DOI:
10.1039/D1AN01864G
(Paper)
Analyst, 2022,
147, 72-79
Multi-tailoring of a modified MOF-derived CuxO electrochemical transducer for enhanced hydrogen peroxide sensing†
Received
14th October 2021
, Accepted 14th November 2021
First published on 16th November 2021
Abstract
Reasonable control of the redox states within the catalytic units together with the interconnection degrees of the substrate is of great significance in the modulation of a well-performing transducer. Herein, a novel carbon black (CB)-modified copper metal–organic framework nanomaterial (CB@Cu-MOF) prepared at room temperature was utilized as a precursor to synthesize mixed-valent copper–oxide composite catalysts (NC/CuxO-T). By tuning the carbonization process of the precursor at different temperatures (T = 100 °C, 200 °C, 300 °C and 400 °C), the different ratio configurations of the redox-alternated CuxO portions were successfully controlled with the simultaneous effective tailoring of the defect abundance in the N-doped carbon substrate. As a result, an optimized NC/CuxO-300 electrochemical H2O2 sensor was able to present a low detection limit (0.26 μM) and decent linear ranges (0.02–1.79 mM and 2.29–9.29 mM). Our strategy using easily available initial materials with mild preparation conditions is expected to promote the practical application of the star materials in laboratories.
Introduction
Fast and accurate determination of H2O2 has drawn considerable attention due to its essential role in industrial, environmental and medical applications.1 Exhibiting high sensitivity, low cost and robust controllability, electrochemical methods for H2O2 detection have been widely accepted in various fields from water monitoring to clinical diagnostics.2,3 Therefore, it is of great practical significance to use electrochemical techniques as well as their corresponding functional interfaces to establish accurate, sensitive, rapid and convenient analytical systems. Among them, copper-based transducer materials have always been popular thanks to their unique characteristics.4,5
Copper oxides and their composites can offer fascinating electrochemical properties due to the particular electron energy states of the copper centers.6 Specifically, CuO has an unfilled d-shell (3d9) as a p-type semiconductor. Its energy band structure can be divided into a low-energy valence band and high-energy conduction band, which has been widely applied in gas sensing and photo-catalysis.7,8 Cu2O, also a p-type semiconductor, is a commonly used narrow gap (ca. 2.1 eV) sensitizer with non-toxicity and a suitable energy level position, and thus is supposed to be a promising transducer candidate for electrochemical sensors.9 In recent years, Cu2O-based materials have also been applied in electronic devices, photo-catalysis, and lithium-ion batteries.10,11 The nanosize composite of CuO and Cu2O with a high specific surface area can further separate the generated electron–hole pairs effectively and inhibit their recombination, therefore possessing enhanced catalytic activity.12 Currently, CuO/Cu2O nano-composites can be prepared by hydrothermal methods, sonochemical methods, and a one-step flame spray pyrolysis process.13,14 These pioneering techniques opened up and promoted the application of copper-based functional interfaces, but there still remain limitations to be overcome for practical use, such as cost efficiency, preparation accessibility, or non-green chemical procedures.15
As an emerging category of porous crystalline materials, metal–organic frameworks (MOFs) present a versatile platform for the subsequent synthesis of copper-based electrocatalysts due to their advantages of large specific surface area, diverse architecture, adjustable pore size, uniform atomic distribution, etc.16–18 They are quite suitable either as self-sacrificed precursors or catalyst carriers because many structural properties, such as porosity and highly dispersed metal centers, can be inherited even after vigorous chemical processes like carbonization.19 In addition, MOFs can be further composited with other functional materials to reinforce the weakness in performance.20
Therefore, in our work, a common and easily available carbonaceous material,21 carbon black (CB), was introduced as a supplemental component in the synthesis of a copper-based MOF precursor at room temperature (Scheme 1). Due to the excellent conductivity and the reductive environment provided by CB, we investigated the controllability of this CB-doped reaction system for multiple modulations of the catalytic center's valence proportions as well as the deficiency abundance in the supporting substrate during pyrolysis. Combined with the electrochemical analysis of the calcined products obtained at 100 °C, 200 °C, 300 °C and 400 °C, we speculated the evolutionary procedures of the N-doped carbon-based multivalent copper oxide nanocatalysts (NC/CuxO-T). Based on their distinguished electrochemical activities for hydrogen peroxide reduction, a transducer interface constructed using NC/CuxO-300 was proposed with its H2O2 sensing capability examined. We hope that our protocol, which only involves readily available reagents and mild reaction conditions, can promote the widespread application of MOF derivatives in practical settings.
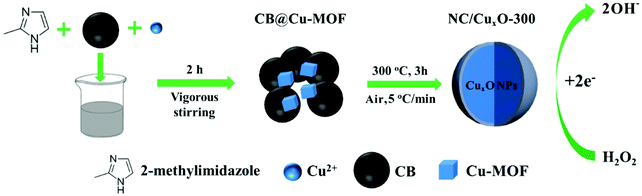 |
| Scheme 1 Schematic illustration of the fabrication process of NC/CuxO-300. | |
Experimental section
Chemicals and materials
Copper(II) nitrate trihydrate (Cu(NO3)2·3H2O, AR, 99%) and hydrogen peroxide (H2O2, AR, ≥30%) were purchased from Sigma-Aldrich Chemical Co., Ltd (Shanghai, China). 2-Methyl-imidazole (2-MI, 99%) was bought from J&K Scientific Ltd (Shanghai, China). Methanol (GR, ≥99.7%) was obtained from Sinopharm Chemical Reagent Co., Ltd (Beijing, China). Carbon black was purchased from Macklin Biochemical Technology Co., Ltd (Shanghai, China). Distilled (DI) water was used throughout the experiments.
Preparation of electrocatalysts
In a typical preparation, 0.1 g CB was added to 30 mL 0.1 M Cu(NO3)2·3H2O methanol solution with vigorous stirring at ambient temperature. Thereafter, the suspension was mixed with 30 mL 0.8 M 2-MI aqueous solution and vigorously stirred for 2 h. The product suspension was centrifuged for 15 min at 8000 rpm, and the obtained black precipitate was washed three times with 1
:
1 mixed methanol and water solution. Finally, lyophilization was carried out and the precursor CB@Cu-MOF was prepared. The contrast precursor, which only contained the Cu-MOF, was prepared using an almost identical method, except for the CB. Finally, the as-prepared precursor was finely ground for 10 min and then calcined at 100, 200, 300, and 400 °C with a heating rate of 5 °C min−1. The obtained samples were named as NC/CuxO-100, NC/CuxO-200, NC/CuxO-300 and NC/CuxO-400, respectively.
Materials characterization
The thermal gravimetric (TG) analysis was carried out on a Synchro Thermal Analyzer (STA 449 F1 Jupiter, NETZSCH) under an air atmosphere with a heating rate of 5 °C min−1. An iHR550 Raman microscope (HORIBA) was introduced for the Raman test with an excitation source of a 532 nm solid laser. The morphology analysis was performed by a XL-30E scanning electron microscope (SEM, Philips). More specific morphology was explored by a transmission electron microscope (TEM, Tecnai G2, FEI) and a high-angle annular dark-field scanning transmission electron microscope (STEM-HAADF, JEM-2100F, JEOL). X-ray photoelectron spectroscopy (XPS) studies were conducted using K-Alpha X-ray on a Thermo ESCALAB 250XI platform. The XPS binding energies were calibrated using the C 1s peak at 284.6 eV.
Electrochemical measurements
All of the electrochemical analyses were performed in a three-electrode system on an electrochemical workstation (CHI660D, CH Instrument). Typically, 5 mg of the catalysts were dispersed in 1 mL DI water by sonicating for 20 min to form a homogenous ink. To prepare a working electrode, 10 μL of the ink were loaded on a polished glass carbon electrode (GCE, ∅ 5 mm). Then, 10 μL Nafion (5 wt%) solution was dropped on the surface of the above-modified electrode and dried under ambient conditions. Meanwhile, a saturated calomel electrode (SCE) was used as the reference electrode, and a Pt wire was used as the counter electrode. The electrocatalytic performance was investigated by cyclic voltammetry (CV) in N2-saturated 0.1 M phosphate buffered saline (PBS) aqueous solution with a typical scan rate of 10 mV s−1 and a potential range from 0.5 V to −0.8 V. Steady-state measurements were performed using rotating disk electrodes (RDE, ∅ 3 mm).
Results and discussion
Pyrolysis setup for the modified MOF precursor
The constitution of the precursor CB@Cu-MOF was first examined by powder X-ray diffraction (PXRD). The striking narrow peaks could be indexed well as a 2D MOF nanosheet reported in a previous paper.22 In addition, a typical broad bulge of amorphous carbon was observed at 2θ ≈ 25°, as seen in Fig. S1,† indicating the co-existence of the copper-based MOF crystals and amorphous carbon in the composite precursor. The relationship between the calcination temperature and the mass change of CB@Cu-MOF was then determined by TG analysis. As shown in Fig. 1A, the residual mass of the precursor was 98.69% of the original at 100 °C due to the volatilization of the solvents. As the temperature increased to 400 °C, the mass loss was mainly attributed to the decomposition of the hydrogen-, oxygen- and nitrogen-containing groups with a residual mass of 91.26%. The process was accompanied by a series of exothermal peaks from the differential scanning calorimetric (DSC) curve, indicating that multiple reactions, such as the oxidation of some reductive substances, happened.23 With further increase of the carbonization temperature, the species of carbon began to decompose, which resulted in a sharp decrease of the residual mass, accompanied by a severe exothermal peak. The residual mass remained a constant of 1.87% when the temperature reached 460 °C, which means that the carbon species almost completely decomposed. According to the sharply reduced residual mass of the over-heated sample, we presumed the following formation mechanism of the composite precursor: (1) the electronegative dopant atoms in CB anchored the copper ions from the solution; (2) ligands assembled onto the copper ions and thus in situ formed a small quantity of MOF nanosheets upon the CB surface. Therefore, the temperatures for carbonization modulation were set at T = 100, 200, 300, and 400 °C accordingly, with their respective products denoted as NC/CuxO-T.
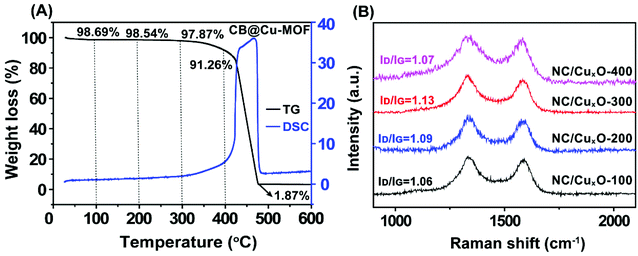 |
| Fig. 1 (A) TG–DSC curve of CB@Cu-MOF in an air atmosphere with a heating rate of 5 °C min−1. (B) Raman spectra of NC/CuxO-100, NC/CuxO-200, NC/CuxO-300 and NC/CuxO-400. | |
Multiple tailoring effects induced by CB
In order to examine the modulation of the carbon-based substrate with different carbonization temperatures, Raman measurements were carried out to confirm the carbon species in our as-prepared samples (Fig. 1B). The highest ID/IG ratio within the NC/CuxO-300 composites (1.06 for NC/CuxO-100, 1.09 for NC/CuxO-200 and 1.07 for NC/CuxO-400) demonstrated the presence of more defective structures in the carbon network, which allowed a faster electron transport.
More precisely, XPS data were analyzed to obtain a further insight into the redox states and bonding configurations of the atoms in our catalysts, as a result of the modulation in terms of the catalytic unit's formation. The full XPS survey spectra of the NC/CuxO-T catalysts (Fig. S2†) showed obvious peaks corresponding to the C 1s, N 1s, O 1s and Cu 2p orbitals at 284.6 eV, 400.9 eV, 532.8 eV and 934.0 eV, respectively. Among them, we first focused on the spectra of the Cu 2p orbital (Fig. 2A) as it could provide the most significant information about the central metal's constitution. The peaks at around 952.0 eV and 933.0 eV were ascribed to the characteristic 2p1/2 and 2p3/2 peaks for copper atoms. These excerpted spectra exhibited a significant evolutionary tendency due to the temperature control. In the low-temperature treated groups, namely the NC/CuxO-100 and NC/CuxO-200, main peaks of both the 2p1/2 and 2p3/2 orbitals appeared at the positions of lower binding energies (BEs).24 To further determine the valence state of copper in NC/CuxO-100 and NC/CuxO-200, the Cu LM2 Auger peaks were investigated, as shown in Fig. 2B. The peaks located at 916.7 eV were assigned to CuI,25 which indicated that Cu2O existed in these low-temperature treated catalysts.
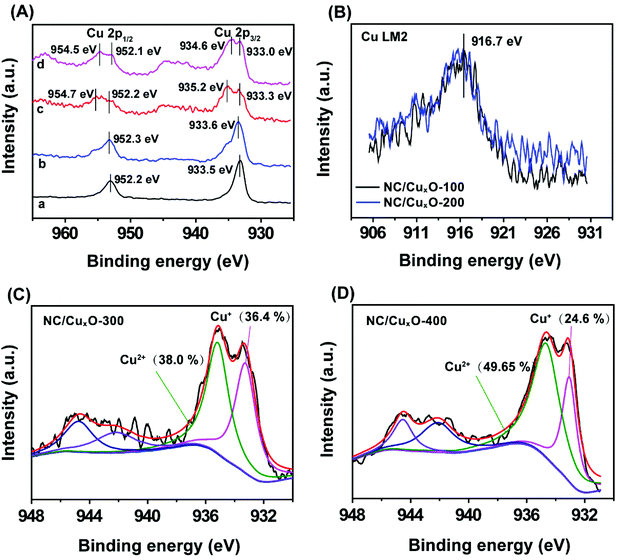 |
| Fig. 2 (A) The excerpted XPS spectra of Cu 2p in (a) NC/CuxO-100, (b) NC/CuxO-200 (c) NC/CuxO-300 and (d) NC/CuxO-400. (B) The Auger XPS spectra of Cu LM2 in NC/CuxO-100 and NC/CuxO-200. High resolution XPS spectra of Cu 2p3/2 in (C) NC/CuxO-300 and (D) NC/CuxO-400. | |
Construction of the mixed-valent catalytic nanocomposite
With the increase of temperature to 300 °C and 400 °C, additional peaks located at higher BEs of ∼935.0 eV and ∼954.5 eV could be ascribed to CuII oxides, accompanied by the characteristic CuII shake-up satellite peaks. This result suggested that the Cu species existed in the form of both plentiful Cu2O and CuO in NC/CuxO-300 and NC/CuxO-400. In contrast, Cu2O possessed the dominant portion in NC/CuxO-100 and NC/CuxO-200. Interestingly, the content of CuII gradually increased with the rise in temperature according to the stepwise humping of the high-BE peak intensities. Calculated from the high-resolution Cu 2p3/2 XPS spectra, the abundance ratio of CuO/Cu2O equaled about 1 and 2 in NC/CuxO-300 and NC/CuxO-400, respectively, as shown in Fig. 2C and D. The O 1s fine XPS spectra (Fig. S3†) demonstrated the complementing results. With the increase in temperature, the ratio of lattice oxygen, which belongs to the metal oxides, rose. Meanwhile, adequate oxygen vacancies remained in the NC/CuxO-300 catalyst.26
The morphology of NC/CuxO-300 was then examined. As shown in the SEM image (Fig. 3A), NC/CuxO-300 displayed a typical sphere-like morphology with a diameter of less than 100 nm. In the TEM images (Fig. 3B and C), the overall uniform appearance suggested a typical carbon-based and metallic compound-decorated character. Further, the dispersion of Cu, O, N and C was precisely presented by the STEM-HAADF elemental mapping image (Fig. 3D). Obviously, the distribution of O was highly associated with that of Cu, indicating that the bright points were ascribed to CuxO. Furthermore, the uniform and almost identical distribution of the N and C elements confirmed the formation of the homogeneous N-doped carbon matrix. Although at the initial precursor stage, CB and Cu-MOF, with their respective different element distributions, were in separated phases, and the subsequent atomic migration due to calcination guaranteed the highly dispersed catalytic nanoclusters and uniform supporting substrate.
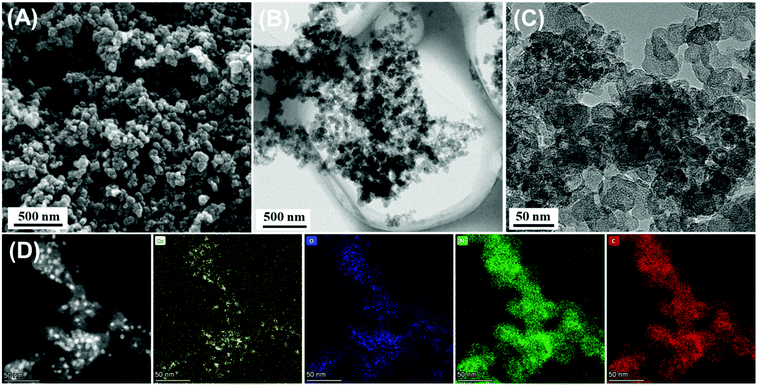 |
| Fig. 3 (A) SEM images of NC/CuxO-300 and (B, C) TEM images of NC/CuxO-300. (D) STEM-HAADF image and EDX mapping of Cu, N, C and O elements in NC/CuxO-300. | |
Enhanced electrochemical catalysis for H2O2 reduction
Knowing the basic characteristics of the NC/CuxO-T catalysts, we next studied their electrochemical behavior to reveal the synergistic mechanism between their catalytic activities and structural properties. Fig. 4A displays the cyclic voltammograms (CVs) of NC/CuxO-100, NC/CuxO-200, NC/CuxO-300 and NC/CuxO-400 for their response to 5 mM H2O2 in 0.1 M PBS (pH = 7.0) solution. The response curve of NC/CuxO-300 significantly deviated from those of the other contrast groups, indicating its superior H2O2 sensing potential. Paticularly, the modulation effect from the addition of CB was investigated. Pure Cu-MOF was used as the precusor of another contrast transducer material, named as NC/CuxO-300 (w/o CB) (Fig. 4B), which was prepared via completely indentical conditions except for the starting precusor. When CB was added (Fig. 4C), the faradaic current sharply increased due to the enhanced electrochemical reactions promoted by the catalyst interface. Hence, the advantages of the CB-induced catalyst modulation are as follows: (1) CB supplemented the inadequate source of certain elements, like C and N, enabling controllable degrees of conductivity, defect abundance and heteroatom doping towards the substrate matrix. An important prerequisite was that, in our proposal, homogeneous distributions of the elements in the substrate were guaranteed through atomic migration during the pyrolysis process. (2) CB effectively modulated the redox environment for the genaration of catalytic units, and meanwhile facilitated the synchronous evolution of their chemical states and morphology. As a result, the cathodic peak current intensity at −0.35 V substantially increased in the presence of H2O2. In comparison with the derivatives directly obtained from the pure MOF precursor in a previous study27 and our contrast group, CB assisted the further modulation of the catalyst's evolution and provided us a new dimension to adjust the performance of the transducer material. In addition, the CVs of the NC/CuxO-300 modified electrode at different scan rates from 10 to 100 mV s−1 were examined (Fig. S5†). With a faster scan rate applied, both the anodic and cathodic peak current linearly increased with the scan rate, suggesting a surface adsorption-controlled electrochemical process.28 The linear calibrations of the peak current intensities were Ipa (μA) = 1.04 + 4.89 V (mV s−1), R2 = 0.999; and Ipc (μA) = −0.49 + 0.77 V (mV s−1), R2 = 0.994, respectively. It was concluded that the well-modulated catalytic centers as well as the supporting substrate codetermined the essential conditions, such as the redox-alternating accessibility and efficient electron transfer, for highly active electrochemical catalysis.
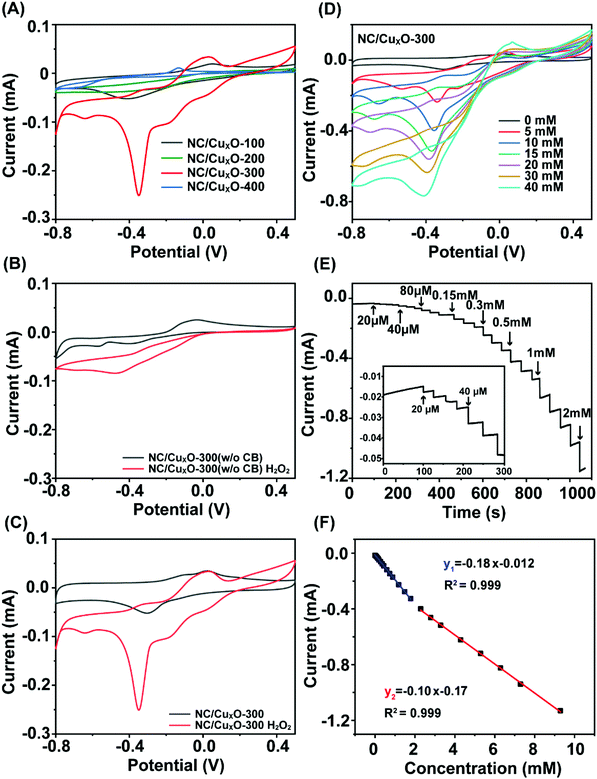 |
| Fig. 4 (A) CVs of NC/CuxO-100, NC/CuxO-200, NC/CuxO-300 and NC/CuxO-400 in 0.1 M PBS (pH = 7.0) solution in the presence of 5 mM H2O2 with a typical scan rate of 10 mV s−1. Tranducer materials prepared (B) without CB or (C) with CB and their CV responses to 5 mM H2O2. (D) CVs of NC/CuxO-300 at different concentrations of H2O2. (E) Amperometric responses of NC/CuxO-300 to the successive addition of H2O2 in N2-saturated 0.1 M PBS solution at −0.3 V vs. SCE. (F) The corresponding calibration curve of (E). | |
Sensing performance based on peroxidase-like activity
Considering the structural properties and the corresponding electrochemical behavior of NC/CuxO-300, the modulated ratio of the mixed-valent CuxO provided the basis of highly active transducing while the N-doped carbon substrate with a large number of defects assisted efficient electron transfer. Therefore, an overpotential-amplified synergetic peroxidase-like mechanism29,30 was speculated, detailed as follows:
Afterwards, this transducer interface fabricated with NC/CuxO-300 was evaluated for the ability of H2O2 detection. Although all the NC/CuxO-T electrodes exhibited obviously enhanced H2O2 reduction peaks with higher concentrations of H2O2 (Fig. S4†), this NC/CuxO-300 transducer material synthesized through optimized multi-modulation demonstrated superior performance, as shown in Fig. 4D. Thanks to the finely controlled ratio of the different redox-states in the CuxO centers, the reduction current intensity reached the milliampere level in response to 40 mM H2O2 at a scan rate of 10 mV s−1. Moreover, real-time amperometric detection was performed at a constant potential of −0.3 V with the stepwise addition of different concentrations, as shown in Fig. 4E. The inset shows the zoomed-in variations of the current responses with successive additions of H2O2 with a concentration as low as 20 μM. The corresponding calibration lines are presented in Fig. 4F. Two distinct linear ranges were noticed in the low concentration (0.02–1.79 mM) and medium concentration (2.29–9.29 mM) periods with regression equations of I (mA) = −0.18 Clow (mM) − 0.012 (R2 = 0.999) and I (mA) = −0.10 Cmedium (mM) − 0.17 (R2 = 0.999), respectively. Calculated with the equation LOD = 3σ/Sd, the limit of detection for this NC/CuxO-300 transducing surface was as low as 0.26 μM. In comparison with copper oxide-based H2O2 sensors summarized in Table S1,† this electrochemically active interface was able to provide competitive performance for trace H2O2 quantification. Besides, we tested the selectivity of this NC/CuxO-300 transducer by adding a few common physiological interferents (Fig. 5). The observations suggested proper selectivity of this catalyst towards the electrochemical reduction of H2O2. After continuous detection for a long period, this sensor maintained its high catalytic activity without material detaching.
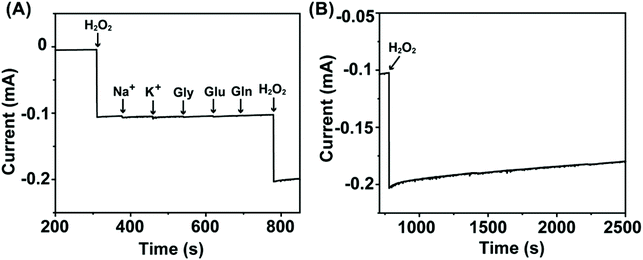 |
| Fig. 5 (A) Amperometric response of the NC/CuxO-300 transducer with the successive addition of 1 mM H2O2, NaCl, KCl, glycine, glucose and glutamine. (B) Amperometric response with the addition of 1 mM H2O2 for the long-term running stability examination. | |
Conclusion
In summary, a CB-supported Cu-MOF nanocomposite (CB@Cu-MOF) prepared feasibly at room temperature was explored as a self-modulated precusor for the downstream synthesis of electrochemial peroxidase-mimic catalysts. By monitoring the temperature-modulated product series, the evolutionary route from this composite precusor towards highly dispersed CuxO-decorated carbon-based transducing materials was intensively investigated. Therefore, a finely optimized catalyst NC/CuxO-300, of which the substrate possessed abundant defects and homogeneous N-doping, while the functional catalytic units of CuxO owned a controllable redox-state allocation, was applied to fabricate a sensitive interface for H2O2 detection. Due to the proper tailoring of the aforementioned structural properties, a proof-of-concept sensor was capable of offering a low detection limit as well as wide linear ranges. We envision that our study can shed light on an effective and accessible strategy for the mass production of well-modulated proprietary catalysts, further promoting their practical applications.
Conflicts of interest
There are no conflicts to declare.
Acknowledgements
The authors greatly acknowledge the financial support from the National Natural Science Foundation of China (No. 62001224), the Natural Science Foundation of Jiangsu Province (No. BK20190457), the 69th batch of China Postdoctoral Science Foundation (No. 2021M691600), the “Overseas Academic Partnership Program” of Nanjing University of Technology (2019), and the Sino-French international research network “New nanostructured materials and biomaterials for renewable electrical energy sources”.
References
- C. Li, R. Wu, J. Zou, T. Zhang, S. Zhang, Z. Zhang, X. Hu, Y. Yan and X. Ling, MNPs@anionic MOFs/ERGO with the size selectivity for the electrochemical determination of H2O2 released from living cells, Biosens. Bioelectron., 2018, 116, 81–88 CrossRef CAS.
- H. Sies, Hydrogen peroxide as a central redox signaling molecule in physiological oxidative stress: Oxidative eustress, Redox Biol., 2017, 11, 613–619 CrossRef CAS.
- N. Thomas, D. D. Dionysiou and S. C. Pillai, Heterogeneous Fenton catalysts: A review of recent advances, J. Hazard. Mater., 2021, 404, 124082 CrossRef CAS PubMed.
- R. Kour, S. Arya, S.-J. Young, V. Gupta, P. Bandhoria and A. Khosla, Review-Recent Advances in Carbon Nanomaterials as Electrochemical Biosensors, J. Electrochem. Soc., 2020, 167(3), 037555 CrossRef CAS.
- H. Sun, Y. Zhou, J. Ren and X. Qu, Carbon Nanozymes: Enzymatic Properties, Catalytic Mechanism, and Applications, Angew. Chem., Int. Ed., 2018, 57(30), 9224–9237 CrossRef CAS PubMed.
- J. M. George, A. Antony and B. Mathew, Metal oxide nanoparticles in electrochemical sensing and biosensing: a review, Microchim. Acta, 2018, 185(7), 358 CrossRef.
- D. Li, X. Zu, D. Ao, Q. Tang, Y. Fu, Y. Guo, K. Bilawal, M. B. Faheem, L. Li, S. Li and Y. Tang, High humidity enhanced surface acoustic wave (SAW) H2S sensors based on sol–gel CuO films, Sens. Actuators, B, 2019, 294, 55–61 CrossRef CAS.
- C. Zhao, X. Wu, P. Li, C. Zhao and X. Qian, Hydrothermal deposition of CuO/rGO/Cu2O nanocomposite on copper foil for sensitive nonenzymatic voltammetric determination of glucose and hydrogen peroxide, Microchim. Acta, 2017, 184(7), 2341–2348 CrossRef CAS.
- J. Wang, J. Ma, X. Li, Y. Li, G. Zhang, F. Zhang and X. Fan, Cu2O mesoporous spheres with a high internal diffusion capacity and improved catalytic ability for the aza-Henry reaction driven by visible light, Chem. Commun., 2014, 50(91), 14237–14240 RSC.
- S. Karthikeyan, S. Kumar, L. J. Durndell, M. A. Isaacs, C. M. A. Parlett, B. Coulson, R. E. Douthwaite, Z. Jiang, K. Wilson and A. F. Lee, Size-Dependent Visible Light Photocatalytic Performance of Cu2O Nanocubes, ChemCatChem, 2018, 10(16), 3554–3563 CrossRef CAS.
- Y. Zhang, Q. Wang, D. Liu, Q. Wang, T. Li and Z. Wang, Cu2O-BiOI isotype (p-p) heterojunction: Boosted visible-light-driven photoelectrochemical activity for non-enzymatic H2O2 sensing, Appl. Surf. Sci., 2020, 521, 146434 CrossRef CAS.
- X. Mo, J. Hu, H. Shen, L. Shui, D. Shu, J. He, G. He, Y. Wang, W. Li and Q. He, Surface modification of micro-sized CuO by in situ-growing heterojunctions CuO/Cu2O and CuO/Cu2O/Cu: effect on surface charges and photogenerated carrier lifetime, Appl. Phys. A, 2018, 124(10), 719 CrossRef.
- Y. Zhu, Z. Xu, K. Yan, H. Zhao and J. Zhang, One-Step Synthesis of CuO–Cu2O Heterojunction by Flame Spray Pyrolysis for Cathodic Photoelectrochemical Sensing of l-Cysteine, ACS Appl. Mater. Interfaces, 2017, 9(46), 40452–40460 CrossRef CAS.
- Q. Zhang, M. Li, Z. Wang, C. Qin, M. Zhang and Y. Li, Porous CuxO/Ag2O (x = 1, 2) nanowires anodized on nanoporous Cu-Ag bimetal network as a self-supported flexible electrode for glucose sensing, Appl. Surf. Sci., 2020, 515, 146062 CrossRef CAS.
- J. Kong, Z. Rui, S. Liu, H. Liu and H. Ji, Homeostasis in CuxO/SrTiO3 hybrid allows highly active and stable visible light photocatalytic performance, Chem. Commun., 2017, 53(91), 12329–12332 RSC.
- S. Li, X. Liu, H. Chai and Y. Huang, Recent advances in the construction and analytical applications of metal-organic frameworks-based nanozymes, TrAC, Trends Anal. Chem., 2018, 105, 391–403 CrossRef CAS.
- L. Liu, Y. Zhou, S. Liu and M. Xu, The Applications of Metal-Organic Frameworks in Electrochemical Sensors, ChemElectroChem, 2018, 5(1), 6–19 CrossRef CAS.
- C.-S. Liu, J. Li and H. Pang, Metal-organic framework-based materials as an emerging platform for advanced electrochemical sensing, Coord. Chem. Rev., 2020, 410, 213222 CrossRef CAS.
- J. Ji, S. Y. Ko, K. M. Choi and Y. Kwon, Hydrogen peroxide sensor using the biomimetic structure of peroxidase including a metal organic framework, Appl. Surf. Sci., 2021, 554, 148786 CrossRef.
- G. Zang, W. Hao, X. Li, S. Huang, J. Gan, Z. Luo and Y. Zhang, Copper nanowires-MOFs-graphene oxide hybrid nanocomposite targeting glucose electro-oxidation in neutral medium, Electrochim. Acta, 2018, 277, 176–184 CrossRef CAS.
- X. Yi, X. He, F. Yin, B. Chen, G. Li and H. Yin, Co-CoO-Co3O4/N-doped carbon derived from metal-organic framework: The addition of carbon black for boosting oxygen electrocatalysis and Zn-Air battery, Electrochim. Acta, 2019, 295, 966–977 CrossRef CAS.
- S. Duan, L. Wu, J. Li, Y. Huang, X. Tan, T. Wen, T. Hayat, A. Alsaedi and X. Wang, Two-dimensional copper-based metal–organic frameworks nano-sheets composites: One-step synthesis and highly efficient U(VI) immobilization, J. Hazard. Mater., 2019, 373, 580–590 CrossRef CAS.
- Y. Ma, L. Wang, J. Ma, H. Wang, C. Zhang, H. Deng and H. He, Investigation into the Enhanced Catalytic Oxidation of o-Xylene over MOF-Derived Co3O4 with Different Shapes: The Role of Surface Twofold-Coordinate Lattice Oxygen (O2f), ACS Catal., 2021, 11(11), 6614–6625 CrossRef CAS.
- J. Li, W.-L. Xin, Y.-X. Dai, G. Shu, X.-J. Zhang, R. S. Marks, S. Cosnier and D. Shan, Postmodulation of the Metal-Organic Framework Precursor toward the Vacancy-Rich CuxO Transducer for Sensitivity Boost: Synthesis, Catalysis, and H2O2 Sensing, Anal. Chem., 2021, 93(32), 11066–11071 CrossRef CAS PubMed.
- W. Zhen, W. Jiao, Y. Wu, H. Jing and G. Lu, The role of a metallic copper interlayer during visible photocatalytic hydrogen generation over a Cu/Cu2O/Cu/TiO2 catalyst, Catal. Sci. Technol., 2017, 7(21), 5028–5037 RSC.
- Y. Wang, Y. Lü, W. Zhan, Z. Xie, Q. Kuang and L. Zheng, Synthesis of porous Cu2O/CuO cages using Cu-based metal–organic frameworks as templates and their gas-sensing properties, J. Mater. Chem. A, 2015, 3(24), 12796–12803 RSC.
- Q. Wu, L. He, Z. W. Jiang, Y. Li, Z. M. Cao, C. Z. Huang and Y. F. Li, CuO nanoparticles derived from metal-organic gel with excellent electrocatalytic and peroxidase-mimicking activities for glucose and cholesterol detection, Biosens. Bioelectron., 2019, 145, 111704 CrossRef CAS PubMed.
- M. Zhang, J. Zheng, J. Wang, J. Xu, T. Hayat and N. S. Alharbi, Direct electrochemistry of cytochrome c immobilized on one dimensional Au nanoparticles functionalized magnetic N-doped carbon nanotubes and its application for the detection of H2O2, Sens. Actuators, B, 2019, 282, 85–95 CrossRef CAS.
- J. Wu, W. Lv, Q. Yang, H. Li and F. Li, Label-free homogeneous electrochemical detection of MicroRNA based on target-induced anti-shielding against the catalytic activity of two-dimension nanozyme, Biosens. Bioelectron., 2021, 171, 112707 CrossRef CAS PubMed.
- Y. Zhu, R. Zhu, Y. Xi, J. Zhu, G. Zhu and H. He, Strategies for enhancing the heterogeneous Fenton catalytic reactivity: A review, Appl. Catal., B, 2019, 255, 117739 CrossRef CAS.
Footnote |
† Electronic supplementary information (ESI) available. See DOI: 10.1039/d1an01864g |
|
This journal is © The Royal Society of Chemistry 2022 |