussed on the application of LbL assembly to coat titanium implants in an effort to prevent implant-related infection and increase the bone cell interactions at the implant surface. Multilayered hyaluronic acid–dopamine/CH deposited onto the surface of titanium alloy implants (Ti–Nb–Zr) have shown improved anti-infection properties, as well as accelerated osteoblast cell adhesion and differentiation when compared to non-coated titanium implants.179 Tannic acid-alendronate (TA-ALN) nano-complexes have also been applied using the LbL assembly technique in order to augment the antioxidant, anti-inflammatory and osteogenic potentials. In this study also the final result showed that the TA-ALNs have great potential for anti-inflammation, and osteogenic acceleration and can be used to bone repair and regeneration in bone defects. Therefore, using LbL assembly or self-assembly could have significant potential in controlling antioxidant, anti-microbial, and osteogenic potency.180
LbL-assembled films of poly-β-amino ester/PEI/gentamicin sulfate were deposited onto titanium implants to treat an existing infection in a rabbit bone model. The coating released 70% of the gentamicin in vitro during the initial three days through diffusion and film erosion because of the hydrolytic degradation of the poly-β-amino ester when placed in an aqueous environment, and continued to release the gentamicin over the next four weeks. The degradation products were not cytotoxic towards pre-osteoblast MC3T3-E1 cells. In vivo, the coated 3D titanium implants significantly decreased the viable bacterial count compared to the uncoated implant in a simulated one-stage re-implantation model to treat implant-related Staphylococcus aureus infection in rabbits.181
Systems for delivering both antibiotics and growth factors were combined by coating the surface of silicon substrates with a poly-β-aminoester 1 and 2, PAA loaded with gentamicin, bone growth factor BMP-2 with PDDA/chitosan and clay barrier layers for compartmentalised hybrid coatings with controlled release. The multidrug coating aimed to accelerate the bone healing process while preventing implant failure due to post-operative infection. The clay barrier blocked interlayer diffusion, leading to a 10-fold increase in the release rate of the growth factor and enabling customized release behaviour. These tunable LbL assembly coatings were implemented on planar silicon, but can potentially be translated into a variety of implants with complex geometries, including scaffold materials.182
Another example of a dual system for the delivery of a cell adhesion peptide and growth factors was reported by Wang et al. Negatively charged oxidised alginate (OAlg) was deposited with positively charged chitosan (CH) and bovine-serum albumin (BSA)-based nanoparticles were loaded with BMP-2, resulting in positively charged BSA-BMP-2 nanoparticles. These nanoparticles were incorporated into films via LbL assembly together with negatively charged peptide layers. The cell adhesion and proliferation properties of peptide-glycine-arginine-glycine-aspartate-serine (GRGDS) grafted OAlg/CH films were also explored. When deposited onto porous titanium scaffolds, GRGD and BMP-2 provided a favourable microenvironment for bone tissue ingrowth. The resulting coatings achieved sustained release of BMP-2 over 28 days. This long release period was attributed to bovine-serum albumin, which impeded the diffusion of BMPs. Both the biomolecules, BMP and GRGDS, promoted MSC attachment, proliferation and differentiation, and enhanced bone tissue formation.28
Mertz et al. fabricated nanoscale barriers by alternately depositing linearly growing PDDA/PSS and exponentially growing PLL/hyaluronic acid. The barriers acted as mechanically active nanovalves: when a mechanical stimulus was applied, nanopores in the exponentially growing film opened and allowed the diffusion of PLL through the barrier. The opening and closing of the pores were reversible and could be controlled by the magnitude of mechanical deformation.81 Drug release can also be stimulated by pH and salt concentration. For example, PAA/PAH bilayers assembled at a pH of 2.5 have been loaded with methylene blue dye (as a model drug) within the permeable structure of the PAA. The dye was anchored to the PAA by interactions with the carboxylate groups, which were disrupted by a lower pH (2.5) in the environment, thereby triggering the release of the dye.183
The addition of exosomes and osteogenic growth factors to LbL-assembled films has also been investigated for bone tissue regeneration applications. In a recent research study, Cheng et al. developed a core–shell silk fibroin (SF)/polycaprolactone (PCL)/polyvinyl alcohol (PVA) nanofibrous film using co-axial electrospinning and LbL assembly as part of a dual growth factor-release system. BMP2 was incorporated within the nanofibers and the connective tissue growth factor (CTGF) was attached to the surface by a LbL-assembled film. The combination of electrospinning and LbL approaches provided an effective platform for BMP-2 and CTGF delivery, and in vitro and in vivo studies showed improved angiogenesis and osteogenesis.170 The LbL-assembled film fabricated from polycationic poly L-lysine (PLL) and glycosaminoglycan hyaluronic acid (HA) has also been shown to mimic the interactions between exosomes, cells, and the ECM, which dramatically increased the affinity to cells from different lineages.184 Therefore, according to the recent studies, the incorporation of functional exosomes and osteogenic growth factors into LbL-assembled films is a promising approach to better mimic the host structure, improve the delivery of growth factors and augment biological functions.185
3.7 Hierarchical natural structure in micro and nanoscale environments
Hierarchical nano- and micro-scale architecture and arrangement play important roles in cell performance and it has been postulated that bone scaffolds should be designed to mimic the hierarchical structure of the targeted bone.187 Scaffold architecture plays a vital role in guiding cell interactions via direct contact. An interconnected porous architecture is a crucial requirement for a functional bone tissue scaffold to promote vascularization, enable cell migration and facilitate the supply of nutrients and removal of waste products.188–190 The use of the LbL assembly technique to apply coatings on porous foam substrates has been successfully demonstrated. The LbL assembly of nanocomposite-coated foams shown in Fig. 4 resulted in materials with porosity ranging from 98.8% for uncoated foams to 96.6% for foams with the thickest coatings applied.47,51 In a similar approach, the LbL assembly of coatings onto colloidal crystals and subsequent removal of the colloidal crystal has been utilised to produce inverted colloidal crystal (ICC) multilayer materials. The LbL assembly of ICCs has been used to fabricate porous cellular environments targeting various tissues other than bone (i.e. connective tissue,191 cartilage,192 bone marrow193).
It is well proven that microscale structures ensure the primary attachment in the bio-interface of the scaffolds and promote cell interaction while nanoscale organization has more significant effects on the adhesion and differentiation of cells.194–196 Bone consists of a mineral phase in the form of apatite nanocrystals embedded in a collagen matrix.197 The nanometre-sized HA crystals play a key role in the high mechanical properties exhibited by bone.198 Nanostructured surfaces have widely been reported to promote osteoblast adhesion and cell growth has been enhanced by the introduction of a randomly distributed nano-topographic surface at a scale of approximately 10 nm.199,200 Cell behaviour can also be influenced by nano- and microscale interactions with ECM components since stem cells are sensitive to the topographical features of the ECM.201,202 The ECM spans several length scales, is composed of fibronectin, collagen and laminin, acts as a storage of growth factors and cytokines, provides complex biochemical and physical signals, and induces cell–cell and cell–matrix interactions.186,203 Hence new strategies in bone tissue engineering include bioinspired 3D printed bone scaffolds with nano- and microscale features mimicking ECM structures.204 Nanostructured bone tissue scaffolds fabricated with nanoparticles, nanofibers, and hydrogels aim to mimic the ECM and provide structural support similar to that of the bone tissue architecture. However, combining current bone scaffold fabrication techniques with nanomaterials is challenging.203,205 Due to their large specific surface area, HA nanocrystals tend to agglomerate in polymer matrices, which differs from the homogeneous dispersion of HA in bone.198,206 LbL assembly is capable of producing multilayer structures with a broad range of potential compositions and functional biomolecules with a finely tuned dosage.186 Fundamental studies have shown that LbL assembly can be successfully implemented using ECM components, including proteins, polysaccharides, growth factors, and cells,91,109,186 and the bioactivity of these components is preserved by the mild deposition conditions.
The LbL assembly of ECM-like biomolecules including hyaluronic acid and collagen has been reported as a strategy for mimicking the organic components of natural bone in synthetic polymer scaffolds of PLLA and PLGA, which are commonly used in the regeneration of bone tissue due to their biocompatibility, biodegradability and suitable mechanical properties, but lack bioactivity and appropriate physiological activity.207,208 After the LbL assembly of ECM-mimicking collagen I coatings on PLLA substrates, the in vitro viability and proliferation of osteoblasts and ALP expression were dramatically improved, and cell extension was directed by contact guidance of the aligned collagen I fibrils.208 Collagen type I and HA nanoparticles have also been deposited onto microstructured PCL scaffolds fabricated by direct polymer melt deposition. The composition and density of the HA nanoparticles and collagen in the multilayer coating were tailored by changing the number of layers deposited by a continuous flow of solutions controlled with a peristaltic pump. Osteogenic differentiation was monitored for human MSCs seeded on these coated PCL scaffolds, and an increase in the activity of ALP confirmed the beneficial effect of the coating. Osteogenic differentiation was also confirmed through the analysis of osteoblast-specific bone sialo protein-II, BMP-2, osteopontin and osteocalcin expression.209
3.8 Combination multilayers for multifunctional coatings
The development of multifunctional bone scaffolds that combine improved mechanical properties with enhanced biofunctionality is a key objective of bone tissue engineering.167,210Tables 1–8 present numerous examples of multilayered coatings that exhibit properties and functions relevant to bone tissue engineering and provide an indication of the large number of multilayers that could potentially be combined into a single LbL-assembled coating in order to obtain scaffold materials with very diverse compositions and capabilities.
Table 3 Examples of crosslinked LbL-assembled films with controlled local stiffness
LbL assembly components |
Substrate |
Treatment |
Functionality |
Cell type |
Ref. |
DAR/PAA |
Silicon wafer |
Crosslinked with UV irradiation using a photo-mask |
Tailored surface biological properties and selective absorption of PS nanoparticles |
N/A |
125
|
PAA/PAM conjugated with 4-azidoaniline |
Glass slide |
Crosslinked with UV irradiation using a photo-mask |
Micropatterning and spatial of LbL multilayers film control over cell adhesion |
L929 |
126
|
|
|
|
|
MG63 |
|
PAA/PAH grafted with benzophenone |
Silicon wafer |
Crosslinked with UV irradiation |
Biocompatible surface with uniform stiffness gradient directing vascular and osteoblast cell behaviour |
A7r5 |
124
|
|
Glass slide |
|
|
U-2 OS |
|
PLL/hyaluronan |
Glass slide |
Crosslinked with EDC |
Tunable stiffness of LbL assembly films. Cellular adhesion without adhesive protein coatings |
C2C12 |
114
|
PLL/hyaluronan |
Silicon wafer |
Crosslinked with EDC, fluorescent PLL and RGD conjugated to PGA |
Cells adhere and spread along the stiffness gradient (200–600 kPa) |
C2C12 |
115
|
|
Glass slide |
|
|
MC3T3-E1 |
|
PAA/PAH |
Glass slides |
Crosslinked with EDC |
Controlled with time-dependent immersion in a solution of EDC, gradients of surface elastic moduli (0.5–110 MPa in the hydrated state) |
Human dermal fibroblasts |
116
|
CSA/PLL |
Glass slides |
Crosslinked with genipin |
Stiffness (170–280 kPa) and topography of the coating controlled by changing the concentration of genipin; stiffened surface films promoted cell adhesion, proliferation, and early and late osteogenic differentiation |
MC3T3-E1 |
119
|
PDDA/MTM |
3D PAM hydrogel scaffold |
N/A |
LbL assembly film modified the surface of the hydrogel, increased stiffness, and enhanced cell adhesion |
CRL-7163 |
112
|
|
|
|
|
CCL-240 |
|
Table 4 Examples of biodegradable LbL assembled films
LbL assembly components |
Substrate |
Treatment |
Functionality |
Cell type |
Ref. |
CH/hyaluronic acid and PLL/hyaluronic acid |
Glass slides |
Crosslinked with EDC and N-hydrosulfosuccinimide (NHS) |
Controlled the degradation by altering the concentration of EDC during treatments, crosslinking increased stiffness and surface roughness of biodegradable and bioactive films |
HT29 |
129
|
PLL/hyaluronic acid (reservoirs) PLGA (barrier) |
Silica slides |
PLGA solution sprayed over the hydrated bilayers PLL/HA film for controlling the biodegradation |
Multicompartment films degraded by seeded bone marrow cells |
Murine bone marrow cells |
130
|
PLL/alginate |
AZ31 substrates (one of the commercial Mg alloy systems) |
EDC crosslinking fibronectin immobilized |
LbL assembly films improved the bioactivity of the scaffold; in vitro degradation of layers did not affect the degradation kinetics of the substrates |
MC3T3-E1 |
134
|
Table 5 Examples of electroactive LbL-assembled films
LbL assembly components |
Substrate |
Treatment |
Functionality |
Cell type |
Ref. |
STP/PEI |
Glass slides |
Low voltage electrical stimulus (ES) applied to electroactive films |
Erodible, electroactive LbL material system that promoted cell adhesion and growth |
L-929 |
142
|
|
|
|
|
C2C12 |
|
PGA-tetraaniline/PLL-tetraaniline |
Glass quartz or silicon slides |
Low voltage ES applied to electroactive films |
Increase the level of biocompatibility because of using PLL and PGA, electroactive and degradable film enhanced the commitment of pre-osteoblast cells to osteogenic lineage |
MC3T3-E1 |
143
|
Table 6 Examples of LbL-assembled films promoting cell or protein adhesion
LbL assembly components |
Substrate |
Treatment |
Functionality |
Cell type |
Ref. |
PEI-(PSS/PAH) and terminating layers of PGA, (PGA/PLL), PSS, or (PSS/PEI) |
Glass slides |
N/A |
Good biocompatibility of PSS-, PGA-, and PLL-terminated films for SaOS-2 cells |
SaOS-2, PDL |
150
|
Gelatin/PDAC |
PLLA nanofibre scaffold |
LbL-assembled coating was crosslinked with EDC and NHS |
The amount of gelatin was controlled by number of bilayers deposited; coating improved hydrophilicity; coating improved proliferation and homogeneous distribution of osteoprogenitor cells |
MC3T3-E1 |
152
|
PLL/DNA |
Titanium films |
Both layers of PLL and DNA deposited |
Bioactive coating enhanced proliferation of osteoblasts |
Osteoblasts |
156
|
CH/HA |
Titanium discs |
N/A |
Coatings increased biomineralisation, exhibited biocompatibility with pre-osteoblast cells, and significant anti-bacterial activity against infection by Streptococcus gordonii |
MC3T3-E1 |
163
|
Table 7 Examples of LbL-assembled films for drug delivery
LbL assembly components |
Substrate |
Treatment |
Functionality |
Cell type |
Ref. |
PDDA/PSS (barrier) |
Silicone sheet |
Fluorescein isothiocyanate labelled PLL and rhodamine Red™-X, succinimidyl ester used to monitor diffusion |
Barriers responded to mechanical stimuli; diffusion of PLL through the barriers can be switched on/off by tuning the mechanical stretching |
N/A |
81
|
PLL/hyaluronic acid (reservoir) |
|
|
|
|
|
PAA/PAH |
Glass and silicon slides |
Assembled films loaded with methylene blue dye |
The release of methylene blue dye was pH-sensitive (lower pH increased the release rate). Release was controlled by the incorporation of capping layers |
N/A |
183
|
Poly-β-amino ester/PEI/gentamicin sulfate |
3D titanium Implant |
Solutions prepared in sodium acetate buffer for drug (gentamicin) release experiments |
Coated titanium implant locally delivered hydrophilic antibiotics (gentamicin sulfate) for treatment of bone infection in vivo |
MC3T3-E1 |
181
|
Poly-β-aminoester 2-PAA |
Macroporous PCL/β-TCP cylindrical scaffold |
Solutions prepared in sodium acetate buffer |
BMP-2 eluted from the coating for 2 weeks; release of VEGF was released in the first 8 days; both growth factors retained efficacy |
MC3T3-E1 |
168
|
BMP-2-PAA |
|
|
|
|
|
Poly-β-aminoester 2-PAA |
|
|
|
|
|
rhVEGF165-2-PAA |
|
|
|
|
|
PLL/hyaluronic acid |
TCP/HA porous scaffolds |
Assembled films were crosslinked with EDC and NHS; rhBMP-2 was loaded in crosslinked films |
Coating acted as a delivery reservoir of rhBMP-2; could be stored and maintained bioactivity for 3 weeks; degree of crosslinking influenced the loading of BMP-2 |
C2C12 |
172
|
Poly-β-aminoester 2/chondroitin sulfate |
PCL-β-TCP scaffolds |
Terminating bilayer of BMP-2/chondroitin sulfate |
Coatings facilitated the microgram-scale release of active BMP-2; 80% released during the initial 2 days and the remaining 20% released over the following 2 weeks |
MC3T3-E1 |
173
|
Graphene oxide (GO)-NH3+/GO-COO− |
Planar titanium |
Assembled films were crosslinked with NHS, loaded with BMP-2, and freeze-dried |
Coating increased adsorption and sustained release of BMP-2 and improved in vitro osteogenic differentiation; in vivo more robust bone formation observed in coated implants |
MSCs |
174
|
Poly-β-amino esters 1/PAA/gentamicin sulfate |
Planar silicon wafer |
Barrier layers of CH/PDDA introduced between drug and protein-containing quadlayers |
Coating acted as a delivery system of antibiotics and growth factors; clay barrier slowed diffusion-based release |
MC3T3-E1 |
182
|
Poly-β-amino esters 2/PAA/BMP-2 |
|
|
|
|
|
CH/OAlg /GRGDS |
Titanium discs and porous titanium scaffolds |
Substrates treated with polydopamine |
Sustained release of BMP-2 over 28 days; ECM-mimicking coatings with BMP-2 promoted MSC functions and new bone formation |
MSCs |
186
|
CH/OAlg/BSA-BMP-2/OAlg |
|
|
|
|
|
Hyaluronic acid–dopamine/CH |
Ti–Nb–Zr alloys |
Dopamine-modified HA (DHA)/CH multilayers were deposited on the surface of Ti alloys |
Coating improved anti-infection properties and accelerated osteoblast cell adhesion and differentiation |
MC3T3-E1 |
179
|
BMP2/CTGF |
SF/PCL/PVA nanofibrous film |
Separation of the labelled proteins in the nanofibers performed to monitor the distribution |
Sustained release of BMP2/CTGF promoted MSCs functions for angiogenesis and osteogenesis |
MSCs |
170
|
Table 8 Examples of LbL-assembled films with hierarchical structures
LbL assembly components |
Substrate |
Treatment |
Functionality |
Cell type |
Ref. |
Collagen I/hyaluronic acid |
PLLA discs |
Substrate surface was modified by covalently bonded PEI |
Collagen improved the biocompatibility of substrates (i.e. cell viability, cell proliferation and ALP expression. Osteoblast extensions were directed by contact guidance of the aligned collagen fibrils |
Osteoblasts |
208
|
HA/collagen |
PCL microfibrous scaffold |
HA was modified with dopamine-conjugated hyaluronic acid |
LbL-assembled films showed enhanced osteogenic activities |
MSCs |
209
|
In an example of combining numerous multilayers to produce a multifunctional coating, Shah et al.211 reported a coating system consisting of two different multilayer materials systems that were deposited onto titanium or PEEK bone implants. The first multilayer system consisted of chitosan/hydroxyapatite complexes (CH–HA) and PAA bilayers. These CH–HA/PAA layers were osteoconductive and had an average stiffness of ∼11 GPa (within the typical range for cortical bone13), which mitigated the mechanical mismatch between implants and native bone and provided a permanent bone–implant interface. The CH–HA/PAA multilayers were combined with degradable layers of poly-β-amino ester 2/PAA/BMP-2/PAA containing the osteoinductive growth factor BMP-2. These quadlayers containing BMP-2 were deposited on top of the CH–HA/PAA layers, and enabled gradual and tunable release of therapeutic levels of growth factor without sudden burst-release. The loading of BMP-2 was controlled by the number of quadlayers deposited. The LbL-assembled coating resulted in a long-term stable fixation of the implant in vivo with rodent host tissue, indicating that the mechanical properties of the initial CH–HA/PAA base layers can be usefully combined with the biofunctionalities of the top layers in LbL-assembled coatings.
Mechanically reinforcing and biocompatible layers have also been combined to produce coating systems consisting of mechanically reinforcing PEI/PAA/PEI/nanoclay quadlayers capped with a final layer of thermally cross-linked PAA.51 These coatings were applied onto open-cell foam templates, resulting in coated scaffold materials with a wide range of tailorable porosity (72.3%–95.2%) within the suitable range for bone scaffolds212,213 and with a range of stiffness values (0.604–16.64 MPa) approaching the lower range of cancellous bone. Despite the presence of cytotoxic PEI within the coating structure, no cytotoxic response was observed in MSCs exposed to coated scaffolds with the cross-linked PAA final layers.
LbL-assembled coatings can also be combined with materials derived from other fabrication routes to produce novel and complex material systems. Romanelli et al. utilised organic nanofibers (fluorenylmethyloxycarbonyl protected valyl-cetylamide) as substrates that were coated with collagen type I, an HA binding peptide, and BMP-4 using LbL assembly.214 These multilayered coatings were then incubated with HA nanocrystals and doped with TiO2 nanoparticles. The coated nanofibers were embedded into an alginate hydrogel that is biodegradable under physiological conditions. The coated 3D scaffolds were biocompatible, enabled adhesion of pre-osteoblast MC3T3-E1 cells, and promoted osteogenic differentiation as indicated by ALP assay. Furthermore, the coated 3D scaffolds were biodegradable and demonstrated inherent antibacterial activity.
The LbL concept can also be integrated with other modern additive manufacturing technologies, e.g. 3D printing. Combining the LbL assembly technique with 3D printing platforms offers many advantages for the fabrication of functional LbL nanocomposites targeted towards regenerative medicine and pharmaceutical applications.215,216 Guduric et al.217 fabricated PLA/chloroform solution as printing inks to create PLA membranes using extrusion-based 3D printing with human bone marrow MSCs and endothelial progenitor cells by the LbL bioassembly of cellularised sheets. Assessment of MSC viability, phenotype, and proliferation showed osteoblastic differentiation after 7 days in culture media, which indicated the potential of the LbL assembly approach for the promotion of homogeneous cell distribution within the scaffold. In another study by Chen et al.58 fabricated hydroxyapatite–gelatin scaffolds using extrusion-based 3D printing for bone tissue engineering applications. Thereafter, LbL-assembled coatings of chitosan and sodium hyaluronate were deposited onto the scaffolds. The application of the LbL coating to the hydroxyapatite–gelatin scaffolds reduced the swelling ratio, increased the compressive strength and slowed down the degradation rate. In addition, there was no significant cytotoxicity effect on MC-3T3E1 cells and the scaffolds provided appropriate conditions for cell adhesion and proliferation, indicating that combining 3D printing with LbL assembly technologies offers great potential in bone tissue engineering as a sustained delivery system.
A plethora of potential combination multilayer coatings, which may include the material systems listed in Table 9 or others, remain unexplored and provide rich opportunities for multifunctional material systems for bone scaffolds and wider biomedical applications. In addition to the wide range of constituents that may be utilised in LbL assembly, the thickness, roughness, and morphology of coatings may be tailored through adjustment of assembly parameters (e.g. the number of depositions, pH values of solutions and salt concentrations, intermittent drying steps, and dipping times).67,81,218 Tuning the physical and mechanical properties of the porous structures by depositing suitable LbL-assembled coatings and tailoring the assembly conditions could lead to an appropriate balance of sufficiently high mechanical properties and high levels of porosity required for engineered bone tissue applications, whereas the biological requirements could be satisfied by the incorporation of biomolecules and surface modifications.51,215
Table 9 Examples of multifunctional LbL assembled films
LbL assembly components |
Substrate |
Treatment |
Functionality |
Cell type |
Ref |
MSCs/endothelial progenitor cells (EPCs) |
3D printed PLA membranes |
3D constructs were prepared by assembling four PLA membranes seeded with MSC alone or co-culture of MSCs and EPCs |
Coatings promoted a homogeneous distribution of cells throughout the scaffold and osteoblastic differentiation was evident |
MSCs |
217
|
|
|
|
|
EPCs |
|
CH–HA/PAA (base) |
PEEK and titanium rods and sheets |
N/A |
CH–HA/PAA eased mechanical mismatch and encouraged deposition of cohesive trabecular bone on the implant surface |
MSCs |
211
|
Poly-β-amino ester 2/PAA/BMP-2/PAA (drug delivery system) |
|
|
Tuned release of BMP-2 controlled by hydrolytic degradation of poly-β-amino ester 2 |
|
|
PEI/PAA/PEI/nanoclay |
Polyurethane open cell foam |
Altered assembly conditions: pH of solutions, salt concentration, contact time between solution and template, number of layers deposited and cyclic loading crosslinked at 180 °C |
Tunable mechanical (compressive strength and stiffness) and physical (density and porosity) properties of coated foams. Crosslinked coatings biocompatible with MSCs |
MSCs |
51
|
Thermally crosslinked PAA capping layers |
|
|
|
|
|
Collagen/HA binding peptide/BMP-4 |
Fluorenylmethyloxycarbonyl protected valyl-cetylamide nanofibers |
Assembled template was incubated with HA nanocrystals blended with TiO2 nanoparticles and coated with alginate to form a 3D scaffold |
Coated scaffolds were biodegradable, biocompatible, allowed for adhesion of pre-osteoblast cells, promoted osteogenic differentiation and demonstrated inherent antibacterial activity |
MC3T3 |
214
|
Chitosan/sodium hyaluronate |
3D-printed hydroxyapatite–gelatin scaffolds |
Scaffolds were coated with 10 and 20 bilayers of the coating and then freeze-dried |
Swelling, mechanical and degradation properties were improved following the application of the LbL-assembled coating. Scaffolds provided appropriate conditions for cell adhesion and proliferation of MC3T3-E1 cells |
MC3T3-E1 |
58
|
4 Concluding remarks and future perspective
An ongoing challenge for biomaterials remains to satisfy the very diverse requirements for engineered tissue within a single material system. LbL assembly offers the advantage of a large variety of constituents that can be combined into a single multilayered material system, and is a relatively simple and low-cost coating technique to implement onto a wide variety of substrate materials. In recent years a range of new methods and materials have been explored for the development of LbL-assembled materials. These studies have reported the capability to provide controlled thickness, mechanical reinforcement, local control of mechanical stiffness, biodegradability, biologically useful electroactivity, enhanced cell and protein adhesion, and drug delivery, and to enable hierarchical microstructural features. LbL assembly has been implemented for deposition onto planar 2D substrates and 3D porous substrates, resulting in larger-scale bulk materials. Several examples of LbL-assembled material systems that satisfy one or more of the key requirements of biomaterials for bone tissue engineering, including tailored mechanical properties on bulk and local scales, a porous micro-architecture, biodegradability, biocompatibility and bioactivity, and the delivery of drugs and growth factors have been developed. More recently, combinations of different multilayers have been reported to produce LbL-assembled coatings with multifunctional capabilities derived from combining coatings that satisfy different requirements (e.g. mechanically reinforcing layers combined with drug delivery layers).
The application of the LbL approach for bone scaffold materials in bone repair and regeneration is full of opportunities and challenges. Despite the development of many new technologies and approaches for bone tissue repair and regeneration, enormous scope for further progress remains. Going forward, LbL assembly presents a novel approach for the further development of synergistic combinations of multilayer material systems that individually satisfy one or more requirements and that together can potentially meet all of the diverse needs within a combined coating material system for bone tissue engineering applications. Progress toward this goal has the strong potential for exciting technological advances and high impact in the repair of bone defects and a wider range of biomedical applications. In the past decade, the development in LbL assembly in the biomedical and tissue engineering fields has been growing rapidly, and it is expected that a dramatic increase in demand for academic and industrial applications will be witnessed in the design and fabrication of multifunctional coatings using this LbL approach.
LbL assembly is a highly versatile and simple approach for multilayer coatings in order to enhance the mechanical, biological, chemical and physical properties of biomaterials. This new technique has attracted biomaterials researchers in the last decade and probably in the next few coming years has great ability to be considered more than ever because it has excellent capability to fabricate mechanically robust multilayer coatings with controlled micro and nanostructures, controlled degradation rate, and above all tunable local surface stiffness from extensive choices of usable materials for a wide range of biomedical applications. The LbL assembly approach, because of the versatility and tunability to fabricate multilayer coatings with controllable structures and mechanical properties, will be considered as a promising technique in the field of biomaterials for tissue repair. LbL has also strong potential for application as a method for cell encapsulation in cell-based biosensors, cell transplantation, cell/molecule delivery, and tissue engineering in the near future. The LbL assembly technique not only provides advances for surface modification with nanoscale control but also opens the new door of biomaterials for the fabrication of novel deposited scaffolds for tissue engineering applications. In conclusion, the application of the LbL approach for bone scaffold materials in bone repair and regeneration is full of opportunities and challenges. In the future, further studies on the effects of coating mechanisms using loading conditions and perfusion on the scaffold materials coated on bone cell behaviour and bone repair under hydrated conditions will be developed in bone tissue engineering. The development of the LbL technique occurs, mainly in the biomedical and tissue engineering field, and it is expected that a dramatic increase in demand for academic and industrial applications will be witnessed in the design and fabrication of multifunctional coatings by the LbL approach.
Conflicts of interest
There are no conflicts to declare.
Acknowledgements
The research conducted in this publication was funded by the Irish Research Council (Government of Ireland Scholarship Scheme) under the award number GOIPG/2020/1344.
Notes and references
- P. V. Giannoudis, H. Dinopoulos and E. Tsiridis, Bone substitutes: an update, Injury, 2005, 36(3), S20–S27 CrossRef PubMed.
- A. R. Vaccaro, The role of the osteoconductive scaffold in synthetic bone graft, Orthopedics, 2002, 25(5), S571–S578 CrossRef PubMed.
- A. R. Amini, C. T. Laurencin and S. P. Nukavarapu, Bone tissue engineering: recent advances and challenges, Crit. Rev. Biomed. Eng., 2012, 40(5), 363–408 CrossRef PubMed.
- Global Bone Graft And Substitutes Market Report, 2021–2028 [Internet]. [cited 2021 Jul 20]. Available from: https://www.grandviewresearch.com/industry-analysis/bone-grafts-substitutes-market.
- V. Campana, G. I. Milano, E. Pagano, M. Barba, C. Cicione, G. Salonna, W. Lattanzi and G. Logroscino, Bone substitutes in orthopaedic surgery: from basic science to clinical practice, J. Mater. Sci.: Mater. Med., 2014, 25(10), 2445–2461 CrossRef CAS PubMed.
- S. Bose, M. Roy and A. Bandyopadhyay, Recent advances in bone tissue engineering scaffolds, Trends Biotechnol., 2012, 30(10), 546–554 CrossRef CAS PubMed.
- R. Fradique, T. R. Correia, S. P. Miguel, K. D. De Sa, D. R. Figueira, A. G. Mendonça and I. J. Correia, Production of new 3D scaffolds for bone tissue regeneration by rapid prototyping, J. Mater. Sci.: Mater. Med., 2016, 27(4), 1–4 CrossRef CAS PubMed.
- S. J. Hollister, Scaffold design and manufacturing: from concept to clinic, Adv. Mater., 2009, 21(32–33), 3330–3342 CrossRef CAS PubMed.
- A. J. Salgado, O. P. Coutinho and R. L. Reis, Bone tissue engineering: state of the art and future trends, Macromol. Biosci., 2004, 4(8), 743–765 CrossRef CAS PubMed.
- E. J. Sheehy, D. J. Kelly and F. J. O'Brien, Biomaterial-based endochondral bone regeneration: a shift from traditional tissue engineering paradigms to developmentally inspired strategies, Mater. Today Bio, 2019, 3, 100009 CrossRef CAS PubMed.
- Y. Li, H. Jahr, J. Zhou and A. A. Zadpoor, Additively manufactured biodegradable porous metals, Acta Biomater., 2020, 115, 29–50 CrossRef PubMed.
- E. Alsberg, H. J. Kong, Y. Hirano, M. K. Smith, A. Albeiruti and D. J. Mooney, Regulating bone formation via controlled scaffold degradation, J. Dent. Res., 2003, 82(11), 903–908 CrossRef CAS PubMed.
- D. W. Hutmacher, J. T. Schantz, C. X. Lam, K. C. Tan and T. C. Lim, State of the art and future directions of scaffold–based bone engineering from a biomaterials perspective, J. Tissue Eng. Regener. Med., 2007, 1(4), 245–260 CrossRef CAS PubMed.
- A. Matsumoto, H. Kajiya, M. N. Yamamoto, T. Yanagi, A. Imamura, K. Okabe, T. Fukushima, H. Kido and J. Ohno, Degradation rate of DNA scaffolds and bone regeneration, J. Biomed. Mater. Res., Part B, 2019, 107(1), 122–128 CrossRef CAS PubMed.
- P. Chocholata, V. Kulda and V. Babuska, Fabrication of scaffolds for bone-tissue regeneration, Materials, 2019, 12(4), 568 CrossRef CAS PubMed.
- M. Bahraminasab, Challenges on optimization of 3D-printed bone scaffolds, Biomed. Eng. Online, 2020, 19(1), 1–33 CrossRef PubMed.
- A. Eltom, G. Zhong and A. Muhammad, Scaffold techniques and designs in tissue engineering functions and purposes: a review, Adv. Mater. Sci. Eng., 2019, 2019 Search PubMed.
- M. Drabik, A. Grzeczkowicz, P. Bącal, A. Kwiatkowska, M. Strawski, M. Antosiak-Iwańska, B. Kazimierczak, E. Godlewska and L. H. Granicka, Nanocomposite membrane scaffolds for cell function maintaining for biomedical purposes, Nanomaterials, 2021, 11(5), 1094 CrossRef CAS PubMed.
- P. Kazimierczak and A. Przekora, Osteoconductive and osteoinductive surface modifications of biomaterials for bone regeneration: A concise review, Coatings, 2020, 10(10), 971 CrossRef CAS.
- M. A. Velasco, C. A. Narváez-Tovar and D. A. Garzón-Alvarado, Design, materials, and mechanobiology of biodegradable scaffolds for bone tissue engineering, BioMed Res. Int., 2015, 2015 Search PubMed.
- X. Zhang, Y. Xu, X. Zhang, H. Wu, J. Shen, R. Chen, Y. Xiong, J. Li and S. Guo, Progress on the layer-by-layer assembly of multilayered polymer composites: Strategy, structural control and applications, Prog. Polym. Sci., 2019, 89, 76–107 CrossRef CAS.
- A. Devlin–Mullin, N. M. Todd, Z. Golrokhi, H. Geng, M. A. Konerding, N. G. Ternan, J. A. Hunt, R. J. Potter, C. Sutcliffe, E. Jones and P. D. Lee, Atomic layer deposition of a silver nanolayer on advanced titanium orthopedic implants inhibits bacterial colonization and supports vascularized de novo bone ingrowth, Adv. Healthcare Mater., 2017, 6(11), 1700033 CrossRef PubMed.
- R. Bosco, J. Van Den Beucken, S. Leeuwenburgh and J. Jansen, Surface engineering for bone implants: a trend from passive to active surfaces, Coatings, 2012, 2(3), 95–119 CrossRef CAS.
- M. Fousova, D. Vojtech, E. Jablonska, J. Fojt and J. Lipov, Novel approach in the use of plasma spray: Preparation of bulk titanium for bone augmentations, Materials, 2017, 10(9), 987 CrossRef PubMed.
- Y. Zhang, D. Zhai, M. Xu, Q. Yao, H. Zhu, J. Chang and C. Wu, 3D-printed bioceramic scaffolds with antibacterial and osteogenic activity, Biofabrication, 2017, 9(2), 025037 CrossRef PubMed.
- D. Alkekhia, P. T. Hammond and A. Shukla, Layer-by-layer biomaterials for drug delivery, Annu. Rev. Biomed. Eng., 2020, 22, 1–24 CrossRef CAS PubMed.
- S. Cao, Y. Zhao, Y. Hu, L. Zou and J. Chen, New perspectives: In situ tissue engineering for bone repair scaffold, Composites, Part B, 2020, 202, 108445 CrossRef CAS.
- W. Wang, Y. Xu, S. Backes, A. Li, S. Micciulla, A. B. Kayitmazer, L. Li, X. Guo and R. von Klitzing, Construction of compact polyelectrolyte multilayers inspired by marine mussel: effects of salt concentration and pH as observed by QCM-D and AFM, Langmuir, 2016, 32(14), 3365–3374 CrossRef CAS PubMed.
- T. Boudou, T. Crouzier, K. Ren, G. Blin and C. Picart, Multiple functionalities of polyelectrolyte multilayer films: new biomedical applications, Adv. Mater., 2010, 22(4), 441–467 CrossRef CAS PubMed.
- H. Ai, S. A. Jones and Y. M. Lvov, Biomedical applications of electrostatic layer-by-layer nano-assembly of polymers, enzymes, and nanoparticles, Cell Biochem. Biophys., 2003, 39(1), 23–43 CrossRef CAS PubMed.
- Q. Chen, W. Li, Q. Yao, R. Liang, R. Pérez-Garcia, J. Munoz and A. R. Boccaccini, Multilayered drug delivery coatings composed of daidzein-loaded PHBV microspheres embedded in a biodegradable polymer matrix by electrophoretic deposition, J. Mater. Chem. B, 2016, 4(29), 5035–5045 RSC.
- P. Gentile, I. Carmagnola, T. Nardo and V. Chiono, Layer-by-layer assembly for biomedical applications in the last decade, Nanotechnology, 2015, 26(42), 422001 CrossRef CAS PubMed.
- K. Kirchhof, K. Hristova, N. Krasteva, G. Altankov and T. Groth, Multilayer coatings on biomaterials for control of MG-63 osteoblast adhesion and growth, J. Mater. Sci.: Mater. Med., 2009, 20(4), 897–907 CrossRef CAS PubMed.
- L. Ma, J. Zhou, C. Gao and J. Shen, Incorporation of basic fibroblast growth factor by a layer-by-layer assembly technique to produce bioactive substrates, J. Biomed. Mater. Res., Part B, 2007, 83(1), 285–292 CrossRef PubMed.
- J. S. Martinez, T. C. Keller III and J. B. Schlenoff, Cytotoxicity of free versus multilayered polyelectrolytes, Biomacromolecules, 2011, 12(11), 4063–4070 CrossRef CAS PubMed.
- C. J. Detzel, A. L. Larkin and P. Rajagopalan, Polyelectrolyte multilayers in tissue engineering, Tissue Eng., Part B, 2011, 17(2), 101–113 CrossRef CAS PubMed.
- H. Rezaei, M. Shahrezaee, M. Jalali Monfared, F. Ghorbani, A. Zamanian and M. Sahebalzamani, Mussel-inspired polydopamine induced the osteoinductivity to ice-templating PLGA-gelatin matrix for bone tissue engineering application, Biotechnol. Appl. Biochem., 2021, 68(1), 185–196 CrossRef CAS PubMed.
- V. Gribova, R. Auzely-Velty and C. Picart, Polyelectrolyte multilayer assemblies on materials surfaces: from cell adhesion to tissue engineering, Chem. Mater., 2012, 24(5), 854–869 CrossRef CAS PubMed.
- Y. Yan, H. Chen, H. Zhang, C. Guo, K. Yang, K. Chen, R. Cheng, N. Qian, N. Sandler, Y. S. Zhang and H. Shen, Vascularized 3D printed scaffolds for promoting bone regeneration, Biomaterials, 2019, 190, 97–110 CrossRef PubMed.
- A. M. Ferreira, C. Tonda-Turo, E. Mancuso and P. Gentile, Multilayer nanoscale functionalization to treat disorders and enhance regeneration of bone tissue, Nanomedicine, 2019, 19, 22–38 CrossRef CAS PubMed.
- S. A. Yavari, M. Croes, B. Akhavan, F. Jahanmard, C. C. Eigenhuis, S. Dadbakhsh, H. C. Vogely, M. M. Bilek, A. C. Fluit, C. H. Boel and B. C. van der Wal, Layer by layer coating for bio-functionalization of additively manufactured meta-biomaterials, Addit. Manuf., 2020, 32, 100991 Search PubMed.
- Y. Qian, L. Li, Y. Song, L. Dong, P. Chen, X. Li, K. Cai, O. Germershaus, L. Yang and Y. Fan, Surface modification of nanofibrous matrices via layer-by-layer functionalized silk assembly for mitigating the foreign body reaction, Biomaterials, 2018, 164, 22–37 CrossRef CAS PubMed.
- J. Song, B. Winkeljann and O. Lieleg, Biopolymer–Based Coatings: Promising Strategies to Improve the Biocompatibility and Functionality of Materials Used in Biomedical Engineering, Adv. Mater. Interfaces, 2020, 7(17), 2000850 CrossRef CAS.
- X. Ma, G. Wu, F. Dai, D. Li, H. Li, L. Zhang and H. Deng, Chitosan/polydopamine layer by layer self-assembled silk fibroin nanofibers for biomedical applications, Carbohydr. Polym., 2021, 251, 117058 CrossRef CAS PubMed.
- Z. Tang, Y. Wang, P. Podsiadlo and N. A. Kotov, Biomedical applications of layer–by–layer assembly: from biomimetics to tissue engineering, Adv. Mater., 2006, 18(24), 3203–3224 CrossRef CAS.
- J. Lipton, G. M. Weng, J. A. Röhr, H. Wang and A. D. Taylor, Layer-by-layer assembly of two-dimensional materials: meticulous control on the nanoscale, Matter, 2020, 2(5), 1148–1165 CrossRef.
- M. Ziminska, N. Dunne and A. R. Hamilton, Porous materials with tunable structure and mechanical properties via templated layer-by-layer assembly, ACS Appl. Mater. Interfaces, 2016, 8(34), 21968–21973 CrossRef CAS PubMed.
- G. Ladam, P. Schaad, J. C. Voegel, P. Schaaf, G. Decher and F. Cuisinier, In situ determination of the structural properties of initially deposited polyelectrolyte multilayers, Langmuir, 2000, 16(3), 1249–1255 CrossRef CAS.
- O. Azzaroni and K. A. Lau, Layer-by-layer assemblies in nanoporous templates: nano-organized design and applications of soft nanotechnology, Soft Matter, 2011, 7(19), 8709–8724 RSC.
- A. E. El Haitami, J. S. Thomann, L. Jierry, A. Parat, J. C. Voegel, P. Schaaf, B. Senger, F. Boulmedais and B. Frisch, Covalent layer-by-layer assemblies of polyelectrolytes and homobifunctional spacers, Langmuir, 2010, 26(14), 12351–12357 CrossRef CAS PubMed.
- M. Ziminska, M. J. Chalanqui, P. Chambers, J. G. Acheson, H. O. McCarthy, N. J. Dunne and A. R. Hamilton, Nanocomposite-coated porous templates for engineered bone scaffolds: A parametric study of layer-by-layer assembly conditions, Biomed. Mater., 2019, 14(6), 065008 CrossRef CAS PubMed.
- M. Michel, V. Toniazzo, D. Ruch and V. Ball, Deposition mechanisms in layer-by-layer or step-by-step deposition methods: From elastic and impermeable films to soft membranes with ion exchange properties, ISRN Mater. Sci., 2012, 2012 Search PubMed.
- O. S. Manoukian, A. Aravamudhan, P. Lee, M. R. Arul, X. Yu, S. Rudraiah and S. G. Kumbar, Spiral layer-by-layer micro-nanostructured scaffolds for bone tissue engineering, ACS Biomater. Sci. Eng., 2018, 4(6), 2181–2192 CrossRef CAS PubMed.
- A. M. Yola, J. Campbell and D. Volodkin, Microfluidics meets layer-by-layer assembly for the build-up of polymeric scaffolds, Appl. Surf. Sci. Adv., 2021, 5, 100091 CrossRef.
- M. Salomäki, L. Marttila, H. Kivelä, M. Tupala and J. Lukkari, Oxidative Spin-Spray-Assembled Coordinative Multilayers as Platforms for Capacitive Films, Langmuir, 2020, 36(24), 6736–6748 CrossRef PubMed.
- K. Ariga, E. Ahn, M. Park and B. S. Kim, Layer–by–layer assembly: recent progress from layered assemblies to layered nanoarchitectonics, Chem. – Asian J., 2019, 14(15), 2553–2566 CrossRef CAS PubMed.
- Y. Li, X. Wang and J. Sun, Layer-by-layer assembly for rapid fabrication of thick polymeric films, Chem. Soc. Rev., 2012, 41(18), 5998–6009 RSC.
- S. Chen, Y. Shi, Y. Luo and J. Ma, Layer-by-layer coated porous 3D printed hydroxyapatite composite scaffolds for controlled drug delivery, Colloids Surf., B, 2019, 179, 121–127 CrossRef CAS PubMed.
- J. Kong, B. Wei, T. Groth, Z. Chen, L. Li, D. He, R. Huang, J. Chu and M. Zhao, Biomineralization improves mechanical and osteogenic properties of multilayer–modified PLGA porous scaffolds, J. Biomed. Mater. Res., Part A, 2018, 106(10), 2714–2725 CrossRef CAS PubMed.
- J. M. Mets, J. T. Wilson, W. Cui and E. L. Chaikof, An Automated Process for Layer–by–Layer Assembly of Polyelectrolyte Multilayer Thin Films on Viable Cell Aggregates, Adv. Healthcare Mater., 2013, 2(2), 266–270 CrossRef CAS PubMed.
- S. Park, U. Han, D. Choi and J. Hong, Layer-by-layer assembled polymeric thin films as prospective drug delivery carriers: design and applications, Biomater. Res., 2018, 22(1), 1–3 CrossRef PubMed.
- S. Vozar, Y. C. Poh, T. Serbowicz, M. Bachner, P. Podsiadlo, M. Qin, E. Verploegen, N. Kotov and A. J. Hart, Automated spin-assisted layer-by-layer assembly of nanocomposites, Rev. Sci. Instrum., 2009, 80(2), 023903 CrossRef PubMed.
- J. J. Richardson, M. Björnmalm and F. Caruso, Technology-driven layer-by-layer assembly of nanofilms, Science, 2015, 348(6233), aaa2491 CrossRef PubMed.
- C. Cho, K. L. Wallace, D. A. Hagen, B. Stevens, O. Regev and J. C. Grunlan, Nanobrick wall multilayer thin films grown faster and stronger using electrophoretic deposition, Nanotechnology, 2015, 26(18), 185703 CrossRef PubMed.
- C. M. Andres, M. L. Fox and N. A. Kotov, Traversing material scales: macroscale LBL-assembled nanocomposites with microscale inverted colloidal crystal architecture, Chem. Mater., 2012, 24(1), 9–11 CrossRef CAS PubMed.
- H. Lee, Y. Lee, A. R. Statz, J. Rho, T. G. Park and P. B. Messersmith, Substrate–independent layer–by–layer assembly by using mussel–adhesive–inspired polymers, Adv. Mater., 2008, 20(9), 1619–1623 CrossRef CAS PubMed.
- C. Porcel, P. Lavalle, G. Decher, B. Senger, J. C. Voegel and P. Schaaf, Influence of the polyelectrolyte molecular weight on exponentially growing multilayer films in the linear regime, Langmuir, 2007, 23(4), 1898–1904 CrossRef CAS PubMed.
- P. Lavalle, C. Picart, J. Mutterer, C. Gergely, H. Reiss, J. C. Voegel, B. Senger and P. Schaaf, Modeling the buildup of polyelectrolyte multilayer films having exponential growth, J. Phys. Chem. B, 2004, 108(2), 635–648 CrossRef CAS.
- E. D. de Avila, A. G. Castro, O. Tagit, B. P. Krom, D. Löwik, A. A. van Well, L. J. Bannenberg, C. E. Vergani and J. J. van den Beucken, Anti-bacterial efficacy via drug-delivery system from layer-by-layer coating for percutaneous dental implant components, Appl. Surf. Sci., 2019, 488, 194–204 CrossRef CAS.
- C. A. Ghiorghita, F. Bucatariu and E. S. Dragan, Influence of cross-linking in loading/release applications of polyelectrolyte multilayer assemblies. A review, Mater. Sci. Eng., C, 2019, 105, 110050 CrossRef CAS PubMed.
- D. S. Linnik, Y. V. Tarakanchikova, M. V. Zyuzin, K. V. Lepik, J. L. Aerts, G. Sukhorukov and A. S. Timin, Layer-by-Layer technique as a versatile tool for gene delivery applications, Expert Opin. Drug Delivery, 2021, 18(8), 1047–1066 CrossRef CAS PubMed.
- C. Picart, J. Mutterer, L. Richert, Y. Luo, G. D. Prestwich, P. Schaaf, J. C. Voegel and P. Lavalle, Molecular basis for the explanation of the exponential growth of polyelectrolyte multilayers, Proc. Natl. Acad. Sci. U. S. A., 2002, 99(20), 12531–12535 CrossRef CAS PubMed.
- P. Podsiadlo, Z. Tang, B. S. Shim and N. A. Kotov, Counterintuitive effect of molecular strength and role of molecular rigidity on mechanical properties of layer-by-layer assembled nanocomposites, Nano Lett., 2007, 7(5), 1224–1231 CrossRef CAS PubMed.
- C. Husteden, F. Doberenz, N. Goergen, S. R. Pinnapireddy, C. Janich, A. Langner, F. Syrowatka, A. Repanas, F. Erdmann, J. Jedelska and U. Bakowsky, Contact-triggered lipofection from multilayer films designed as surfaces for in situ transfection strategies in tissue engineering, ACS Appl. Mater. Interfaces, 2020, 12(8), 8963–8977 CrossRef CAS PubMed.
- D. Kim, P. Tzeng, K. J. Barnett, Y. H. Yang, B. A. Wilhite and J. C. Grunlan, Highly size–selective ionically crosslinked multilayer polymer films for light gas separation, Adv. Mater., 2014, 26(5), 746–751 CrossRef CAS PubMed.
- R. M. DuChanois, R. Epsztein, J. A. Trivedi and M. Elimelech, Controlling pore structure of polyelectrolyte multilayer nanofiltration membranes by tuning polyelectrolyte-salt interactions, J. Membr. Sci., 2019, 581, 413–420 CrossRef CAS.
- S. T. Dubas and J. B. Schlenoff, Swelling and smoothing of polyelectrolyte multilayers by salt, Langmuir, 2001, 17(25), 7725–7727 CrossRef CAS.
- D. F. Kienle, A. F. Chaparro Sosa, J. L. Kaar and D. K. Schwartz, Polyelectrolyte multilayers enhance the dry storage and pH stability of physically entrapped enzymes, ACS Appl. Mater. Interfaces, 2020, 12(20), 22640–22649 CrossRef CAS PubMed.
- J. Fu, J. Ji, L. Shen, A. Küller, A. Rosenhahn, J. Shen and M. Grunze, pH-amplified exponential growth multilayers: a facile method to develop hierarchical micro-and nanostructured surfaces, Langmuir, 2009, 25(2), 672–675 CrossRef CAS PubMed.
- F. Han, P. Zhang, T. Chen, C. Lin, X. Wen and P. Zhao, A LbL-assembled bioactive coating modified nanofibrous membrane for rapid tendon-bone healing in ACL reconstruction, Int. J. Nanomed., 2019, 14, 9159 CrossRef CAS PubMed.
- D. Mertz, J. Hemmerlé, J. Mutterer, S. Ollivier, J. C. Voegel, P. Schaaf and P. Lavalle, Mechanically responding nanovalves based on polyelectrolyte multilayers, Nano Lett., 2007, 7(3), 657–662 CrossRef CAS PubMed.
- Y. Wang, E. Jan, M. Cuddihy, J. H. Bahng and N. Kotov, Layered biomimetic nanocomposites replicate bone surface in three-dimensional cell cultures, Nanocomposites, 2018, 4(4), 156–166 CrossRef CAS.
- W. Zhang, Y. Yang and B. Cui, New perspectives on the roles of nanoscale surface topography in modulating intracellular signaling, Curr. Opin. Solid State Mater. Sci., 2021, 25(1), 100873 CrossRef CAS PubMed.
- K. E. Tanner, Bioactive composites for bone tissue engineering, Proc. Inst. Mech. Eng., Part H, 2010, 224(12), 1359–1372 CrossRef CAS PubMed.
-
A. M. Tarawneh, M. Wettergreen and M. A. K. Liebschner, Computer-Aided Tissue Engineering: Benefiting from the Control Over Scaffold Micro-Architecture, in Computer-Aided Tissue Engineering, ed. M. A. K. Liebschner, Humana Press, Totowa, NJ, 2012, pp. 1–25 [cited 2016 Oct 20]. Available from: https://link.springer.com/10.1007/978-1-61779-764-4_1 Search PubMed.
- F. G. Aliev, M. A. Correa-Duarte, A. Mamedov, J. W. Ostrander, M. Giersig, L. M. Liz-Marzán and N. A. Kotov, Layer–By–Layer Assembly of Core–Shell Magnetite Nanoparticles: Effect of Silica Coating on Interparticle Interactions and Magnetic Properties, Adv. Mater., 1999, 11(12), 1006–1010 CrossRef CAS.
- R. Puffr, J. L. Špátová and J. Brožek, Clay mineral/polyamide nanocomposites obtained by in situ polymerization or melt intercalation, Appl. Clay Sci., 2013, 83, 294–299 CrossRef.
- X. Tang, S. Alavi and T. J. Herald, Barrier and mechanical properties of starch–clay nanocomposite films, Cereal Chem., 2008, 85(3), 433–439 CrossRef CAS.
- M. P. Nikolova and M. S. Chavali, Recent advances in biomaterials for 3D scaffolds: A review, Bioact. Mater., 2019, 4, 271–292 CrossRef PubMed.
- Z. Tang, N. A. Kotov, S. Magonov and B. Ozturk, Nanostructured artificial nacre, Nat. Mater., 2003, 2(6), 413–418 CrossRef CAS PubMed.
- P. Podsiadlo, A. K. Kaushik, E. M. Arruda, A. M. Waas, B. S. Shim, J. Xu, H. Nandivada, B. G. Pumplin, J. Lahann, A. Ramamoorthy and N. A. Kotov, Ultrastrong and stiff layered polymer nanocomposites, Science, 2007, 318(5847), 80–83 CrossRef CAS PubMed.
- P. Podsiadlo, M. Michel, J. Lee, E. Verploegen, N. Wong Shi Kam, V. Ball, J. Lee, Y. Qi, A. J. Hart, P. T. Hammond and N. A. Kotov, Exponential growth of LBL films with incorporated inorganic sheets, Nano Lett., 2008, 8(6), 1762–1770 CrossRef CAS PubMed.
- A. A. Mamedov, N. A. Kotov, M. Prato, D. M. Guldi, J. P. Wicksted and A. Hirsch, Molecular design of strong single-wall carbon nanotube/polyelectrolyte multilayer composites, Nat. Mater., 2002, 1(3), 190–194 CrossRef CAS PubMed.
- X. Fan, M. K. Park, C. Xia and R. Advincula, Surface structural characterization and mechanical testing by nanoindentation measurements of hybrid polymer/clay nanostructured multilayer films, J. Mater. Res., 2002, 17(7), 1622–1633 CrossRef CAS.
- P. Augat, M. Hollensteiner and C. von Rüden, The role of mechanical stimulation in the enhancement of bone healing, Injury, 2021, 52, S78–S83 CrossRef PubMed.
- J. D. Boerckel, Y. M. Kolambkar, H. Y. Stevens, A. S. Lin, K. M. Dupont and R. E. Guldberg, Effects of in vivo mechanical loading on large bone defect regeneration, J. Orthop. Res., 2012, 30(7), 1067–1075 CrossRef PubMed.
- A. M. Yousefi, P. F. James, R. Akbarzadeh, A. Subramanian, C. Flavin and H. Oudadesse, Prospect of stem cells in bone tissue engineering: a review, Stem Cells Int., 2016, 2016 Search PubMed.
- R. Olivares-Navarrete, E. M. Lee, K. Smith, S. L. Hyzy, M. Doroudi, J. K. Williams, K. Gall, B. D. Boyan and Z. Schwartz, Substrate stiffness controls osteoblastic and chondrocytic differentiation of mesenchymal stem cells without exogenous stimuli, PLoS One, 2017, 12(1), 1–18 CrossRef PubMed.
- O. Chaudhuri, L. Gu, D. Klumpers, M. Darnell, S. A. Bencherif, J. C. Weaver, N. Huebsch, H. P. Lee, E. Lippens, G. N. Duda and D. J. Mooney, Hydrogels with tunable stress relaxation regulate stem cell fate and activity, Nat. Mater., 2016, 15(3), 326–334 CrossRef CAS PubMed.
- K. Kim, A. Yeatts, D. Dean and J. P. Fisher, Stereolithographic bone scaffold design parameters: osteogenic differentiation and signal expression, Tissue Eng., Part B, 2010, 16(5), 523–539 CrossRef CAS PubMed.
- A. J. Engler, S. Sen, H. L. Sweeney and D. E. Discher, Matrix elasticity directs stem cell lineage specification, Cell, 2006, 126(4), 677–689 CrossRef CAS PubMed.
- R. G. Breuls, T. U. Jiya and T. H. Smit, Scaffold stiffness influences cell behavior: opportunities for skeletal tissue engineering, Open Orthop. J., 2008, 2, 103 CrossRef PubMed.
- K. F. Eichholz, I. Woods, M. Riffault, G. P. Johnson, M. Corrigan, M. C. Lowry, N. Shen, M. N. Labour, K. Wynne, L. O'Driscoll and D. A. Hoey, Human bone marrow stem/stromal cell osteogenesis is regulated via mechanically activated osteocyte-derived extracellular vesicles, Stem Cells Transl. Med., 2020, 9(11), 1431–1447 CrossRef CAS PubMed.
- C. B. Horner, M. Maldonado, Y. Tai, R. I. K. Rony and J. Nam, Spatially regulated multiphenotypic differentiation of stem cells in 3D via engineered mechanical gradient, ACS Appl. Mater. Interfaces, 2019, 11(49), 45479–45488 CrossRef CAS PubMed.
- G. Yourek, S. M. McCormick, J. J. Mao and G. C. Reilly, Shear stress induces osteogenic differentiation of human mesenchymal stem cells, Regener. Med., 2010, 5(5), 713–724 CrossRef CAS PubMed.
- B. Pilicheva, Y. Uzunova, I. Bodurov, A. Viraneva, G. Exner, S. Sotirov, T. Yovcheva and M. Marudova, Layer-by-layer self-assembly films for buccal drug delivery: The effect of polymer cross-linking, J. Drug Delivery Sci. Technol., 2020, 59, 101897 CrossRef CAS.
- W. Yuan, G. M. Weng, J. Lipton, C. M. Li, P. R. Van Tassel and A. D. Taylor, Weak polyelectrolyte-based multilayers via layer-by-layer assembly: Approaches, properties, and applications, Adv. Colloid Interface Sci., 2020, 282, 102200 CrossRef CAS PubMed.
- T. Swift, pH Dependence of Acrylate-Derivative Polyelectrolyte Properties, Acrylate Polym. Adv. Appl., 2019, 1–19 Search PubMed.
- Y. H. Yang, M. Haile, Y. T. Park, F. A. Malek and J. C. Grunlan, Super gas barrier of all-polymer multilayer thin films, Macromolecules, 2011, 44(6), 1450–1459 CrossRef CAS.
- Y. C. Li, J. Schulz and J. C. Grunlan, Polyelectrolyte/nanosilicate thin-film assemblies: influence of pH on growth, mechanical behavior, and flammability, ACS Appl. Mater. Interfaces, 2009, 1(10), 2338–2347 CrossRef CAS PubMed.
- H. Mjahed, G. Cado, F. Boulmedais, B. Senger, P. Schaaf, V. Ball and J. C. Voegel, Restructuring of exponentially growing polyelectrolyte multilayer films induced by salt concentration variations after film deposition, J. Mater. Chem., 2011, 21(23), 8416–8421 RSC.
- S. H. Oh, D. B. An, T. H. Kim and J. H. Lee, Wide-range stiffness gradient PVA/HA hydrogel to investigate stem cell differentiation behavior, Acta Biomater., 2016, 35, 23–31 CrossRef CAS PubMed.
-
J. Acheson, M. Ziminska, S. Goel, N. Dunne and A. Hamilton, Effect of hydration on the mechanical behaviour of nanocomposite-coated porous bone scaffold materials. In United Kingdom Society of Biomaterials 2015 Jun 25.
- K. Ren, T. Crouzier, C. Roy and C. Picart, Polyelectrolyte multilayer films of controlled stiffness modulate myoblast cell differentiation, Adv. Funct. Mater., 2008, 18(9), 1378–1389 CrossRef CAS PubMed.
- J. Almodóvar, T. Crouzier, Š. Selimović, T. Boudou, A. Khademhosseini and C. Picart, Gradients of physical and biochemical cues on polyelectrolyte multilayer films generated via microfluidics, Lab Chip, 2013, 13(8), 1562–1570 RSC.
- I. Hopp, A. Michelmore, L. E. Smith, D. E. Robinson, A. Bachhuka, A. Mierczynska and K. Vasilev, The influence of substrate stiffness gradients on primary human dermal fibroblasts, Biomaterials, 2013, 34(21), 5070–5077 CrossRef CAS PubMed.
- Y. Yang, K. Wang, X. Gu and K. W. Leong, Biophysical regulation of cell behavior—cross talk between substrate stiffness and nanotopography, Engineering, 2017, 3(1), 36–54 CrossRef CAS PubMed.
-
W. Huang, Clay nanopapers, in Nanopapers, William Andrew Publishing, 2018, pp. 59–86 Search PubMed.
- D. E. Discher, P. Janmey and Y. L. Wang, Tissue cells feel and respond to the stiffness of their substrate, Science, 2005, 310(5751), 1139–1143 CrossRef CAS PubMed.
- F. Gaudière, S. Morin-Grognet, L. Bidault, P. Lembré, E. Pauthe, J. P. Vannier, H. Atmani, G. Ladam and B. Labat, Genipin-cross-linked layer-by-layer assemblies: biocompatible microenvironments to direct bone cell fate, Biomacromolecules, 2014, 15(5), 1602–1611 CrossRef PubMed.
- C. F. Guimarães, L. Gasperini, A. P. Marques and R. L. Reis, The stiffness of living tissues and its implications for tissue engineering, Nat. Rev. Mater., 2020, 5(5), 351–370 CrossRef.
- A. S. Rowlands, P. A. George and J. J. Cooper-White, Directing osteogenic and myogenic differentiation of MSCs: interplay of stiffness and adhesive ligand presentation, Am. J. Physiol.: Cell Physiol., 2008, 295(4), C1037–C1044 CrossRef CAS PubMed.
- J. Solon, I. Levental, K. Sengupta, P. C. Georges and P. A. Janmey, Fibroblast adaptation and stiffness matching to soft elastic substrates, Biophys. J., 2007, 93(12), 4453–4461 CrossRef CAS PubMed.
- J. S. Martinez, A. M. Lehaf, J. B. Schlenoff and T. C. Keller III, Cell durotaxis on polyelectrolyte multilayers with photogenerated gradients of modulus, Biomacromolecules, 2013, 14(5), 1311–1320 CrossRef CAS PubMed.
- F. Shi, Z. Wang, N. Zhao and X. Zhang, Patterned polyelectrolyte multilayer: Surface modification for enhancing selective adsorption, Langmuir, 2005, 21(4), 1599–1602 CrossRef CAS PubMed.
- H. W. Chien, T. Y. Chang and W. B. Tsai, Spatial control of cellular adhesion using photo-crosslinked micropatterned polyelectrolyte multilayer films, Biomaterials, 2009, 30(12), 2209–2218 CrossRef CAS PubMed.
- S. Wei, J. X. Ma, L. Xu, X. S. Gu and X. L. Ma, Biodegradable materials for bone defect repair, Mil. Med. Res., 2020, 7(1), 1–25 Search PubMed.
- M. Frőhlich, W. L. Grayson, L. Q. Wan, D. Marolt, M. Drobnic and G. Vunjak-Novakovic, Tissue engineered bone grafts: biological requirements, tissue culture and clinical relevance, Curr. Stem Cell Res. Ther., 2008, 3(4), 254–264 CrossRef PubMed.
- A. Schneider, C. Vodouhê, L. Richert, G. Francius, E. Le Guen, P. Schaaf, J. C. Voegel, B. Frisch and C. Picart, Multifunctional polyelectrolyte multilayer films: combining mechanical resistance, biodegradability, and bioactivity, Biomacromolecules, 2007, 8(1), 139–145 CrossRef CAS PubMed.
- J. M. Garza, N. Jessel, G. Ladam, V. Dupray, S. Muller, J. F. Stoltz, P. Schaaf, J. C. Voegel and P. Lavalle, Polyelectrolyte multilayers and degradable polymer layers as multicompartment films, Langmuir, 2005, 21(26), 12372–12377 CrossRef CAS PubMed.
- F. Wu, M. Misra and A. K. Mohanty, Challenges and new opportunities on barrier performance of biodegradable polymers for sustainable packaging, Prog. Polym. Sci., 2021, 117, 101395 CrossRef CAS.
- W. Xu, K. Yagoshi, Y. Koga, M. Sasaki and T. Niidome, Optimized polymer coating for magnesium alloy-based bioresorbable scaffolds for long-lasting drug release and corrosion resistance, Colloids Surf., B, 2018, 163, 100–106 CrossRef CAS PubMed.
- M. A. Khalili and E. Tamjid, Controlled biodegradation of magnesium alloy in physiological environment by metal organic framework nanocomposite coatings, Sci. Rep., 2021, 11(1), 1–3 CrossRef PubMed.
- S. Kunjukunju, A. Roy, M. Ramanathan, B. Lee, J. E. Candiello and P. N. Kumta, A layer-by-layer approach to natural polymer-derived bioactive coatings on magnesium alloys, Acta Biomater., 2013, 9(10), 8690–8703 CrossRef CAS PubMed.
- K. Cai, X. Sui, Y. Hu, L. Zhao, M. Lai, Z. Luo, P. Liu and W. Yang, Fabrication of anticorrosive multilayer onto magnesium alloy substrates via spin-assisted layer-by-layer technique, Mater. Sci. Eng., C, 2011, 31(8), 1800–1808 CrossRef CAS.
- P. Liu, X. Pan, W. Yang, K. Cai and Y. Chen, Improved anticorrosion of magnesium alloy via layer-by-layer self-assembly technique combined with micro-arc oxidation, Mater. Lett., 2012, 75, 118–121 CrossRef CAS.
- P. R. Kuzyk and E. H. Schemitsch, The science of electrical stimulation therapy for fracture healing, Indian J. Orthop., 2009, 43(2), 127 CrossRef PubMed.
- S. Mobini, L. Leppik, V. T. Parameswaran and J. H. Barker, In vitro effect of direct current electrical stimulation on rat mesenchymal stem cells, PeerJ, 2017, 5, e2821 CrossRef PubMed.
- C. Galli, G. Pedrazzi, M. Mattioli-Belmonte and S. Guizzardi, The use of pulsed electromagnetic fields to promote bone responses to biomaterials in vitro and in vivo, Int. J. Biomater., 2018, 2018 Search PubMed.
- H. Palza, P. A. Zapata and C. Angulo-Pineda, Electroactive smart polymers for biomedical applications, Materials, 2019, 12(2), 277 CrossRef CAS PubMed.
- G. Kaur, R. Adhikari, P. Cass, M. Bown and P. Gunatillake, Electrically conductive polymers and composites for biomedical applications, RSC Adv., 2015, 5(47), 37553–37567 RSC.
- D. Mawad, K. Gilmore, P. Molino, K. Wagner, P. Wagner, D. L. Officer and G. G. Wallace, An erodible polythiophene-based composite for biomedical applications, J. Mater. Chem., 2011, 21(15), 5555–5560 RSC.
- H. Cui, Y. Wang, L. Cui, P. Zhang, X. Wang, Y. Wei and X. Chen, In vitro studies on regulation of osteogenic activities by electrical stimulus on biodegradable electroactive polyelectrolyte multilayers, Biomacromolecules, 2014, 15(8), 3146–3157 CrossRef CAS PubMed.
- Z. Liu, L. Dong, K. Cheng, Z. Luo and W. Weng, Charge injection based electrical stimulation on polypyrrole planar electrodes to regulate cellular osteogenic differentiation, RSC Adv., 2018, 8(33), 18470–18479 RSC.
- Y. F. Chen, C. Goodheart and D. Rua, The Body's Cellular and Molecular Response to Protein-Coated Medical Device Implants: A Review Focused on Fibronectin and BMP Proteins, Int. J. Mol. Sci., 2020, 21(22), 8853 CrossRef CAS PubMed.
- A. B. Novaes Jr., S. L. Souza, R. R. Barros, K. K. Pereira, G. Iezzi and A. Piattelli, Influence of mplant surfaces on osseointegration, Braz. Dent. J., 2010, 21(6), 471–481 CrossRef PubMed.
- M. Ansari, Bone tissue regeneration: biology, strategies and interface studies, Prog. Biomater.,, 2019, 8(4), 223–237 CrossRef CAS PubMed.
- S. Zhang, M. Xing and B. Li, Biomimetic layer-by-layer self-assembly of nanofilms, nanocoatings, and 3D scaffolds for tissue engineering, Int. J. Mol. Sci., 2018, 19(6), 1641 CrossRef PubMed.
- S. Liang, Y. Guan and Y. Zhang, Layer-by-Layer Assembly of Microgel Colloidal Crystals via Photoinitiated Alkyne–Azide Click Reaction, ACS Omega, 2019, 4(3), 5650–5660 CrossRef CAS PubMed.
- P. Tryoen-Tóth, D. Vautier, Y. Haikel, J.-C. Voegel, P. Schaaf, J. Chluba and J. Ogier, Viability, adhesion, and bone phenotype of osteoblast-like cells on polyelectrolyte multilayer films, J. Biomed. Mater. Res., Part B, 2002, 60(4), 657–667 CrossRef PubMed.
- N. Faucheux, R. Schweiss, K. Lützow, C. Werner and T. Groth, Self-assembled monolayers with different terminating groups as model substrates for cell adhesion studies, Biomaterials, 2004, 25(14), 2721–2730 CrossRef CAS PubMed.
- X. Liu, L. Smith, G. Wei, Y. Won and P. X. Ma, Surface engineering of nano-fibrous poly (L-lactic acid) scaffolds via self-assembly technique for bone tissue engineering, J. Biomed. Nanotechnol., 2005, 1(1), 54–60 CrossRef CAS.
- H. Geckil, F. Xu, X. Zhang, S. Moon and U. Demirci, Engineering hydrogels as extracellular matrix mimics, Nanomedicine, 2010, 5(3), 469–484 CrossRef CAS PubMed.
- T. Hu, H. Xu, L. Sang, L. Wang, K. D. Dokiparty, H. Lv, J. Ouyang and X. Qiu, A novel layer-by-layer self-assembly biomimetic hydroxyapatite-cell sheet for bone tissue engineering, J. Biomater. Tissue Eng., 2016, 6(12), 931–937 CrossRef.
-
J. V. Nguyen and E. Ghafar-Zadeh, Biointerface materials for cellular adhesion: recent progress and future prospects, in Actuators, Multidisciplinary Digital Publishing Institute, 2020, vol. 9, No. 4, p. 137 Search PubMed.
- W. Gao, B. Feng, X. Lu, J. Wang, S. Qu and J. Weng, Characterization and cell behavior of titanium surfaces with PLL/DNA modification via a layer–by–layer technique, J. Biomed. Mater. Res., Part A, 2012, 100(8), 2176–2185 CrossRef PubMed.
- G. Delechiave, A. F. Naves, E. Kolanthai, R. A. da Silva, R. C. Vlasman, D. F. Petri, R. M. Torresi and L. H. Catalani, Tuning protein delivery from different architectures of layer-by-layer assemblies on polymer films, Mater, Adv,, 2020, 1(6), 2043–2056 RSC.
- J. M. Yang, R. Z. Tsai and C. C. Hsu, Protein adsorption on polyanion/polycation layer-by-layer assembled polyelectrolyte films, Colloids Surf., B, 2016, 142, 98–104 CrossRef CAS PubMed.
- R. Pei, X. Cui, X. Yang and E. Wang, Assembly of alternating polycation and DNA multilayer films by electrostatic layer-by-layer adsorption, Biomacromolecules, 2001, 2(2), 463–468 CrossRef CAS PubMed.
- S. Jiang, X. Chen and M. Liu, The pH stimulated reversible loading and release of a cationic dye in a layer-by-layer assembled DNA/PAH film, J. Colloid Interface Sci., 2004, 277(2), 396–403 CrossRef CAS PubMed.
- G. B. Sukhorukov, H. Möhwald, G. Decher and Y. M. Lvov, Assembly of polyelectrolyte multilayer films by consecutively alternating adsorption of polynucleotides and polycations, Thin Solid Films, 1996, 284, 220–223 CrossRef.
-
U. Jammalamadaka, K. Tappa and D. Mills, Osteoinductive calcium phosphate clay nanoparticle bone cements (CPCs) with enhanced mechanical properties, 36th Annual International Conference of the IEEE Engineering in Medicine and Biology Society, IEEE, 2014 Aug 26, pp. 3917–3920 Search PubMed.
- J. P. Govindharajulu, X. Chen, Y. Li, J. C. Rodriguez-Cabello, M. Battacharya and C. Aparicio, Chitosan-recombinamer layer-by-layer coatings for multifunctional implants, Int. J. Mol. Sci., 2017, 18(2), 369 CrossRef PubMed.
- D. M. Lynn and R. Langer, Degradable poly (β-amino esters): synthesis, characterization, and self-assembly with plasmid DNA, J. Am. Chem. Soc., 2000, 122(44), 10761–10768 CrossRef CAS.
- C. Romagnoli, F. D'Asta and M. L. Brandi, Drug delivery using composite scaffolds in the context of bone tissue engineering, Clin. Cases Miner. Bone Metab., 2013, 10(3), 155 Search PubMed.
- B. M. Wohl and J. F. Engbersen, Responsive layer-by-layer materials for drug delivery, J. Controlled Release, 2012, 158(1), 2–14 CrossRef CAS PubMed.
- V. Mouriño and A. R. Boccaccini, Bone tissue engineering therapeutics: controlled drug delivery in three-dimensional scaffolds, J. R. Soc., Interface, 2010, 7(43), 209–227 CrossRef PubMed.
- N. J. Shah, M. L. Macdonald, Y. M. Beben, R. F. Padera, R. E. Samuel and P. T. Hammond, Tunable dual growth factor delivery from polyelectrolyte multilayer films, Biomaterials, 2011, 32(26), 6183–6193 CrossRef CAS PubMed.
- R. Guillot, F. Gilde, P. Becquart, F. Sailhan, A. Lapeyrere, D. Logeart-Avramoglou and C. Picart, The stability of BMP loaded polyelectrolyte multilayer coatings on titanium, Biomaterials, 2013, 34(23), 5737–5746 CrossRef CAS PubMed.
- G. Cheng, C. Yin, H. Tu, S. Jiang, Q. Wang, X. Zhou, X. Xing, C. Xie, X. Shi, Y. Du and H. Deng, Controlled co-delivery of growth factors through layer-by-layer assembly of core–shell nanofibers for improving bone regeneration, ACS Nano, 2019, 13(6), 6372–6382 CrossRef CAS PubMed.
- M. N. Collins, G. Ren, K. Young, S. Pina, R. L. Reis and J. M. Oliveira, Scaffold fabrication technologies and structure/function properties in bone tissue engineering, Adv. Funct. Mater., 2021, 31(21), 2010609 CrossRef CAS.
- T. Crouzier, F. Sailhan, P. Becquart, R. Guillot, D. Logeart-Avramoglou and C. Picart, The performance of BMP-2 loaded TCP/HAP porous ceramics with a polyelectrolyte multilayer film coating, Biomaterials, 2011, 32(30), 7543–7554 CrossRef CAS PubMed.
- M. L. MacDonald, R. E. Samuel, N. J. Shah, R. F. Padera, Y. M. Beben and P. T. Hammond, Tissue integration of growth factor-eluting layer-by-layer polyelectrolyte multilayer coated implants, Biomaterials, 2011, 32(5), 1446–1453 CrossRef CAS PubMed.
- W. G. La, S. Park, H. H. Yoon, G. J. Jeong, T. J. Lee, S. H. Bhang, J. Y. Han, K. Char and B. S. Kim, Delivery of a therapeutic protein for bone regeneration from a substrate coated with graphene oxide, Small, 2013, 9(23), 4051–4060 CrossRef CAS PubMed.
- D. K. Lee, M. R. Ki, E. H. Kim, C. J. Park, J. J. Ryu, H. S. Jang, S. P. Pack, Y. K. Jo and S. H. Jun, Biosilicated collagen/β-tricalcium phosphate composites as a BMP-2-delivering bone–graft substitute for accelerated craniofacial bone regeneration, Biomater. Res., 2021, 25(1), 1–1 CrossRef PubMed.
- K. Chae, W. Y. Jang, K. Park, J. Lee, H. Kim, K. Lee, C. K. Lee, Y. Lee, S. H. Lee and J. Seo, Antibacterial infection and immune-evasive coating for orthopedic implants, Sci. Adv., 2020, 6(44), 1–15 Search PubMed.
- S. B. Goodman, Z. Yao, M. Keeney and F. Yang, The future of biologic coatings for orthopaedic implants, Biomaterials, 2013, 34(13), 3174–3183 CrossRef CAS PubMed.
- Q. Shi, Z. Qian, D. Liu and H. Liu, Surface modification of dental titanium implant by layer-by-layer electrostatic self-assembly, Front. Physiol., 2017, 8, 574 CrossRef PubMed.
- X. Zhang, Z. Li, X. Yuan, Z. Cui and X. Yang, Fabrication of dopamine-modified hyaluronic acid/chitosan multilayers on titanium alloy by layer-by-layer self-assembly for promoting osteoblast growth, Appl. Surf. Sci., 2013, 284, 732–737 CrossRef CAS.
- S. Choi, H. S. Jo, H. Song, H. J. Kim, J. K. Oh, J. W. Cho, K. Park and S. E. Kim, Multifunctional tannic acid-alendronate nanocomplexes with antioxidant, anti-inflammatory, and osteogenic potency, Nanomaterials, 2021, 11(7), 1812 CrossRef CAS PubMed.
- J. S. Moskowitz, M. R. Blaisse, R. E. Samuel, H. P. Hsu, M. B. Harris, S. D. Martin, J. C. Lee, M. Spector and P. T. Hammond, The effectiveness of the controlled release of gentamicin from polyelectrolyte multilayers in the treatment of Staphylococcus aureus infection in a rabbit bone model, Biomaterials, 2010, 31(23), 6019–6030 CrossRef CAS PubMed.
- J. Min, R. D. Braatz and P. T. Hammond, Tunable staged release of therapeutics from layer-by-layer coatings with clay interlayer barrier, Biomaterials, 2014, 35(8), 2507–2517 CrossRef CAS PubMed.
- A. J. Chung and M. F. Rubner, Methods of loading and releasing low molecular weight cationic molecules in weak polyelectrolyte multilayer films, Langmuir, 2002, 18(4), 1176–1183 CrossRef CAS.
-
G. Prieto, V. Domínguez-Arca, R. R. Costa, A. M. Carvalho, P. Taboada, R. L. Reis and I. Pashkuleva, Liposomes Embedded in Layer by Layer Constructs as Simplistic Exosome Transfer Model [Internet], in Review, 2020, [cited 2022 Feb 5]. Available from: https://www.researchsquare.com/article/rs-46696/v1.
- Y. Zha, Y. Li, T. Lin, J. Chen, S. Zhang and J. Wang, Progenitor cell-derived exosomes endowed with VEGF plasmids enhance osteogenic induction and vascular remodeling in large segmental bone defects, Theranostics, 2021, 11(1), 397 CrossRef CAS PubMed.
- Z. Wang, L. Dong, L. Han, K. Wang, X. Lu, L. Fang, S. Qu and C. W. Chan, Self-assembled Biodegradable Nanoparticles and Polysaccharides as Biomimetic ECM Nanostructures for the Synergistic effect of RGD and BMP-2 on Bone Formation, Sci. Rep., 2016, 6(1), 1–2 CrossRef PubMed.
- H. Zheng, Y. Tian, Q. Gao, Y. Yu, X. Xia, Z. Feng, F. Dong, X. Wu and L. Sui, Hierarchical micro-nano topography promotes cell adhesion and osteogenic differentiation via integrin α2-PI3K-AKT signaling axis, Front. Bioeng. Biotechnol., 2020, 8, 463 CrossRef PubMed.
- G. M. Cunniffe, C. M. Curtin, E. M. Thompson, G. R. Dickson and F. J. O'Brien, Content-dependent osteogenic response of nanohydroxyapatite: an in vitro and in vivo assessment within collagen-based scaffolds, ACS Appl. Mater. Interfaces, 2016, 8(36), 23477–23488 CrossRef CAS PubMed.
- L. Zhu, D. Luo and Y. Liu, Effect of the nano/microscale structure of biomaterial scaffolds on bone regeneration, Int. J. Oral Sci., 2020, 12(1), 1–5 Search PubMed.
- S. Yin, W. Zhang, Z. Zhang and X. Jiang, Recent advances in scaffold design and material for vascularized tissue–engineered bone regeneration, Adv. Healthcare Mater., 2019, 8(10), 1801433 CrossRef PubMed.
- T. Paulraj, N. Feoktistova, N. Velk, K. Uhlig, C. Duschl and D. Volodkin, Microporous Polymeric 3D Scaffolds Templated by the Layer–by–Layer Self–Assembly, Macromol. Rapid Commun., 2014, 35(16), 1408–1413 CrossRef CAS PubMed.
- J. Lee, S. Shanbhag and N. A. Kotov, Inverted colloidal crystals as three-dimensional microenvironments for cellular co-cultures, J. Mater. Chem., 2006, 16(35), 3558–3564 RSC.
- J. E. Nichols, J. Cortiella, J. Lee, J. A. Niles, M. Cuddihy, S. Wang, J. Bielitzki, A. Cantu, R. Mlcak, E. Valdivia and R. Yancy, In vitro analog of human bone marrow from 3D scaffolds with biomimetic inverted colloidal crystal geometry, Biomaterials, 2009, 30(6), 1071–1079 CrossRef CAS PubMed.
- M. A. Sahebalzamani, M. T. Khorasani and M. D. Joupari, Enhancement of fibroblasts outgrowth onto polycaprolactone nanofibrous grafted by laminin protein using carbon dioxide plasma treatment, Nano Biomed. Eng., 2017, 9(3), 191–198 CAS.
- E. Biazar, M. Ahmadian, A. Gazmeh, S. F. Mohammadi, A. Lashay, M. Heidari, H. Eslami, M. Sahebalzamani and H. Hashemi, Electro-spun polyethylene terephthalate (PET) mat as a keratoprosthesis skirt and its cellular study, Fibers Polym., 2017, 18(8), 1545–1553 CrossRef CAS.
- A. Ahmadian, A. Shafiee, N. Aliahmad and M. Agarwal, Overview of nano-fiber mats fabrication via electrospinning and morphology analysis, Textiles, 2021, 1(2), 206–226 CrossRef.
- J. Wang and C. Liu, Biomimetic collagen/hydroxyapatite composite scaffolds: Fabrication and characterizations, J. Bionic Eng., 2014, 11(4), 600–609 CrossRef.
- L. Li, Y. Zuo, Q. Zou, B. Yang, L. Lin, J. Li and Y. Li, Hierarchical structure and mechanical improvement of an n-HA/GCO–PU composite scaffold for bone regeneration, ACS Appl. Mater. Interfaces, 2015, 7(40), 22618–22629 CrossRef CAS PubMed.
- P. Jiang, Y. Zhang, R. Hu, X. Wang, Y. Lai, G. Rui and C. Lin, Hydroxyapatite-modified micro/nanostructured titania surfaces with different crystalline phases for osteoblast regulation, Bioact. Mater., 2021, 6(4), 1118–1129 CrossRef CAS PubMed.
- F. Song, W. Jie, T. Zhang, W. Li, Y. Jiang, L. Wan, W. Liu, X. Li and B. Liu, Room-temperature fabrication of a three-dimensional reduced-graphene oxide/polypyrrole/hydroxyapatite composite scaffold for bone tissue engineering, RSC Adv., 2016, 6(95), 92804–92812 RSC.
- M. F. Griffin, P. E. Butler, A. M. Seifalian and D. M. Kalaskar, Control of stem cell fate by engineering their micro and nanoenvironment, World J. Stem Cells, 2015, 7(1), 37 CrossRef PubMed.
- Y. Tang, Y. Zhao, X. Wang and T. Lin, Layer–by–layer assembly of silica nanoparticles on 3D fibrous scaffolds: Enhancement of osteoblast cell adhesion, proliferation, and differentiation, J. Biomed. Mater. Res., Part A, 2014, 102(11), 3803–3812 CrossRef PubMed.
- T. Gong, J. Xie, J. Liao, T. Zhang, S. Lin and Y. Lin, Nanomaterials and bone regeneration, Bone Res., 2015, 3(1), 1–7 Search PubMed.
- J. G. Lyons, M. A. Plantz, W. K. Hsu, E. L. Hsu and S. Minardi, Nanostructured biomaterials for bone regeneration, Front. Bioeng. Biotechnol., 2020, 922 CrossRef PubMed.
- M. M. Stevens, Biomaterials for bone tissue engineering, Mater. Today, 2008, 11(5), 18–25 CrossRef CAS.
- G. Wang, G. Qian, J. Zan, F. Qi, Z. Zhao, W. Yang, S. Peng and C. Shuai, A co-dispersion nanosystem of graphene oxide@ silicon-doped hydroxyapatite to improve scaffold properties, Mater. Des., 2021, 199, 109399 CrossRef CAS.
- E. Saito, E. E. Liao, W. W. Hu, P. H. Krebsbach and S. J. Hollister, Effects of designed PLLA and 50: 50 PLGA scaffold architectures on bone formation in vivo, J. Tissue Eng. Regener. Med., 2013, 7(2), 99–111 CrossRef CAS PubMed.
- M. Y. Zhao, L. H. Li, B. Li and C. R. Zhou, LBL coating of type I collagen and hyaluronic acid on aminolyzed PLLA to enhance the cell-material interaction, eXPRESS Polym. Lett., 2014, 8(5), 322–335 CrossRef.
- T. G. Kim, S. H. Park, H. J. Chung, D. Y. Yang and T. G. Park, Microstructured scaffold coated with hydroxyapatite/collagen nanocomposite multilayer for enhanced osteogenic induction of human mesenchymal stem cells, J. Mater. Chem., 2010, 20(40), 8927–8933 RSC.
- R. Donate, M. Monzón and M. E. Alemán-Domínguez, Additive manufacturing of PLA-based scaffolds intended for bone regeneration and strategies to improve their biological properties, e-Polym., 2020, 20(1), 571–599 CAS.
- N. J. Shah, M. N. Hyder, J. S. Moskowitz, M. A. Quadir, S. W. Morton, H. J. Seeherman, R. F. Padera, M. Spector and P. T. Hammond, Surface-mediated bone tissue morphogenesis from tunable nanolayered implant coatings, Sci. Transl. Med., 2013, 5(191), 1–24 Search PubMed.
- S. M. Best, A. E. Porter, E. S. Thian and J. Huang, Bioceramics: past, present and for the future, J. Eur. Ceram. Soc., 2008, 28(7), 1319–1327 CrossRef CAS.
- W. Bonfield, Designing porous scaffolds for tissue engineering, Philos. Trans. R. Soc., A, 2006, 364(1838), 227–232 CrossRef CAS PubMed.
- S. M. Romanelli, K. R. Fath, A. P. Phekoo, G. A. Knoll and I. A. Banerjee, Layer-by-layer assembly of peptide based bioorganic–inorganic hybrid scaffolds and their interactions with osteoblastic MC3T3-E1 cells, Mater. Sci. Eng., C, 2015, 51, 316–328 CrossRef CAS PubMed.
- S. Zhao, F. Caruso, L. Dähne, G. Decher, B. G. De Geest, J. Fan, N. Feliu, Y. Gogotsi, P. T. Hammond, M. C. Hersam and A. Khademhosseini, The future of layer-by-layer assembly: a tribute to ACS nano associate editor Helmuth Mohwald, ACS Nano, 2019, 13(6), 6151–6169 CrossRef CAS PubMed.
- Y. Tsukamoto, T. Akagi and M. Akashi, Vascularized cardiac tissue construction with orientation by layer-by-layer method and 3D printer, Sci. Rep., 2020, 10(1), 1–16 CrossRef PubMed.
- V. Guduric, C. Metz, R. Siadous, R. Bareille, R. Levato, E. Engel, J. C. Fricain, R. Devillard, O. Luzanin and S. Catros, Layer-by-layer bioassembly of cellularized polylactic acid porous membranes for bone tissue engineering, J. Mater. Sci.: Mater. Med., 2017, 28(5), 1–11 CrossRef CAS PubMed.
-
J. F Joanny and M. Castelnovo, Polyelectrolyte Adsorption and Multilayer Formation, in Multilayer Thin Films: Sequential Assembly of Nanocomposite Materials, Wiley-VCH Verlag GmbH & Co. KGaA, Weinheim, 2002, pp. 87–97.
Footnote |
† Joint first authors. |
|
This journal is © The Royal Society of Chemistry 2022 |