DOI:
10.1039/D1AY01747K
(Paper)
Anal. Methods, 2022,
14, 44-51
Eu-Doped MOF-based high-efficiency fluorescent sensor for detecting 2,4-dinitrophenol and 2,4,6-trinitrophenol simultaneously†
Received
13th October 2021
, Accepted 25th November 2021
First published on 30th November 2021
Abstract
Nitroaromatic explosives pose a great threat to the environment and human safety. It is very important to design simple, highly efficient and multifunctional sensors for detecting nitroaromatic explosives. However, a few sensors can determine multicomponent nitroaromatic explosives simultaneously. Eu functionalized MOF-253 (Eu@MOF-253) hybrid material was synthesized using the post-synthetic modification method. The introduction of Eu3+ in MOF-253 caused the fluorescence peak of the ligand to show a distinct red-shift due to its polarization enhancement effect in the presence of 2,4-DNP. The emission and excitation spectra of the Eu@MOF-253 sensor showed overlap with the ultraviolet-visible (UV-vis) absorption spectra of the representative nitroaromatic explosives 2,4-dinitrophenol (2,4-DNP) and 2,4,6-trinitrophenol (TNP). Therefore, it is feasible to discriminate and quantify TNP and 2,4-DNP simultaneously. As proposed, the Eu@MOF-253 luminescent sensor was highly sensitive and selective towards TNP and 2,4-DNP. The other coexisting nitroaromatic explosives did not interfere with the determination. Upon addition of TNP, the fluorescence of the Eu@MOF-253 sensor decreased dramatically and showed an excellent quenching constant (Ksv) of 1.58 × 106. The fluorescence intensities of the Eu@MOF-253 sensor presented good linear relationships with concentrations of TNP and 2,4-DNP ranging from 0.01–100 μM and 0.01–25 μM, respectively. Low limits of detection (LOD) for both 2,4-DNP and TNP were approximately 10 nM. The determination mechanism is mainly ascribed to the internal filtration effect (IFE) and electron transfer. This work provides a practical method for the highly efficient determination of nitroaromatic explosives.
1. Introduction
The rapid, highly selective and sensitive determination of nitroaromatic explosives has attracted extensive attention in recent years. Many nitroaromatic compounds such as nitrobenzene (NB), p-nitrotoluene (PNT), 1,4-dinitrobenzene (1,4-DNB), 1,2-dinitrobenzene (1,2-DNB), 2,4-dinitrotoluene (2,4-DNT), 2,4-dinitrophenol (2,4-DNP), 2,4,6-trinitrophenol (TNP), and p-nitrophenol (PNP) belong to nitroaromatic explosives. Once these nitroaromatic explosives leak into the environment, public security will be seriously threatened.1–4 Excessive inhalation of nitroaromatic explosives leads to a series of human health problems, such as skin lesions, damage to the central nervous system and cardiovascular systems.5,6 Many instrumental methods including surface-enhanced Raman spectroscopy, gas chromatography, mass spectrometry, capillary electrophoresis have been developed to detect nitroaromatic explosives efficiently.7–11 However, some instruments are expensive and require tedious pretreatment steps, which are not suitable for on-line determination.
Fluorescence analysis technology has generated considerable attention due to its rapid response, simple and high sensitivity.12–14 Many fluorescent probes for nitroaromatic explosives with high sensitivity have been reported, such as polymers/monomers with delocalized-electrons, organic compounds with polyaromatic groups.15–17 Most of these organic fluorescent probes for nitroaromatic explosives are based on the mechanism of fluorescence quenching. MOFs have been explored as promising fluorescent probes for small molecules due to their adjustable pore size, multifunctional groups, large surface area, and easy preparation.18–21 Some of these MOFs were utilized as nitroaromatic explosives fluorescent probes.22–24 However, most of the organic and metal complex-based fluorescent probes are toxic and show poor water-solubility and exhibit fluorescence emission only in highly toxic organic solvents. Moreover, most of them show poor selectivity and cannot distinguish these nitroaromatic explosives with an obvious fluorescent response. Therefore, it is still a great challenge to design a highly selective fluorescent probe for the determination of multicomponent nitroaromatic explosives simultaneously.
To achieve this goal, low toxicity, economical and high-density N-containing ligand (2,2′-bipyridine-5,5′-dicarboxylic acid) was used to coordinate with Al3+ to synthesize the MOF-253 fluorescent sensor for the sensing of multicomponent nitroaromatic explosives simultaneously (Scheme 1). N atoms in Eu@MOF-253 show a strong affinity with electron-deficient target analytes and energy transfer is inhibited from the ligand to metal in the presence of electron-deficient target analytes.25 Eu3+ was introduced into MOF-253 considering that Eu3+ can bond with nitro groups of target analytes and enhance the interaction between MOF-253 and target analytes.26 Therefore, the determination ability of target analytes is improved. The excitation and emission spectra of Eu@MOF-253 show an overlap with absorption spectra of 2,4-DNP and TNP. Spectral superposition facilitates the highly selective determination of 2,4-DNP and TNP without any interference from other nitroaromatic explosives. The experiment results confirm that the Eu@MOF-253 sensor shows excellent determination performance for 2,4-DNP and TNP with extraordinary sensitivity, high selectivity and good stability.
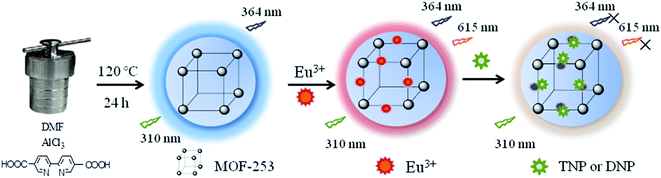 |
| Scheme 1 Schematic representation for the synthesis of Eu@MOF-253 sensor and its interaction with 2,4-DNP and TNP. | |
2. Experimental
2.1 Reagents
Europium nitrate (Eu(NO3)3, 99.99%) was procured from Ruike Rare Earth Metallurgy and Functional Materials Co., Ltd (Baotou, China). 2,2′-bipyridine-5,5′-dicarboxylic acid (H2bpydc) was purchased from Aladdin Reagent Co., Ltd (Shanghai, China). Unless specifically stated, all reagents were analytically pure and used directly without further treatment.
2.2 Instrument
The morphology of Eu@MOF-253 nanocomposite materials was studied using scanning electron microscopy (SEM, S-3400N, HITACHI). UV-visible absorption spectra were obtained on a Lambda 35 spectrophotometer (PerkinElmer, UK). Fourier transform infrared spectra (FTIR) were recorded on an avatar 360 FTIR spectrometer (Nicolet, USA). The measurements of fluorescence spectra were performed on an LS-55 fluorescence spectrometer (PerkinElmer, UK). X-ray diffraction (XRD) patterns were recorded using an AXS-D8 X-ray diffractometer (Bruker, Germany).
2.3 Synthesis of MOF-253
MOF-253 was prepared according to the previous report.27
2.4 Post-synthetic modification of Eu@MOF-253
Eu@MOF-253 was synthesized by a post-modification method. MOF-253 powder (100 mg) was introduced into 10 mL of EuCl3·6H2O ethanol solution (2 mmol). Then, the mixed solution was continually stirred at 25 °C for 1 day. The resulting solution was centrifuged and washed with ethanol three times to achieve the final product. The prepared Eu@MOF-253 white powder was dried at 80 °C in a vacuum oven for 6 h.28
2.5 2,4-DNP and TNP sensing experiment
20 mg of the Eu@MOF-253 sensor was added to 5 mL of ethanol solution and stirred ultrasonically for 5 minutes to obtain a homogeneous suspension (4 mg mL−1). Various nitroaromatic explosives were added to the Eu@MOF-253 ethanol solution to study the selectivity. 9.0 μL of 10 mM nitroaromatic explosives (NB, PNT, 1,4-DNB, 1,2-DNB, 2,4-DNT, TNT, PNP, 2,4-DNP, and TNP) or interferential metal ions (Ca2+, Mg2+, Cu2+, Mn2+, Hg2+, Pb2+, Cd2+ and Zn2+) were added to 1.5 mL Eu@MOF-253 ethanol suspension solution. Emission spectra of mixtures were then measured under 310 nm light excitation. Furthermore, different concentrations of 2,4-DNP (0–100 μM final concentration) and TNP (0–25 μM final concentration) test samples were introduced into 1.5 mL Eu@MOF-253 suspension solution to study the sensitivity. The relationships between fluorescent intensities of Eu@MOF-253 and various concentrations of 2,4-DNP and TNP were analyzed.
3. Results and discussion
3.1 Characterizations of MOF-253 and Eu@MOF-253
A simple one-pot hydrothermal synthesis was performed to prepare MOF-253. Al3+ cations combined with H2bpydc were used to construct a three-dimensional framework structure. SEM images show that the porous framework has a flower-like shape with a 15 μM diameter, and similar morphology was found after doping with Eu3+ (Fig. 1). EDS spectra were used to determine the chemical compositions of MOF-253 and Eu@MOF-253 compounds. As displayed in Fig. S1,† peaks belonging to Al3+, C, N and O elements were found in the MOF-253 compound, a new peak ascribed to Eu3+ was observed in the Eu@MOF-253 compound except for Al3+, C, N and O elements, which testify that Eu3+ has been introduced in MOF-253, successfully. The contents of Al3+ and Eu3+ in Eu@MOF-253 were 5.20 and 0.96 wt%, respectively, which was tested by ICP (inductively coupled plasma analysis). The XRD pattern (Fig. S2†) revealed that MOF-253 has a crystal structure in the absence and presence of Eu3+. The N2 adsorption isotherm experiments on microporous MOF-253 and Eu@MOF-253 were performed at 373 K (Fig. S3†). Brunauer–Emmett–Teller (BET) surface areas of MOF-253 and Eu@MOF-253 were 304 and 109 m2 g−1, respectively. The lower surface area of Eu@MOF-253 resulted from pore-clogging by Eu3+. The porosity of Eu@MOF-253 was beneficial to the enrichment of the target analyte, which was conducive to improving the selectivity and sensitivity of determination.
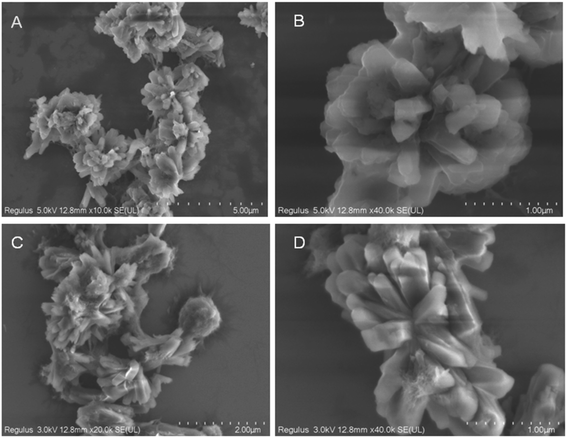 |
| Fig. 1 SEM images of MOF-253 (A and B) and Eu@MOF-253 (C and D). | |
3.2 Fluorescence property of Eu@MOF-253 sensor
The fluorescence spectrum of the Eu@MOF-253 sensor is presented in Fig. S4.† Upon excited at 310 nm, a board band centered at 365 nm is presented in the emission spectrum of the Eu@MOF-253 sensor originating from organic ligands. In addition, strong characteristic peaks are found located at 591, 612 nm attributed to 5D0 → 7F1 (591 nm), 5D0 → 7F2 (612 nm) transitions of Eu3+ (red line). This demonstrates that Eu3+ ions could show sensitized luminescence by the organic ligand. The inset in Fig. S4† shows that the Eu@MOF-253 sensor is a white suspension under visible light, while bright-red fluorescence can be visualized under irradiation of ultraviolet light.
3.3 Fluorescence response of Eu@MOF-253 sensor to different solvents
The influence of various solvents on the fluorescence of the Eu@MOF-253 sensor was studied. 10 μL Eu@MOF-253 sensor stock suspension (4 mg mL−1) was introduced into different solvents (1.5 mL) including deionized water, acetone, ethanol, dichloromethane, trichloromethane, n-hexane, N,N-dimethylformamide, tetrahydrofuran, ethyl acetate, toluene, nitrobenzene, dimethyl sulfoxide and acetonitrile. Interestingly, only nitrobenzene resulted in obvious fluorescence quenching in all solvents (Fig. 2). The test results demonstrate that the Eu@MOF-253 sensor can show strong fluorescence in deionized water and various organic solvents, except for nitrobenzene, which suggests that the Eu@MOF-253 sensor has high selectivity for nitroaromatic compounds. Considering that most nitroaromatic explosives have a higher solubility in ethanol solvents than in deionized water and the fluorescence peak of the Eu@MOF-253 sensor displays a more obvious red-shift with the increase of 2,4-DNP concentrations in ethanol solution than in deionized water (Fig. S5†). Therefore, ethanol solvent was selected in the subsequent experiments.
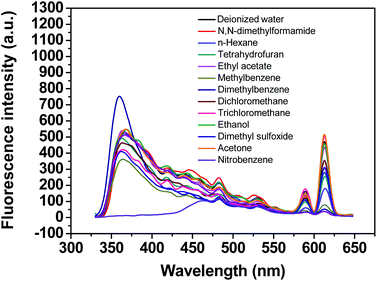 |
| Fig. 2 Fluorescence responses of Eu@MOF-253 sensor in the presence of difference solvents. | |
3.4 Selectivity of Eu@MOF-253 sensor for nitroaromatic explosives
The excellent fluorescence property of the Eu@MOF-253 sensor encouraged us to evaluate its ability for the determination of nitroaromatic explosives. To evaluate the selectivity of Eu@MOF-253 sensor for nitroaromatic explosives, 60 μM solution containing different nitroaromatic explosives (NB, PNT, 1,2-DNB, 2,4-DNT, 1,4-DNB, PNP, 2,4-DNP, TNT and TNP) or metal ions (Ca2+, Mg2+, Cu2+, Mn2+, Hg2+, Pb2+, Cd2+ and Zn2+) was added to interact with the Eu@MOF-253 sensor (1.5 mL, 4 mg mL−1 ethanol solution). As illustrated in Fig. 3, the fluorescence of the Eu@MOF-253 sensor was quenched obviously upon the addition 30 μM 2,4-DNP or TNP, while slight fluorescence quenching was found after adding other nitroaromatic explosives and metal ions. The results suggest that the Eu@MOF-253 sensor in ethanol solution shows high specificity to 2,4-DNP and TNP over other nitroaromatic explosives and coexisting substances.
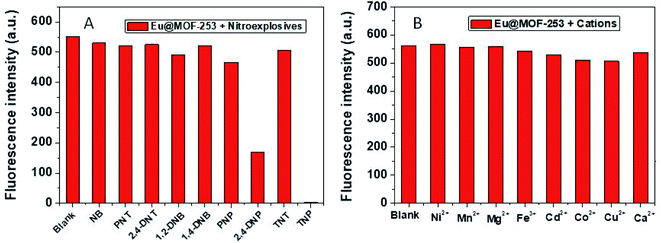 |
| Fig. 3 Fluorescence responses of Eu@MOF-253 sensor upon the addition of 60 μM various nitroaromatic explosives (A) and cations (B). | |
3.5 Fluorescence determination of Eu@MOF-253 sensor for 2,4-DNP and TNP
In view of the high selectivity of the Eu@MOF-253 sensor toward 2,4-DNP and TNP, quantitative tests of 2,4-DNP and TNP were performed under 310 nm excitation wavelength and 365 nm emission wavelength resulting in the data shown in Fig. S6.† The fluorescence intensity of the Eu@MOF-253 sensor decreases gradually with the increase of 2,4-DNP concentration (Fig. 4). Upon 310 nm UV light irradiation, the fluorescence color of the Eu@MOF-253 sensor changes from rose-red to light pink with 2,4-DNP concentration from 0 to 100 μM (inset in Fig. 4). The quenching efficiency (I0/I) of the Eu@MOF-253 sensor shows excellent linearity with 2,4-DNP concentrations in the range of 0.01–100 μM and the LOD for 2,4-DNP is 10 nM. TNP shows a higher quenching efficiency toward the Eu@MOF-253 sensor (Fig. 5) and the rose-red fluorescence of the Eu@MOF-253 sensor (inset in Fig. 5) under 310 nm UV irradiation gradually turns to lavender with increasing TNP concentrations (0–100 μM). I0/I values display good linearity with TNP concentrations ranging from 0.01–25 μM and a low LOD of 10 nM. The addition of 25 μM 2,4-DNP resulted in 60% fluorescence quenching, while 25 μM TNP caused 97% fluorescence quenching. The introduction of TNP in Eu@MOF-253 suspension shows a little red-shift. The red-shift occurrence suggests that the strong interaction between the electron-rich Eu@MOF-253 and electron-deficient 2,4-DNP.29 In this case, the reason responsible for red-shift is related to the number and distribution of nitro groups. 2,4-DNP is m-nitrophenol and the electron distribution on the benzene ring is not uniform, especially under the bonding of Eu3+, resulting in polarization enhancement in the local microenvironment of the sensing system.30 TNP has three –NO2 groups, which are distributed symmetrically on the benzene ring and lead to a uniform electron distribution. Therefore, the polarization of the Eu@MOF-253 sensor induced by TNP is not as strong as that induced by 2,4-DNP. In order to verify the polarization-induced effect of Eu3+, MOF-253 was performed as a sensor for 2,4-DNP and TNP. From the observation of Fig. S7,† a slightly red-shift was found in the fluorescence emission of MOF-253 upon addition of different concentrations of 2,4-DNP. While no red-shift was found in the case of TNP. Perhaps this is because of the fact that Eu3+ can bond with –NO2 groups in 2,4-DNP and generate dipole–dipole interaction with 2,4-DNP, resulting in a red-shift of Eu@MOF-253 fluorescence emission.31,32 The Stern–Volmer (SV) equation was used to calculate the quenching constant (Ksv) to further evaluate the determination ability of the sensor. Excited by 310 nm wavelength, SV plots of 2,4-DNP and TNP presented linear relationships in a wide range, indicating that the quenching was static quenching (Fig. S8†).33,34 The calculated Ksv values are 4.78 × 104 for 2,4-DNP and 2.33 × 105, 1.58 × 106 for TNP. The Ksv of TNP can be compared with those reported in the literature (Table S1†). A much higher Ksv for the Eu@MOF-253 sensor by TNP indicates the high sensitivity of the Eu@MOF-253 sensor for the determination of TNP.
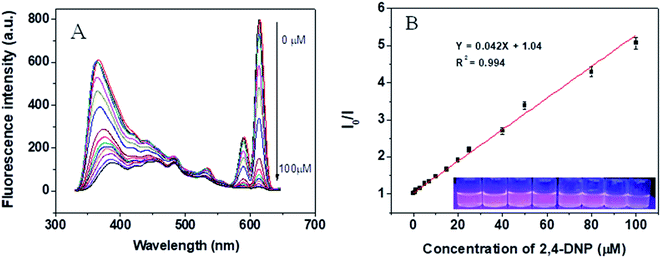 |
| Fig. 4 (A) Fluorescence emission spectra of Eu@MOF-253 compound upon the addition of different concentrations of 2,4-DNP (0, 0.1, 0.3, 0.8, 1, 3, 5, 7, 10, 15, 20, 25, 40, 50, 80, 100 μM); (B) the linear relationship between the fluorescence intensities of the Eu@MOF-253 sensor at 365 nm and the concentrations of 2,4-DNP, inset: fluorescence color photos of Eu@MOF-253 sensor upon various 2,4-DNP concentrations (under 302 nm UV lamp). | |
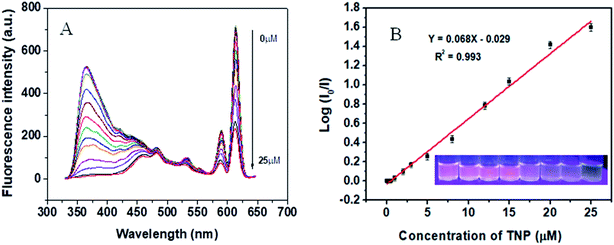 |
| Fig. 5 (A) Fluorescence emission spectra of Eu@MOF-253 compound exposed to various concentrations of TNP (0, 0.01, 0.08, 0.1, 0.5, 1, 2, 3, 5, 7, 8, 10, 12, 15, 20, 25 μM); (B) calibration plot of log I0/I vs. the TNP concentrations. Fluorescence color photos of Eu@MOF-253 sensor over various TNP concentrations (under 302 nm UV lamp). | |
3.6 The principles of simultaneous determination of TNP and 2,4-DNP
Nitroaromatic explosives with electron-deficient properties usually work as electron acceptors. Most fluorescence sensors for nitroaromatic explosives are based on the mechanism of fluorescence quenching resulting from electron transfer. However, not all nitroaromatic explosives can trigger a highly sensitive response of the sensor. It is related to the interaction between nitroaromatic explosives and sensors. Those nitroaromatic explosives with strong electron-deficient properties and hydroxyl groups show a strong interaction with high-density N-containing sensors. To verify the interaction between Eu@MOF-253 and nitro compounds, FT-IR spectroscopy experiments were performed. As shown in Fig. 6(A), peaks located at 1474 and 1672 cm−1 in Eu@MOF-253 (correspond to stretching vibration of C
C and C
O, respectively) changed little in the presence of TNP and 2,4-DNP, confirming the chemical bonding interaction did not exist between Eu@MOF-253 and TNP or the 2,4-DNP compound. The hydroxyl stretching vibration peak (at 3414 cm−1) shifts to 3429 and 3435 cm−1 after the addition of TNP and 2,4-DNP, respectively. The red-shift of hydroxyl stretching vibration peak suggests the generation of hydroxyl bonding interaction between Eu@MOF-253 and TNP or the 2,4-DNP compound.35 The formation of hydrogen bonding provides a bridge facilitating electron transfer from the Eu@MOF-253 sensor to nitroaromatic explosives.36,37
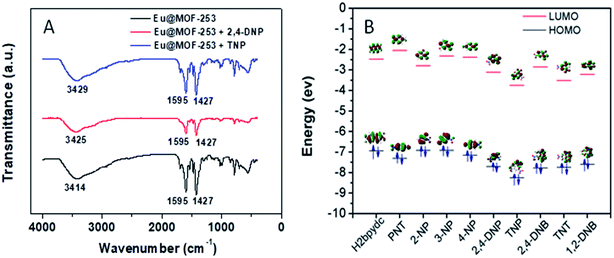 |
| Fig. 6 (A) FTIR spectra of Eu@MOF-253, Eu@MOF-253 + 2.4-DNP and Eu@MOF-253 + TNP; (B) energy profile diagram of ligand (H2bpydc) and nitroaromatic explosives with calculated HOMO (black) and LUMO (red) energies by DFT. | |
To elucidate the electron transfer quenching mechanism, the ultraviolet diffuse reflection of the Eu@MOF-253 sensor was used to determine the changes of semiconductor energy gaps (Eg) upon the addition of various nitroaromatic explosives. The detection of Eg is based on the following formula: (Ahν/K)1/2 = hν − Eg. A curve was drawn according to this formula. hν was set as the abscissa and (Ahν/K)1/2 as the ordinate. Then, the intersection of tangent and abscissa is Eg. As shown in Fig. S9,† the Eg of Eu@MOF-253 sensor decreases from 3.43 to 2.73 and 2.35 eV, respectively, exposure to 60 μM 2,4-DNP and TNP. The results suggest a strong interaction exists between the Eu@MOF-253 sensor and 2,4-DNP or TNP, which is corresponding to the results of fluorescence determination.
In the meantime, the lowest unoccupied molecular orbitals (LUMO) of the electron-rich donor and electron-deficient receptor were calculated using the B3LYP/6-31G* method of density functional theory (DFT). Upon excitation, electrons in the conduction band (CB) of electron-rich sensors transform into the LUMO of electron-deficient target analytes, resulting in fluorescence quenching. As shown in Fig. 6(B), the order of LUMO energy levels of nitroaromatic explosives and ligand is as follows: TNP < 2,4-DNB < TNT < 2,4-DNP < 1,2-DNB < PNT < ligand < 4-NP < 2-NP < 3-NP. The LUMO energy of TNP is the lowest among these target nitroaromatic explosives. The greatest LUMO energy-level difference between ligand and TNP means that electrons on the sensor are more likely to transfer to TNP, which leads to the highest quenching efficiency for the Eu@MOF-253 sensor. However, the above LUMO energy order of the nitroaromatic explosives is not consistent with their fluorescence quenching efficiency, suggesting the photoinduced electron transfer is not the main cause for quenching.
The absorption spectra of the nitroaromatic explosives and the fluorescence spectra of Eu@MOF-253 were measured. As displayed in Fig. 7, the absorption spectra of 2,4-DNP and TNP show the largest spectral superimposition with the emission spectrum of Eu@MOF-253, which leads to fluorescence quenching of the Eu@MOF-253 sensor ascribed to resonance energy transfer. The quenching degree of the sensor is consistent with the size of the spectral superposition area. A competitive light absorption will also result in fluorescence quenching. The excitation spectrum of the Eu@MOF-253 sensor also shows a large spectral superposition with the absorption spectra of TNP and 2,4-DNP (Fig. S10†). Therefore, 2,4-DNP or TNP will absorb the excitation light competitively and decrease the excitation light intensity leading to fluorescence quenching. Resonance energy transfer and a competitive light absorption result in an internal filtering effect (IFE).38,39 The results indicate that the joint action of electron transfer and IFE cause fluorescence quenching of the Eu@MOF-253 sensor.
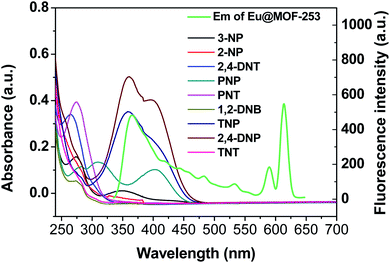 |
| Fig. 7 UV-vis absorption spectra of various nitro explosives and fluorescence excitation (green line) and emission spectra (purple line) of Eu@MOF-253. | |
3.7 Practical applications of Eu@MOF-253 sensor for 2,4-DNP and TNP
Cycling tests were used to study the recyclability of the Eu@MOF-253 sensor. 60 μM DNP and 25 μM TNP were added to Eu@MOF-253 ethanol solution with the same concentration, standing for 5 minutes, and changes of fluorescence intensities were tested. Eu@MOF-253 ethanol solution can be regenerated by centrifuging and recycling up to 4 times. As displayed in Fig. S11,† the fluorescence intensity of the Eu@MOF-253 sensor slightly decreased after being repeatedly used 4 times. The experimental results suggest that the Eu@MOF-253 sensor possesses excellent recyclability. The stability of the Eu@MOF-253 sensor in the absence and presence of 2,4-DNP or TNP was tested at room temperature over 30 days (Fig. S12†). The results indicate that the Eu@MOF-253 sensor in ethanol solution displays excellent optical stability. The standard addition method was used to evaluate the practical application of the Eu@MOF-253 sensor for 2,4-DNP and TNP determination. 1.0 mmol of 2,4-DNP or TNP solid powder was mixed with 5 g dried soil. Then, the mixture was extracted with ethanol in a 100 mL volumetric flask. The extract of soil was separated by filtration. 20 mg of Eu@MOF-253 sensor was added to the filtrate. Then, the fluorescence spectra of dispersions were determined under 310 nm excitation. The results are shown in Table 1. The mean recoveries of 2,4-DNP and TNP were 91.6–96.1% and 93.4–96.2% with a relative standard deviation (RSD) of ±1.19–2.94% and ±2.11–3.15%, respectively. Therefore, the Eu@MOF-253 sensor works efficiently as 2,4-DNP and TNP sensor in the presence of soil.
Table 1 Measurements of 2,4-DNP or TNP spiked in dry soil using Eu@MOF-253 sensor. Data were obtained from three replicates
Analytes |
Spiked (μM) |
Detected |
Recovery (μM, mean ± SD) |
RSD (n = 3) (%, mean) |
2,4-DNP |
5.00 |
4.58 ± 0.11 |
91.6 ± 2.20 |
2.94 |
10.00 |
9.47 ± 0.16 |
94.7 ± 1.60 |
2.07 |
30.00 |
28.83 ± 0.28 |
96.1 ± 0.93 |
1.19 |
TNP |
5.00 |
4.67 ± 0.12 |
93.4 ± 2.40 |
3.15 |
10.00 |
9.62 ± 0.23 |
96.2 ± 2.30 |
2.93 |
20.00 |
19.12 ± 0.33 |
95.6 ± 1.65 |
2.11 |
4. Conclusion
A simple, highly selective and sensitive Eu@MOF-253 sensor was fabricated based on a high-density nitrogen-containing ligand, which can be used to selectively determine nitroaromatic explosives 2,4-DNP and TNP simultaneously, even coexisting with a certain number of other nitroaromatic explosives and common metal ions. The mechanisms for detecting 2,4-DNP and TNP are mainly ascribed to IFE and electron transfer. The eu@MOF-253 sensor shows high Ksv values and low LOD values for 2,4-DNP and TNP. Moreover, the Eu@MOF-253 sensor possesses advantages of excellent stability and recyclability. The measurement of soil samples containing nitroaromatic explosives verified the reliability of the sensor. The results demonstrate that the Eu@MOF-253 sensor is a promising sensor for simultaneously monitoring nitroaromatic explosives 2,4-DNP and TNP in environmental samples.
Author contributions
Lili Chen: investigation, writing-review & editing, supervision. Zihan Cheng: conceptualization, investigation, data curation, writing-original draft. Xinyue Peng: investigation, data curation. Guoqiao Qiu: writing, correcting. Li Wang: writing-review & editing, supervision, funding acquisition.
Conflicts of interest
The authors declare that they have no known competing financial interests or personal relationships that could have appeared to influence the work reported in this paper.
Acknowledgements
This work was financially supported by National Natural Science Foundation of China (21765009 and 21964010), and the program of Scientific Research Fund of Jiang Xi Provincial Education Department (grant no. 200312).
References
- S. Devi, S. Shaswat, V. Kumar, A. Sachdev, P. Gopinath and S. Tyagi, Microchim. Acta, 2020, 187, 536 CrossRef CAS PubMed.
- M. Bagheri, M. Y. Masoomi, A. Morsali and A. Schoedel, ACS Appl. Mater. Interfaces, 2016, 8, 21472–21479 CrossRef CAS PubMed.
- R. R. Gao, J. H. Wang, H. Wang, W. Dong and J. W. Zhu, Microchim. Acta, 2021, 188, 18 CrossRef CAS PubMed.
- R. B. Fu, S. M. Hu and X. T. Wu, J. Mater. Chem. A, 2017, 5, 1952–1956 RSC.
- D. Tian, Y. Li, R. Y. Chen, Z. Chang, G. Y. Wang and X. H. Bu, J. Mater. Chem. A, 2014, 2, 1465–1470 RSC.
- Y. Hu, M. Ding, X. Q. Liu, L. B. Sun and H. L. Jiang, Chem. Commun., 2016, 52, 5734–5737 RSC.
- B. Zheng, Y. Li, F. Tao, Y. Cui and T. Li, Sens. Actuators, B, 2017, 241, 357–363 CrossRef CAS.
- A. Kumar and P. S. Chae, Sens. Actuators, B, 2017, 240, 1–9 CrossRef CAS.
- Y. Zhang, M. Xu, B. R. Bunes, N. Wu, D. E. Gross, J. S. Moore and L. Zang, ACS Appl. Mater. Interfaces, 2015, 7, 7471–7475 CrossRef CAS PubMed.
- M. Liu and W. Chen, Biosens. Bioelectron., 2013, 46, 68–73 CrossRef CAS PubMed.
- Z. Guo, J. Hwang, B. Zhao, H. Chung and G. Cho, Analyst, 2014, 139, 807–812 RSC.
- P. Tayaa, B. Maitib, V. Kumara, P. Deb and S. Satapathi, Sens. Actuators, B, 2018, 255, 2628–2634 CrossRef.
- K. Ponnuvel, G. Banuppriya and V. Padmini, Sens. Actuators, B, 2016, 234, 34–45 CrossRef CAS.
- L. Ding, Y. Bai, Y. Cao, G. Ren, G. J. Blanchard and Y. Fang, Langmuir, 2014, 30, 7645–7653 CrossRef CAS.
- P. G. Del Rosso, M. F. Almassio and R. O. Garay, Tetrahedron Lett., 2011, 52, 4911–4915 CrossRef CAS.
- H. Bai, C. Li and G. Shi, Sens. Actuators, B, 2008, 130, 777–782 CrossRef CAS.
- P. Venkatesan, S. P. Wu, D. Udhayakumari and S. Velmathi, Apyrene-linked thiourea as a chemosensor for cations and simple fluorescent sensor for picric acid, Anal. Methods, 2015, 7, 1161–1166 RSC.
- J. A. Mason, J. Oktawiec, M. K. Taylor, M. R. Hudson, J. Rodriguez, J. E. Bachman, M. I. Gonzalez, A. Cervellino, A. Guagliardi, C. M. Brown, P. L. Llewellyn, N. Masciocchi and J. R. Long, Nature, 2015, 527, 357–361 CrossRef CAS PubMed.
- S. Yang, X. Lin, W. Lewis, M. Suyetin, E. Bichoutskaia, J. E. Parker, C. C. Tang, D. R. Allan, P. J. Rizkallah, P. Hubberstey, N. R. Champness, K. M. Thomas, A. J. Blake and M. Schröder, Nat. Mater., 2012, 11, 710–716 CrossRef CAS PubMed.
- H. Furukawa, N. Ko, Y. B. Go, N. Aratani, S. B. Choi, E. Choi, A. Ö. Yazaydin, R. Q. Snurr, M. O'Keeffe, J. Kim and O. M. Yaghi, Science, 2010, 329, 424–428 CrossRef CAS PubMed.
- A. I. Cooper and M. J. Rosseinsky, Nat. Chem., 2009, 1, 26–27 CrossRef CAS.
- B. Parmar, Y. Rachuri, K. K. Bisht, R. Laiya and E. Suresh, Inorg. Chem., 2017, 56, 2627–2638 CrossRef CAS PubMed.
- Z. Xu, Y. Wen, L. Tian and G. Li, Inorg. Chem. Commun., 2017, 77, 11–13 CrossRef CAS.
- L. X. Yin, Y. J. Wang, R. Tan, H. L. Li and Y. F. Tu, Microchim. Acta, 2021, 188, 53 CrossRef CAS PubMed.
- C. Wang, J. Shang, Y. Lan, T. Tian, H. Wang, X. Chen, J. Y. Gu, J. Z. Liu, L. J. Wan, W. Zhu and G. Li, Adv. Funct. Mater., 2015, 25, 6009–6017 CrossRef CAS.
- F. Wang, J. H. Sun, J. P. Wang, S. Y. Bai and X. Wu, Mater. Chem. Phys., 2014, 145, 471–475 CrossRef CAS.
- E. D. Bloch, D. Britt, C. Lee, C. J. Doonan, F. J. Uribe-Romo, H. Furukawa, J. R. Long and O. M. Yaghi, J. Am. Chem. Soc., 2010, 132, 14382–14384 CrossRef CAS.
- S. J. Qin and B. Yan, Sens. Actuators, B, 2018, 259, 125–132 CrossRef CAS.
- P. Das and S. K. Mandal, J. Mater. Chem. C, 2018, 6, 3288–3297 RSC.
- S. P. Yang, L. Lin, L. Z. Yang, J. M. Chen, Q. Q. Chen, D. Cao and X. B. Yu, J. Lumin., 2007, 126, 515–530 CrossRef CAS.
- O. Gungor and M. Kose, Sens. Actuators, B, 2018, 264, 363–371 CrossRef CAS.
- D. H. Zhao and T. M. Swager, Macromolecules, 2005, 38, 9377–9384 CrossRef CAS.
- A. Deshmukh, S. Bandyopadhyay, A. James and A. Patra, J. Mater. Chem. C, 2016, 4, 4427–4433 RSC.
- B. Wang, X. L. Lv, D. Feng, L. H. Xie, J. Zhang, M. Li, Y. Xie, J. R. Li and H. C. Zhou, J. Am. Chem. Soc., 2016, 138, 6204–6216 CrossRef CAS PubMed.
- Y. T. Meng, Y. Jiao, Y. Zhang, W. J. Lu, X. D. Wang, S. M. Shuang and C. Dong, J. Hazard. Mater., 2021, 408, 124422 CrossRef CAS PubMed.
- J. H. Guo, W. J. Lu, H. L. Zhang, Y. T. Meng, F. F. Du, S. M. Shuang and C. Dong, Sens. Actuators, B, 2021, 330, 129360 CrossRef CAS.
- X. Ran, Q. Qu, L. Li, L. M. Zuo, S. H. Zhang, J. W. Gui, Y. X. Kang and L. Yang, ACS Sustainable Chem. Eng., 2018, 6, 11716–11723 CrossRef CAS.
- W. Liu, X. Huang, C. Xu, C. Chen, L. Yang, W. Dou, W. Chen, H. Yang and W. Liu, Chem.–Eur. J., 2016, 22, 18769–18776 CrossRef CAS PubMed.
- R. Lv, J. Wang, Y. Zhang, H. Li, L. Yang, S. Liao, W. Gu and X. Liu, J. Mater. Chem. A, 2016, 4, 15494–15500 RSC.
Footnote |
† Electronic supplementary information (ESI) available. See DOI: 10.1039/d1ay01747k |
|
This journal is © The Royal Society of Chemistry 2022 |