DOI:
10.1039/D2AY00300G
(Paper)
Anal. Methods, 2022,
14, 2090-2099
Development and application of an LC-MS method to the determination of poly- and perfluoroalkyl substances (PFASs) in drinking, sea and surface water samples†
Received
21st February 2022
, Accepted 9th May 2022
First published on 10th May 2022
Abstract
Poly- and perfluoroalkyl substances (PFASs) are a group of synthetic organic surfactants that have become a global concern because of their toxicity and widespread presence in the aquatic environment and organisms globally. In this study, a new analytical method has been developed and validated for the analysis of 15 perfluorinated compounds in different water matrices: river water, drinking water and seawater. Water extraction was performed in anion exchange solid phase extraction cartridges, and extracts were analysed by liquid chromatography in tandem with mass spectrometry. Recoveries for target analytes were between 35 and 120%, depending on the water matrix. Method detection limits were in the range of 0.5–17 ng L−1. The validated method was applied to the determination of perfluorinated compounds in water samples around Ireland. Eight compounds out of fifteen were detected at least in one sample. Measured concentrations were higher in river water than seawater, and drinking water had the lowest levels, although still detectable for a considerable amount of compounds. The most prevalent compounds were PFPeA, PFOA and PFHxA, present in all types of water, and they had the highest concentrations.
1. Introduction
Poly- and perfluoroalkyl substances (PFAS) are a class of thousands of anthropogenic substances that contain an aliphatic fluorinated carbon chain, and that have been in use since the 1950s.1 The C–F bond in the perfluoroalkyl moiety (–CnF2n+1, n > 1) is highly dipolar and relatively non-polarizable, which imparts the fluorinated compounds with polar hydrophobicity, repelling both water and oil.2 This has led to widespread industrial and commercial uses, such as construction, chemistry, food production, coatings and paints, surface repellents and textile production.3–5 As an example, more than 3000 tonnes of PFAS were used just in the Nordic countries.6 According to the OECD, more than 4700 PFAS compounds have been identified.7
Decades of extended global use of these highly persistent (they are often called “forever chemicals'') and mobile compounds have raised the concern about the potential impacts of legacy and new generation fluorinated alternatives on human health and the environment.8 PFAS can be bioaccumulated and biomagnified through the food chain.9–12 PFAS are ubiquitous in the aquatic environment and organisms globally, and have been detected in air, soil, plants and biota.13–15 Several studies on their toxicity have demonstrated that exposure to PFAS can result in neurotoxicity, hepatotoxicity, developmental toxicity, immunotoxicity, endocrine disruption and carcinogenicity.16,17
These studies had led to a great regulatory effort and scrutiny from national and international authorities. PFOS and derivatives have been restricted in Europe for more than 10 years, while PFOA has been banned under the POPs Regulation since 2020.18 Both PFOA and PFHxS are already included or are being considered to be included in the Stockholm Convention on Persistent Organic Pollutants. In Europe, the recast of the Drinking Water Directive, which took effect on 12 January 2021, includes a limit of 0.5 μg L−1 for all PFAS, and a limit value of 0.1 μg L−1 for each individual PFAS.19 Several EU Member States have set drinking water limits for specific PFAS and for groups of PFAS.20 PFOS and their derivatives are included as a priority hazardous substance under the EU Water Framework Directive,21 with a much lower Environmental Quality Standard (AA-EQS) limit value of 0.65 ng L−1 in inland surface waters and 0.13 ng L−1 in seawater, while a number of other PFAS are on the REACH list of Substances of Very High Concern (SVHCs).22 Moreover, several countries have proposed to ban the use of PFAS, except for essential uses under REACH, and it is anticipated that the proposal will be submitted to the European Chemical Agency by 15 July 2022.
As a consequence of these regulatory efforts, global manufacturers have started to produce new chemicals in order to substitute long-chain PFAS, such as shorter-chain compounds or with non-fluorinated substances. However, some of these new chemicals have resulted to be equally environmentally persistent and even more mobile in the environment and more difficult to remove from drinking water than their long-chain counterparts, and some have resulted to be toxic as well (e.g. GenX).23,24 In particular, short-chain PFAS accumulate in the environment and have been found to contaminate surface, ground- and drinking water.25,26
Therefore, there is an urgent need for continuous monitoring of PFAS in aquatic systems, with special emphasis in drinking water, due to their possible negative impact on human health. In this context, the main objectives of this work were (i) to develop and validate an analytical methodology applicable to drinking water, surface water and seawater; and (ii) to assess the occurrence of 15 PFAS in drinking water in the Dublin area, river water from 4 Irish rivers and seawater from Dublin Bay, with a total of 12 samples analysed. The analytical methodology presented here is an advance for the screening of PFAS in the water cycle in Ireland, and the study of the occurrence of these chemicals will be essential for the implementation of future concentration limits by the regulatory authorities for environmental and human protection.
2. Materials & methods
2.1. Chemicals and solutions
PFAS substances included in this study were supplied by Wellington Laboratories Inc. (Canda) including: the acids perfluoropentanoic (PFPeA), perfluorohexanoic (PFHxA), perfluorooctanoic (PFOA), perfluorononanoic (PFNA), perfluorodecanoic (PFDA), perfluoroundecanoic (PFUdA), perfluorododecanoic (PFDoA), perfluoropropoxypropanoic (GenX), and perfluorobutanesulfonate (PFBS), perfluorooctanesulfonate (PFOS), perfluorodecanesulfonate (PFDS), perfluoropentylsulfonate (PFPeS), perfluorohexasulfonate (PFHxS), perfluorooctanesulfonamide (FOSA), perfluorononylsulfonate (PFNS). Internal standards (added before analysis in order to assess and compensate possible losses during sample manipulation and extraction) were purchased from Wellington Laboratories Inc. (Canada) including: [13C8]-perfluorooctanesulfonate (M8PFOS), [13C2]-perfluorooctanoic acid (M2PFOA), [13C5]-perfluorononanoic acid (MPFNA), and [13C2]-perfluorohexanoic acid (MPFHxA).
The list of the target analytes, including class, formula and acronyms are listed in Table 1. Individual stock standards and labelled internal standards were prepared in methanol at a concentration of approximately 1000 mg L−1. Stock solutions and 50 mg L−1 mixtures in methanol were stored at −20 °C and diluted to 1 mg L−1 before each analytical run. Ultrapure water was prepared by a Purelab® Ultra water purification system. Methanol (MeOH) LC-MS grade was obtained from Honeywell (France). Ammonium acetate salt (NH4Ac), ammonia (NH4OH), sodium thiosulfate (Na2S2O3), acetic acid and acetonitrile (ACN) were obtained from Sigma-Aldrich (Dublin, Ireland).
Table 1 Description of target analytes included in the present study
Compound |
Acronym |
CAS number |
Class |
Fluorinated C chain |
Formula |
Perfluorododecanoate |
PFDoA |
307-55-1 |
Acid |
C12 |
C12HF23O2 |
Perfluoroundecanoate |
PFUdA |
2058-94-8 |
Acid |
C11 |
C11HF21O2 |
Perfluorodecanoate |
PFDA |
335-76-2 |
Acid |
C10 |
C10HF19O2 |
Perfluorononanoate |
PFNA |
375-95-1 |
Acid |
C9 |
C9HF17O2 |
Perfluorooctanoate |
PFOA |
335-67-1 |
Acid |
C8 |
C8HF15O2 |
Perfluorohexanoate |
PFHxA |
307-24-4 |
Acid |
C6 |
C6HF11O2 |
Perfluoropropoxypropanoic acid |
Gen X |
13252-13-6 |
Acid |
C6 |
C6HF11O3 |
Perfluoropentanoate |
PFPeA |
2706-90-3 |
Acid |
C5 |
C5HF9O2 |
Perfluorooctanesulfonamide |
FOSA |
754-91-6 |
FOSA |
C8 |
C8H2F17NO2S |
Perfluorodecylsulfonate |
PFDS |
2806-15-7 |
Sulfonate |
C10 |
C10HF21O3S |
Perfluorononylsulfonate |
PFNS |
98789-57-2 |
Sulfonate |
C9 |
C9HF19O3S |
Perfluorooctylsulfonate |
PFOS |
4021-47-0 |
Sulfonate |
C8 |
C8HF17O3S |
Perfluorohexylsulfonate |
PFHxS |
82382-12-5 |
Sulfonate |
C6 |
C6HF13O3S |
Perfluoropentylsulfonate |
PFPeS |
630402-22-1 |
Sulfonate |
C5 |
C7HF13O2 |
Perfluorobutylsulfonate |
PFBS |
29420-49-3 |
Sulfonate |
C4 |
C4HF9O3S |
Perfluoro-1-(13C8) octanesulfonate |
M8PFOS |
— |
Sulfonate |
C8 |
13C8F17SO3 |
Perfluoro-n-[1,2,3,4,5-13C5] nonanoic acid |
MPFNA |
— |
Acid |
C9 |
13C512C4HF17O2 |
Perfluoro-n-[1,2-13C2] octanoic acid |
MPFOA |
— |
Acid |
C8 |
13C212C6HF15O2 |
Perfluoro-n-[1,2-13C2] hexanoic acid |
MPFHxA |
— |
Acid |
C6 |
13C212C4HF11O2 |
2.2. Sample collection and extraction
The study was conducted in different types of water: (i) drinking water: samples were collected in 4 households in Dublin city; (ii) seawater: samples were collected in from 3 sites in the Dublin Bay area; (iii) surface water: samples were collected from 4 river sections around the western part of Ireland (Rivers Annalee, Liffey, Suir, Nore). Fig. 1 represents the locations where samples were collected during this study.
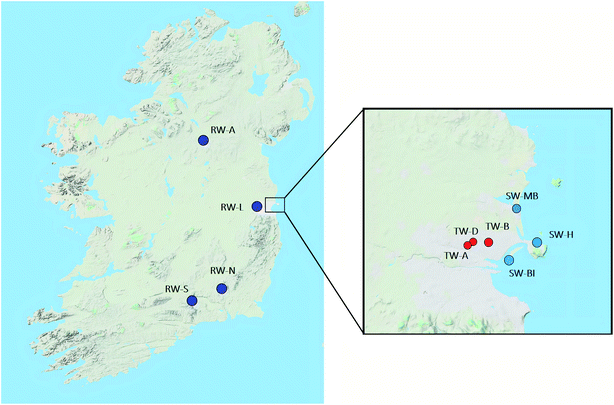 |
| Fig. 1 Samples sites in Ireland. RW: river water. TW: drinking water. SW: seawater. | |
Water samples were collected in amber polypropylene bottles, previously washed with methanol and rinsed with distilled water, and taken to the laboratory, where pH was adjusted to 3 with acetic acid, and they were kept at 4 °C until extraction if extracted within 24 h; otherwise, they were frozen until analysis. To reduce residual chlorine, 200 μL of 250 mg mL−1 solution in water of sodium thiosulfate was added to seawater samples.27 Two types of SPE cartridges (Oasis WAX Cartridges and Oasis HLB, both 500 mg) were conditioned with 5 mL of 0.5% ammonia/methanol (only WAX cartridges), followed by 5 mL of methanol and 5 mL of ultra-pure water (pH 3). Samples (250 mL for river water, 1 L for drinking and marine water) were loaded into the cartridge at a flow rate of 2 mL min−1. Cartridges were rinsed with 5 mL of ultra-pure water, and were dried with air for 30 min. Analytes were eluted with 5 mL of methanol followed by 5 mL of 0.5% ammonia/methanol. Eluates were placed under a N2 current until dryness, and then reconstituted in 1 mL methanol/water (20
:
80). Finally, 5 μL of a 1 mg L−1 standard mixture containing labelled compounds were added to the extracts as internal standards.
2.3. Instrumental analysis
All extracts were analysed by HPLC (Agilent 1260 series Infinity binary pump) coupled to a triple quadrupole mass spectrometer (Agilent 6470), equipped with an electrospray ionization source in negative mode. Ten microliters were injected in an Agilent Zorbax Eclipse Plus C18 column (3.0 × 50 mm; 1.8 μm), set with a guard column of the same materials. Mobile phases were (A): 2
mM formic acid/ammonium formate (pH 3.2) and (B): methanol at a flow rate of 0.4
mL min−1. The gradient elution was: 0–1.5 min, 90–45% A; 1.5–7.0 min, 45–10% A; 7.0–8.5 min, 10–0% A; 8.5–10 min, 0% A; 10–11 min return to initial conditions; 11–12 min, equilibration of the column. Column temperature was set at 35 °C. Representative standard chromatogram is shown in Fig. 2.
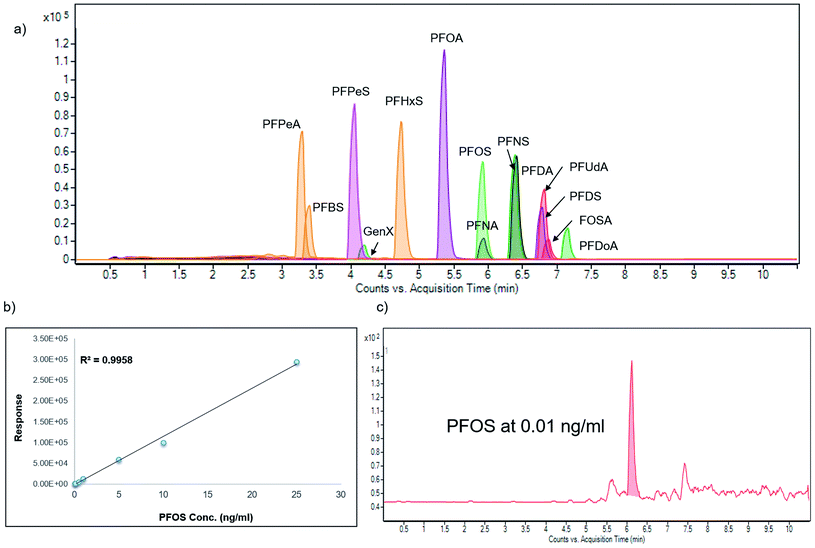 |
| Fig. 2 (a) Compound peaks obtained by injecting a standard mixture at 100 ng mL−1; (b) PFOS calibration curve (levels 0.01 to 25 ng mL−1), with an R2 > 0.99; (c) PFOS chromatogram extracted at a concentration of 0.01 ng mL−1. | |
Compound-dependent mass spectrometric parameters (fragmentor, collision energy) as well as compound-selected reaction monitoring transitions were optimized by direct infusion of individual standard solution of each analyte at 10
ng mL−1 using the Agilent Compound Optimizer software. A summary of these parameters is presented in Table 2. Source-dependent parameters were determined by flow injection analysis: gas temperature: 230 °C; gas flow: 4 L min−1; nebulizer: 15 psi; source temperature, 375
°C; ion spray voltage, 2500; sheath gas flow: 12 L min−1. In the cases where two transitions were monitored, the most intense one was used for quantification purposes, whereas the second one was used to confirm the identity of the target compounds. Instrument control data acquisition and data analysis were carried out using Agilent software.
Table 2 MRM transitions and compound dependant MS parameters for target analytes. For the product ion, second transition was placed between parenthesis. RT: retention time
Compound |
Precursor ion |
Product ion |
RT (min) |
Fragmentor |
Collision energy (V) |
PFDoA |
613 |
519 (219) |
7.2 |
112 |
8 (20) |
PFUdA |
563 |
519 (219) |
6.9 |
112 |
8 (20) |
PFDA |
513 |
469 (269) |
6.6 |
112 |
8 (12) |
PFNA |
463 |
219 (419) |
6.1 |
78 |
16 (8) |
PFOA |
413 |
369 (169) |
5.5 |
78 |
8 (12) |
PFHxA |
313 |
269 (91) |
4.1 |
78 |
4 (8) |
Gen X |
285 |
185 (169) |
4.2 |
78 |
16 (4) |
PFPeA |
263 |
219 |
3.3 |
78 |
4 |
FOSA |
498 |
78 |
7.0 |
180 |
36 |
PFDS |
599 |
80 (99) |
6.9 |
180 |
60 (56) |
PFNS |
549 |
80 (99) |
6.5 |
180 |
56 |
PFOS |
499 |
80 (99) |
6.1 |
180 |
44 |
PFHxS |
399 |
80 (99) |
4.9 |
180 |
44 (40) |
PFPeS |
349 |
80 (99) |
4.1 |
180 |
40 (36) |
PFBS |
299 |
80 (99) |
3.4 |
146 |
40 (36) |
M8PFOS |
507 |
80 |
6.1 |
180 |
52 |
MPFNA |
468 |
219 |
6.1 |
78 |
16 |
MPFOA |
415 |
370 |
5.5 |
78 |
8 |
MPFHxA |
315 |
270 |
4.1 |
78 |
4 |
2.4. Validation
Both MDL and MQL were determined in spiked water samples (n = 3) of all matrices considered as the minimum detectable amount of analyte with a signal-to-noise ratio of 3 and 10, respectively. Precision was calculated from five repeated injections of a spiked sample at 1, 50, and 500 ng mL−1, and was expressed as the relative standard deviation of the measured concentration. Calibration curves were generated using linear regression analysis (R2 > 0.99) in the concentration range from 0.01 to 1000 ng mL−1. To determine the extraction efficiencies, water samples (drinking, surface and marine water) were spiked with a mixture of the target analytes at 100 ng mL−1. Concentrations obtained after the extraction procedure, calculated by internal standard calibration, were compared with the initial spiking levels.
2.5. Background contamination
Careful measures were taken to prevent background levels of PFAS arising from sample manipulation and instrumental analysis. All materials that could potentially contain the target analytes were avoided (Teflon bottles, materials used during SPE, HPLC vials, etc.). Polypropylene was used when possible instead of glass to avoid compound retention on the containers. In order to eliminate the sources of contamination from the analytical system all the polytetrafluoroethylene (PTFE) tubing was changed for polyether ether ketone (PEEK) connections.
Procedural blanks were used during sample collection, extraction and treatment prior to instrumental analysis. Ultra-pure water was used with this purpose for optimization and validation, and these samples were analysed to assess the initial concentrations of PFAS. For sample collection, potential PFAS concentration on empty polypropylene bottles was examined by adding 10 mL of methanol and shaking for 10 min. The extract was pre-concentrated to 50 μL and analysed. SPE cartridges were tested for the presence of PFAS through the entire analytical procedure. Cartridges were preconditioned and eluted with 0.5% ammonia/methanol as described in Section 2.2, except no samples were loaded. Solvent blanks (methanol, methanol/water) were also analysed continuously during each run to check for background PFAS levels. Instrumental blanks consisting of initial conditions of the mobile phase were analysed every 5 sample injections to check for carryover and residual PFAS concentration.
3. Results & discussion
3.1. Method validation
Oasis WAX cartridges were selected for sample extraction, as recoveries were found to be generally higher, and the results were more replicable than with Oasis HLB (see Fig. 3).
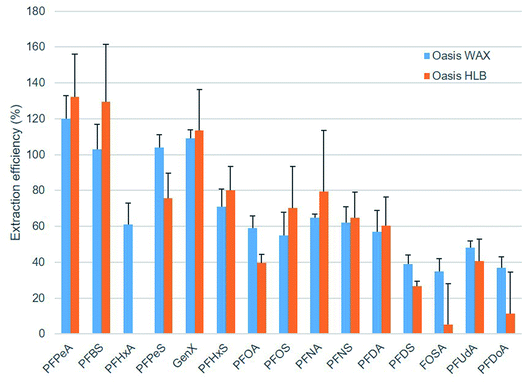 |
| Fig. 3 Extraction efficiencies (%) obtained with two types of SPE cartridges (WAX and HLB) when samples were spiked at 100 ng L−1 (n = 3). | |
Extraction recoveries, method detection and quantification limits were calculated for each target analyte and water matrix (Table 3). Extraction efficiency for all compounds was above 50%, except for PFDS, FOSA, PFUdA and PFoDA (35–48%). Method detection limits (MDLs) were in the range of 0.0.46–12.62 ng L−1 for drinking water, 0.58–15.15 ng L−1 for river water, and 0.65–17.7 ng L−1 in seawater.
Table 3 Mean percent recoveries (n = 3) of 15 PFASs in the three water matrices, and method detection and quantification limits (MDL, MQL) in each water matrix (ng L−1)
|
Recoveries (±RSD) |
MLD (ng L−1) |
MLQ (ng L−1) |
TW |
SW |
RW |
TW |
SW |
RW |
TW |
SW |
RW |
PFPeA |
120 (13) |
98 (12) |
101 (10) |
1.40 |
1.96 |
1.68 |
4.67 |
6.54 |
5.61 |
PFBS |
103 (4) |
96 (9) |
99 (12) |
0.48 |
0.67 |
0.58 |
1.60 |
2.24 |
1.92 |
PFHxA |
61 (12) |
57 (9) |
59 (3) |
1.78 |
2.49 |
2.13 |
5.92 |
8.29 |
7.11 |
PFPeS |
104 (7) |
105 (12) |
102 (4) |
0.75 |
1.05 |
0.90 |
2.49 |
3.48 |
2.99 |
GenX |
109 (5) |
103 (2) |
105 (2) |
1.45 |
2.03 |
1.74 |
4.82 |
6.75 |
5.79 |
PFHxS |
71 (10) |
60 (13) |
67 (3) |
0.46 |
0.65 |
0.55 |
1.54 |
2.16 |
1.85 |
PFOA |
59 (7) |
51 (5) |
55 (7) |
1.27 |
1.78 |
1.53 |
4.24 |
5.93 |
5.09 |
PFOS |
55 (13) |
59 (9) |
55 (12) |
0.91 |
1.28 |
1.10 |
3.04 |
4.26 |
3.65 |
PFNA |
65 (2) |
67 (12) |
63 (14) |
0.81 |
1.14 |
0.98 |
2.71 |
3.80 |
3.26 |
PFNS |
62 (9) |
58 (3) |
61 (12) |
0.66 |
0.93 |
0.80 |
2.21 |
3.09 |
2.65 |
PFDA |
57 (12) |
55 (9) |
59 (10) |
0.48 |
0.67 |
0.58 |
1.61 |
2.25 |
1.93 |
PFDS |
39 (5) |
35 (3) |
41 (3) |
2.43 |
3.40 |
2.91 |
8.09 |
11.32 |
9.70 |
FOSA |
35 (7) |
39 (4) |
41 (6) |
12.62 |
17.67 |
15.15 |
42.07 |
58.90 |
50.48 |
PFUdA |
48 (4) |
45 (7) |
51 (6) |
0.91 |
1.27 |
1.09 |
3.02 |
4.23 |
3.62 |
PFDoA |
37 (6) |
35 (8) |
39 (7) |
4.11 |
5.75 |
4.93 |
13.69 |
19.17 |
16.43 |
Calibration curves for all compounds prepared in solvent were generated using linear regression analysis (R2 > 0.99) in the range from 0.01 ng mL−1 and 1000 ng mL−1 (Fig. S1†). Instrument accuracy was calculated as the deviation of the measured mean concentration from the real concentration, expressed in percentage from 5 repeated injections of a spiked sample at three different levels (1, 50, 500 ng mL−1). Repeated injections were done during the same day (intra-day accuracy) or during 5 consecutive days (inter-day accuracy). At the lowest spiking concentration, values were less than 12.5%, intra-day and 17.5%, inter-day (Table 4).
Table 4 Intra-day and inter-day accuracy (expressed as % RSD) calculated at three spiking levels for 15 PFAS
|
Accuracy (% RSD) |
Intra-day |
Inter-day |
1 ng mL−1 |
50 ng mL−1 |
500 ng mL−1 |
1 ng mL−1 |
50 ng mL−1 |
500 ng mL−1 |
PFPeA |
1.4 |
0.9 |
2.4 |
17.5 |
2.4 |
10.9 |
PFBS |
6.9 |
0.9 |
1.9 |
0.5 |
4.6 |
0.8 |
PFHxA |
2.9 |
15.5 |
6.7 |
1.7 |
2.6 |
5.5 |
PFPeS |
2.0 |
1.5 |
1.5 |
0.8 |
1.3 |
7.3 |
GenX |
12.4 |
1.8 |
1.5 |
7.2 |
5.1 |
0.0 |
PFHxS |
0.7 |
1.2 |
4.3 |
1.2 |
0.8 |
7.7 |
PFOA |
0.7 |
1.2 |
4.3 |
4.2 |
2.2 |
9.4 |
PFOS |
4.0 |
1.0 |
2.2 |
1.7 |
1.7 |
2.7 |
PFNA |
2.1 |
0.4 |
1.1 |
2.2 |
0.9 |
11.1 |
PFNS |
5.1 |
2.4 |
10.2 |
3.0 |
1.7 |
11.0 |
PFDA |
1.2 |
0.4 |
10.3 |
2.5 |
1.0 |
2.0 |
PFDS |
2.9 |
2.5 |
1.3 |
3.7 |
3.5 |
3.8 |
FOSA |
2.6 |
0.4 |
1.1 |
9.2 |
10.0 |
7.6 |
PFUdA |
11.7 |
1.3 |
2.5 |
5.4 |
5.0 |
10.4 |
PFDoA |
5.3 |
1.3 |
2.2 |
3.1 |
2.2 |
7.5 |
3.2. Comparison of the proposed method with previously published methods
The analytical performance of the present method was compared with other published analytical methods for the determination of PFAS in water samples. Table 5 presents the characteristic data of the present method and the other techniques reported in literature. The method reported here presents comparable MDLs and recoveries to similar methods, in some cases reporting better MDLs and recoveries.
Table 5 Comparison of the present method with other analytical methods for the determination of PFAS in water samples
Reference |
Compounds |
Extraction |
Detection |
Sample |
Linearity (R2) |
MDLs (ng L−1) |
Recovery (%) |
Accuracya |
Intra-day precision at the lowest spiking level.
|
Present method |
15 |
SPE |
HPLC MS/MS |
Tap, river, seawater |
>0.99 |
0.5–17.7 |
35–120 |
1–12% |
(Liang et al., 2020)28 |
21 |
SUPRAS-based microextraction |
HPLC-Orbitrap |
Tap, river |
>0.99 |
10–80 |
72–118 |
1–11% |
(Pico et al., 2012)29 |
10 |
SPE |
HPLC-MS/MS |
Surface water |
>0.99 |
0.02–1.5 |
55–85 |
Not reported |
(Gebbink et al., 2017)26 |
14 |
SPE |
UPLC-MS/MS |
Tap, river |
>0.99 |
0.01–4 |
81–115 |
Not reported |
(Ali et al., 2021)30 |
13 |
SPE |
HPLC MS/MS |
Seawater |
>0.99 |
0.04–4.1 |
17–117 |
Up to 10% |
3.3. Background contamination
Procedural blanks (ultra-pure water extracted following the same procedure as the samples) were analysed to assess the initial concentrations of PFAS, and in all cases were below the MQLs. Similarly, the residual concentrations analysed from empty polypropylene bottles was considered negligible (results not shown). SPE cartridge blanks (no sample loaded) were tested for the presence of PFAS through the entire analytical procedure, with no levels detected above the MQLs for any compound. Instrumental blanks (methanol/water) were analysed every 5 sample injections, and no peaks were detected above the MDLs.
3.4. Environmental samples
A total of 12 water samples were analysed for method validation purposes, including a procedural blank (TW-C) and a solvent blank (TW-M). Results obtained are shown in Table 6 (data adjusted with recoveries). Fig. 4 shows the relative quantities of target analytes in each water sample. Out of the 15 compounds in the developed method, 8 were detected at least once in river water (Fig. S2†). These samples contained the highest PFAS concentrations, with a maximum detected of 424 ng L−1 for PFPeA, followed by PFOA (approx 200 ng L−1), similarly to other studies carried out in surface waters, where PFAS levels have been found in the low ng L−1 range in rivers of urban catchments.31–33 In seawater samples, 7 compounds were detected, but only 3 were above MQLs. The highest concentrations were around 45 and 13 ng L−1 for the compounds PFBS and PFPeA, respectively, an order of magnitude lower than in river water, comparable to similar studies in ocean water. However, some studies reported concentrations at several orders of magnitude greater in highly polluted areas.27,34,35 In drinking water samples, only 5 compounds were detected with values > MQLs, in the range of 4–7 ng L−1, with very few exceptions. The most prevalent compounds were PFPeA and PFHxA, present in all types of water, and they had the highest concentrations. Literature shows that drinking water PFAS levels are usually low (pg L−1 range), but some studies have found them up to the μg L−1 level.36,37 As expected, concentrations were much higher in river water than seawater, and drinking water had the lowest levels, although still detectable for a considerable amount of compounds. This screening provides a valuable snapshot of what the situation is in Irish waters, but a more extensive monitoring campaign is required to understand the impact of seasonality and land use on the occurrence of these compounds in water systems.
Table 6 Concentration (ng L−1) of detected PFAS in environmental samples. BLK 1 represents the average results of the procedural blanks (ultra-pure water, n = 3). BLK 2 represents the SPE cartridge blank average results (n = 3). TW: drinking water. SW: seawater. RW: river water
|
Matrix |
PFPeA |
PFBS |
PFHxA |
GenX |
PFOA |
PFNA |
PFDA |
PFUdA |
BLK 1 |
Blank H2O |
<MLQ |
<MLQ |
<MLQ |
<MQL |
<MLQ |
<MLQ |
<MLQ |
<MDL |
BLK 2 |
Blank SPE |
<MLD |
<MDL |
<MDL |
<MQL |
<MDL |
<MQL |
<MQL |
<MDL |
TW-A |
Drinking water |
<MLQ |
<MDL |
<MDL |
<MQL |
5.3 |
<MQL |
<MQL |
<MDL |
TW-B |
Drinking water |
<MLQ |
<MDL |
10.7 |
<MQL |
7.3 |
<MQL |
<MQL |
<MDL |
TW-D |
Drinking water |
5.3 |
4.1 |
6.6 |
15.6 |
6.8 |
<MQL |
<MQL |
<MDL |
SW-MB |
Seawater |
<MLQ |
<MQL |
<MQL |
<MQL |
<MQL |
<MQL |
<MQL |
<MDL |
SW-H |
Seawater |
6.7 |
34.0 |
<MQL |
<MQL |
<MQL |
<MDL |
<MQL |
<MDL |
SW-BI |
Seawater |
13.5 |
45.3 |
7.0 |
<MQL |
<MQL |
<MQL |
<MQL |
<MDL |
RW-N |
River water |
301.2 |
49.5 |
134.6 |
39.1 |
172.9 |
43.0 |
22.2 |
6.9 |
RW-S |
River water |
424.1 |
68.9 |
173.3 |
44.7 |
205.4 |
46.5 |
24.1 |
5.5 |
RW-L |
River water |
250.2 |
64.0 |
122.7 |
33.4 |
138.2 |
30.8 |
15.8 |
6.9 |
RW-A |
River water |
269.5 |
35.2 |
132.6 |
32.0 |
197.5 |
56.4 |
23.7 |
8.8 |
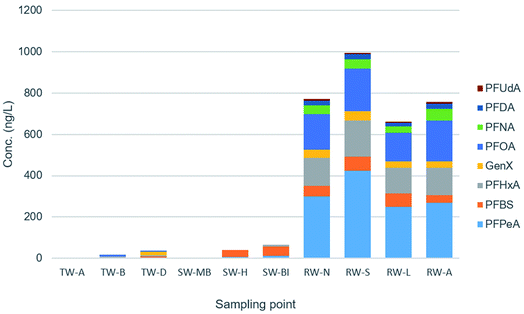 |
| Fig. 4 Concentration (ng L−1) of detected pfas in environmental samples. TW: drinking water. SW: seawater. RW: river water. | |
According to European legislation,18 levels of PFOS should be lower than 0.65 ng L−1 in surface water and lower than 0.13 ng L−1 in seawater. In this study, PFOS was not detected in any of the samples analysed. Quantification of PFAS in seawater at the required protection level and associated performance criteria for marine waters is particularly challenging. The detection limits achieved in this method (e.g., for PFOS in seawater 1.28 ng L−1) would allow for the method to be applied for ultra-trace screening of marine waters. For drinking water, the addition of all concentrations reached a maximum of 65 ng L−1, well below the 500 ng L−1 limit by the Drinking Water Directive.21 More concerning is the occurrence of GenX in surface waters at concentrations around 40 ng L−1. Although GenX is not currently regulated, this compound has demonstrated to be equally toxic than some of the legacy PFAS,24,38 and the US EPA has calculated that chronic toxicity for human consumption could be ten times greater than PFOS.39
4. Conclusions
While there are several LC-MS/MS based analytical methods for PFAS analysis reported in the literature,13,26,27,36,40 reliable analytical methods for trace determination of legacy and emerging PFAS in varied media, suitable for routine environmental risk assessment, is highly desirable. This study developed a simple, rapid, and efficient analytical method for the determination of 15 PFASs, including legacy and novel compounds, some of them heavily regulated, in different types of water: seawater, river and drinking water. The developed method allows the identification of short and long chain compounds, which in many cases it is linked to the type of application/source of each compound. The final procedure consisted of pre-concentration of water samples with anionic exchange solid-phase extraction, and the analysis was performed by HPLC-MS/MS, which provided the necessary sensitivity and selectivity for these contaminants. The method was applied to assess the occurrence of these contaminants in Ireland. The assessment of PFAS in real samples showed that PFPeA, PFOA, PFBS, and PFHxA were present in all types of water, and they occurred at the highest concentrations in all matrices. The highest concentrations were found in surface river waters, with a maximum value detected of 424 ng L−1 for PFPeA in the River Suir (Ireland). For drinking water, the levels detected were below the limits stated by the Drinking Water Directive.19
Conflicts of interest
There are no conflicts to declare.
Acknowledgements
This research (Grant aid agreement No PDOC/19/03/02) is carried out with the support of the Marine Institute, funded under the Marine Research Programme by the Irish Government.
References
- R. C. Buck, J. Franklin, U. Berger, J. M. Conder, I. T. Cousins and P. de Voogt,
et al., Perfluoroalkyl and polyfluoroalkyl substances in the environment: Terminology, classification, and origins, Integr. Environ. Assess. Manage., 2011, 7(4), 513–541 CrossRef CAS PubMed
.
- J. C. Biffinger, H. W. Kim and S. G. DiMagno, The Polar Hydrophobicity of Fluorinated Compounds, ChemBioChem, 2004, 5(5), 622–627 CrossRef CAS PubMed
.
- X. C. Hu, D. Q. Andrews, A. B. Lindstrom, T. A. Bruton, L. A. Schaider and P. Grandjean,
et al., Detection of Poly- and Perfluoroalkyl Substances (PFASs) in U.S. Drinking Water Linked to Industrial Sites, Military Fire Training Areas, and Wastewater Treatment Plants, Environ. Sci. Technol. Lett., 2016, 3(10), 344–350 CrossRef CAS PubMed
.
- R. Dhore and G. S. Murthy, Per/polyfluoroalkyl substances production, applications and environmental impacts, Bioresour. Technol., 2021, 341, 125808 CrossRef CAS PubMed
.
- U. Eriksson, P. Haglund and A. Kärrman, Contribution of precursor compounds to the release of per- and polyfluoroalkyl substances (PFASs) from waste water treatment plants (WWTPs), J. Environ. Sci., 2017, 61, 80–90 CrossRef CAS PubMed
.
- J. Glüge, M. Scheringer, I. T. Cousins, J. C. DeWitt, G. Goldenman and D. Herzke,
et al., An overview of the uses of per- and polyfluoroalkyl substances (PFAS), Environ. Sci.: Processes Impacts, 2020, 22(12), 2345–2373 RSC
.
-
OECD, Towards a New Comprehensive Global Database of Per- and Polyfluoroalkyl Substances (PFASs), 2018 Search PubMed
.
- M. Scheringer, X. Trier, I. T. Cousins, P. de Voogt, T. Fletcher and Z. Wang,
et al., Helsingør Statement on poly- and perfluorinated alkyl substances (PFASs), Chemosphere, 2014, 114, 337–339 CrossRef CAS PubMed
.
- D. A. Miranda, J. P. Benskin, R. Awad, G. Lepoint, J. Leonel and V. Hatje, Bioaccumulation of Per- and polyfluoroalkyl substances (PFASs) in a tropical estuarine food web, Sci. Total Environ., 2021 Feb, 754, 142146 Search PubMed
.
- L. P. Burkhard, Evaluation of Published Bioconcentration Factor (BCF) and Bioaccumulation Factor (BAF) Data for Per- and Polyfluoroalkyl Substances Across Aquatic Species, Environ. Toxicol. Chem., 2021, 40(6), 1530–1543 CrossRef CAS PubMed
.
- D. J. Beale, S. Nilsson, U. Bose, N. Bourne, S. Stockwell and J. A. Broadbent,
et al., Bioaccumulation and impact of maternal PFAS offloading on egg biochemistry from wild-caught freshwater turtles (Emydura macquarii macquarii), Sci. Total Environ., 2022, 817, 153019 CrossRef CAS PubMed
.
- A. Androulakakis, N. Alygizakis, G. Gkotsis, M. C. Nika, V. Nikolopoulou and E. Bizani,
et al., Determination of 56 per- and polyfluoroalkyl substances in top predators and their prey from Northern Europe by LC-MS/MS, Chemosphere, 2022, 287, 131775 CrossRef CAS PubMed
.
- K. Kannan, L. Tao, E. Sinclair, S. D. Pastva, D. J. Jude and J. P. Giesy, Perfluorinated Compounds in Aquatic Organisms at Various Trophic Levels in a Great Lakes Food Chain, Arch. Environ. Contam. Toxicol., 2005, 48(4), 559–566 CrossRef CAS PubMed
.
- C. M. Butt, U. Berger, R. Bossi and G. T. Tomy, Levels and trends of poly- and perfluorinated compounds in the arctic environment, Sci. Total Environ., 2010, 408(15), 2936–2965 CrossRef CAS PubMed
.
- N. H. Lam, C. R. Cho, J. S. Lee, H. Y. Soh, B. C. Lee and J. A. Lee,
et al., Perfluorinated alkyl substances in water, sediment, plankton and fish from Korean rivers and lakes: A nationwide survey, Sci. Total Environ., 2014, 491–492, 154–162 CrossRef CAS PubMed
.
- Y. Cao and C. Ng, Absorption, distribution, and toxicity of per- and polyfluoroalkyl substances (PFAS) in the brain: a review, Environ. Sci.: Processes Impacts, 2021, 23(11), 1623–1640 RSC
.
- A. O. De Silva, J. M. Armitage, T. A. Bruton, C. Dassuncao, W. Heiger-Bernays and X. C. Hu,
et al., PFAS Exposure Pathways for Humans
and Wildlife: A Synthesis of Current Knowledge and Key Gaps in Understanding, Environ. Toxicol. Chem., 2021, 40(3), 631–657 CrossRef CAS PubMed
.
-
European Commission, Regulation (EU) 2019/1021 of the European Parliament and of the Council of 20 June 2019 on persistent organic pollutants (recast), 2019, available from: http://data.europa.eu/eli/reg/2019/1021/2021-03-15.
-
European Commission, Directive (EU) 2020/2184 of the European Parliament and of the Council of 16 December 2020 on the quality of water intended for human consumption, 2020, available from: https://eur-lex.europa.eu/eli/dir/2020/2184/oj.
- X. Dauchy, Per- and polyfluoroalkyl substances (PFASs) in drinking water: Current state of the science, Curr. Opin. Environ. Sci. Health, 2019, 7, 8–12 CrossRef
.
-
European Commission, Directive 2013/39/EU of the European Parliament and of the Council of 12 August 2013 amending Directives 2000/60/EC and 2008/105/EC as regards priority substances in the field of water policy, 2013, available from: https://eur-lex.europa.eu/eli/dir/2013/39/oj.
-
European Commission, Regulation (EC) No 1907/2006 of the European Parliament and of the Council of 18 December 2006 concerning the Registration, Evaluation, Authorisation and Restriction of Chemicals (REACH), establishing a European Chemicals Agency, 2006, available from: https://eur-lex.europa.eu/legal-content/EN/TXT/?uri=CELEX%3A02006R1907-20140410.
- R. E. Cannon, A. C. Richards, A. W. Trexler, C. T. Juberg, B. Sinha and G. A. Knudsen,
et al., Effect of GenX on P-Glycoprotein, Breast Cancer Resistance Protein, and Multidrug Resistance–Associated Protein 2 at the Blood–Brain Barrier, Environ. Health Perspect., 2020, 128(3), 037002 CrossRef CAS PubMed
.
- J. M. Conley, C. S. Lambright, N. Evans, J. McCord, M. J. Strynar and D. Hill,
et al., Hexafluoropropylene oxide-dimer acid (HFPO-DA or GenX) alters maternal and fetal glucose and lipid metabolism and produces neonatal mortality, low birthweight, and hepatomegaly in the Sprague-Dawley rat, Environ. Int., 2021, 146, 106204 CrossRef CAS PubMed
.
-
C. Eschauzier, P. de Voogt, H. J. Brauch and F. T. Lange, Polyfluorinated Chemicals in European Surface Waters, Ground- and Drinking Waters, in Polyfluorinated Chemicals and Transformation Products, ed. T. P. Knepper and F. T. Lange, Springer Berlin Heidelberg, Berlin, Heidelberg, 2012, pp. 73–102, [cited 2022 Feb 2], available from: http://link.springer.com/10.1007/978-3-642-21872-9_5 Search PubMed
.
- W. A. Gebbink, L. van Asseldonk and S. P. J. van Leeuwen, Presence of Emerging Per- and Polyfluoroalkyl Substances (PFASs) in River and Drinking Water near a Fluorochemical Production Plant in the Netherlands, Environ. Sci. Technol., 2017, 51(19), 11057–11065 CrossRef CAS PubMed
.
- N. Yamashita, K. Kannan, S. Taniyasu, Y. Horii, T. Okazawa and G. Petrick,
et al., Analysis of Perfluorinated Acids at Parts-Per-Quadrillion Levels in Seawater Using Liquid Chromatography-Tandem Mass Spectrometry, Environ. Sci. Technol., 2004, 38(21), 5522–5528 CrossRef CAS PubMed
.
- M. Liang,
et al. High throughput analysis of 21 perfluorinated compounds in drinking water, tap water, river water and plant effluent from southern China by supramolecular solvents-based microextraction coupled with HPLC-Orbitrap HRMS, Environ. Pollut., 2020, 263, 114389 CrossRef CAS PubMed
.
- Y. Pico, C. Blasco, M. Farré and D. Barceló, Occurrence of perfluorinated compounds in water and sediment of L’Albufera Natural Park (Valéncia, Spain), Environ. Sci. Pollut. Res., 2012, 19, 946–957 CrossRef CAS PubMed
.
- A. M. Ali,
et al. Per- and polyfluoroalkyl substances (PFASs) in contaminated coastal marine waters of the Saudi Arabian Red Sea: a baseline study, Environ. Sci. Pollut. Res., 2021, 28, 2791–2803 CrossRef CAS PubMed
.
- M. Allinson, N. Yamashita, S. Taniyasu, E. Yamazaki and G. Allinson, Occurrence of perfluoroalkyl substances in selected Victorian rivers and estuaries: An historical snapshot, Heliyon, 2019, 5(9), e02472 CrossRef PubMed
.
- C. Gallen, C. Baduel, F. Y. Lai, K. Thompson, J. Thompson and M. Warne,
et al., Spatio-temporal assessment of perfluorinated compounds in the Brisbane River system, Australia: Impact of a major flood event, Mar. Pollut. Bull., 2014, 85(2), 597–605 CrossRef CAS PubMed
.
- Y. Pan, H. Zhang, Q. Cui, N. Sheng, L. W. Y. Yeung and Y. Sun,
et al., Worldwide Distribution of Novel Perfluoroether Carboxylic and Sulfonic Acids in Surface Water, Environ. Sci. Technol., 2018, 52(14), 7621–7629 CrossRef CAS PubMed
.
- J. E. Naile, J. S. Khim, S. Hong, J. Park, B. O. Kwon and J. S. Ryu,
et al., Distributions and bioconcentration characteristics of perfluorinated compounds in environmental samples collected from the west coast of Korea, Chemosphere, 2013, 90(2), 387–394 CrossRef CAS PubMed
.
- L. Ahrens, Polyfluoroalkyl compounds in the aquatic environment: a review of their occurrence and fate, J. Environ. Monit., 2011, 13(1), 20–31 RSC
.
- S. Harrad, N. Wemken, D. S. Drage, M. A. E. Abdallah and A. M. Coggins, Perfluoroalkyl Substances in Drinking Water, Indoor Air and Dust from Ireland: Implications for Human Exposure, Environ. Sci. Technol., 2019, 53(22), 13449–13457 CrossRef PubMed
.
- J. L. Guelfo and D. T. Adamson, Evaluation of a national data set for insights into sources, composition, and concentrations of per- and polyfluoroalkyl substances (PFASs) in U.S. drinking water, Environ. Pollut., 2018, 236, 505–513 CrossRef CAS PubMed
.
- H. Guo, N. Sheng, Y. Guo, C. Wu, W. Xie and J. Dai, Exposure to GenX and its novel analogs disrupts fatty acid metabolism in male mice, Environ. Pollut., 2021 Dec, 291, 118202 Search PubMed
.
-
US EPA, Fact Sheet: Human Health Toxicity Assessment for GenX Chemical, 2021, available from: https://www.epa.gov/system/files/documents/2021-10/genx-final-tox-assessment-general_factsheet-2021.pdf.
- M. Llorca, M. Farré, Y. Picó, J. Müller, T. P. Knepper and D. Barceló, Analysis of perfluoroalkyl substances in waters from Germany and Spain, Sci. Total Environ., 2012 Aug, 431, 139–150 Search PubMed
.
|
This journal is © The Royal Society of Chemistry 2022 |