DOI:
10.1039/D1TC01027A
(Paper)
J. Mater. Chem. C, 2021,
9, 10749-10758
Dysprosium–dianthracene framework showing thermo-responsive magnetic and luminescence properties†
Received
5th March 2021
, Accepted 6th May 2021
First published on 6th May 2021
Abstract
Metal–organic frameworks showing stimuli-responsive magneto-optical properties are of intensive current interest for potential applications in spintronics and molecular devices. By incorporating a thermo-responsive dianthracene component into a lanthanide phosphonate framework, we successfully obtained compounds Ln2(amp2H2)2(C2O4)(H2O)2·xH2O (MDAF-4Ln, Ln = Dy, x = 10; Gd, x = 9; Er, x = 7; and amp2H4 = pre-photodimerized 9-anthrylmethylphosphonic acid). These compounds are isostructural showing three-dimensional open framework structures composed of Ln-chains and dianthracence linkages. Within the chain, the dimers of {Ln2O2(OPO)2} are connected by oxalate anions. The MOFs generate channels along the a-axis which are hydrophilic lined with protonated phosphonate moieties, and hence exhibit high affinity towards adsorption of water and ammonia. Thermogravimetric analysis and differential scanning calorimetry (DSC) measurements revealed that MDAF-4Ln experienced two consecutive phase transitions: first, the loss of all lattice and coordinated water molecules and then, the dissociation of amp2H22−. Detailed studies on MDAF-4Dy demonstrated that the thermo-induced structural transformations were accompanied by significant changes in its single-molecule magnetic behavior and luminescence properties.
Introduction
Metal–organic frameworks (MOFs) with responsive magneto-optical bifunctions are highly desired not only for developing multifunctional materials but also for applications in spintronics and molecular devices.1 Lanthanide-based MOFs are appealing in this context because lanthanide ions possess significant single-ion magnetic anisotropy arising from strong spin–orbit coupling and crystal-field effects.2 They also exhibit well-resolved characteristic photoluminescence upon sensitization by an organic antenna.3
The intrinsic strong magnetic anisotropy of lanthanide ions has enabled the construction of numerous single-molecule magnets (SMMs) based on lanthanide complexes,4,5 especially the mononuclear species with highly symmetric electrostatic environments,6–10 the blocking temperature of which reached near or even above the temperature of liquid nitrogen.11–13 By contrast, the magnetic performance of Ln-MOFs is far less exciting.14 Apart from the difficulties in controlling the coordination geometries of lanthanide ions in MOFs, organizing lanthanide ions in the framework structure often leads to non-parallel arrangements of the magnetic easy-axes which largely reduce the overall magnetic anisotropy.15 Therefore, only few Ln-MOFs were reported showing SMM behaviour under zero external field (Table S1, ESI†).16–28 Nevertheless, Ln-MOFs have their own advantages over mononuclear or cluster species. They could create arrays of SMM species that are well separated in space. The structural rigidity of MOFs has the advantage of minimizing the spin-phonon fast-relaxation mechanism, which is important for high-performance SMMs.28 Moreover, the magnetic dynamics of Ln-SMMs are very sensitive to subtle structural changes including the coordination geometry, crystal packing and hydrogen bond network.29 The magnetic properties of Ln-MOFs can be tuned by changing the guest molecules inside the pore and modifying the organic linkers in the framework. Indeed, Ln-MOFs with their SMM behaviour responsive to guest exchange and de-solvation have been described.23–27 Regarding the organic linkers, Yamashita and co-workers reported a 2D Dy-dithienylethane compound showing light-triggered changes of SMM properties due to the ring-open and ring-close isomerism.30
Ln-MOFs exhibiting luminescence and SMM bifunctions were much less explored.22,23,31–33 Simultaneous manipulation of both luminescence and SMM behaviour in Ln-MOFs remains to be a formidable challenge since the characteristic luminescence of lanthanide ions is insensitive to external stimuli. The only example, as far as we are aware, was given by Ohkoshi, Chorazy and co-workers.26 They found that the coordination geometry of DyIII in a cyanide-bridged DyIIICoIII framework changed upon dehydration from the D4d to D3h symmetry. This change caused the switch-on of SMM properties and the shift of luminescent colour from nearly white to deep yellow.
Our strategy to construct Ln-MOFs with switchable magneto-optical bifunctions uses stimuli-responsive organic ligands as linkers in the skeleton. To this end, dianthracene derivatives are ideal candidates because they can de-dimerize upon thermal treatment or UV light irradiation with change in luminescence.34–37 Considering that the organophosphonate ligands have strong binding capabilities toward lanthanide ions forming MOFs embedding lanthanide clusters, chains or layers,38–46 herein, we choose a pre-photodimerized 9-anthrylmethylphosphonic acid (amp2H4) as a linker to build new Ln-MOFs that possess magneto-optical bifunctions responsive to external stimuli. Compounds Ln2(amp2H2)2(C2O4)(H2O)2·xH2O (MDAF-4Ln, Ln = Dy, x = 10; Gd, x = 9; Er, x = 7; MDAF means Metal DiAnthracene Framework) were successfully isolated under hydrothermal conditions. They are isostructural showing three-dimensional open framework structures composed of Ln-chains and dianthracence linkages. Within the chain, the dimers of {Ln2O2(OPO)2} are bridged by oxalate anions. Magnetic studies revealed dominant antiferromagnetic (AF) exchange coupling between the magnetic centres within the chain, which was supported by theoretical calculations. Interestingly, MDAF-4Dy showed slow magnetization relaxation under zero static field, characteristic of the SMM behaviour. Theoretical calculations confirmed that the ground Kramers doublet (KD) of an individual DyIII fragment is mostly composed of the mJ = ±15/2 state. For MDAF-4Er, field-induced SMM behaviour was observed. Compound MDAF-4Dy was further subjected to a detailed magnetic and luminescence study upon thermal treatment. The results demonstrated that it experienced thermo-driven two consecutive phase transitions: first, the loss of all lattice and coordination water molecules and then, the dissociation of amp2H22−. The phase transitions were accompanied by dramatic changes in luminescence and magnetic dynamics. To our knowledge, only one Ln-dianthracene MOF was previously reported in the literature without concerning the magnetic or luminescence properties.35 Therefore, compounds MDAF-4Ln are the first examples of Ln-dianthracene MOFs with magneto-optical bifunctions. Particularly, MDAF-4Dy is the first example of Ln-dianthracene MOFs showing thermo-responsive magnetic dynamics and luminescence properties.
Experimental section
Materials and instrumentation
Photodimerized 9-anthrylmethylphosphonic acid (amp2H4) was synthesized according to the literature method.36 All the starting materials were obtained from commercial sources and used without further purification. Elemental analysis for C and H was performed using a PerkinElmer 240C elemental analyzer. The infrared spectra were recorded using a Bruker Tensor 27 spectrometer in the region of 4000–400 cm−1. Thermogravimetric analysis was performed using a Mettler Toledo TGA/DSC 1 instrument in the range of 30–600 °C under a nitrogen flow at a heating rate of 5 °C min−1. Differential scanning calorimetry (DSC) measurements were conducted using a METTLER DSC823e/200 under N2 atmosphere. The powder XRD patterns were recorded using a BRUKER D8 Advance X-ray diffractometer with Cu Kα radiation (λ = 1.5406 Å). Single-crystal diffraction data were collected at 193 K using a Bruker D8 Venture. The UV-vis spectra were recorded using a PerkinElmer Lambda 950 UV-vis spectrometer. The steady fluorescence spectra were obtained using a PerkinElmer Spectrofluorometer LS55. Time-resolved fluorescence and quantum yield were measured using a Fluorolog TCSPC spectrofluorometer (Horiba Scientific). The ac and dc magnetization data were obtained on polycrystalline samples using Quantum Design VSM SQUID magnetometers. The data were corrected for the diamagnetic contributions of the compounds obtained from Pascal's constants and for the sample holders.47
Synthesis of Ln2(amp2H2)2(C2O4)(H2O)2·xH2O (MDAF-4Ln).
Compounds MDAF-4Ln (Ln = Dy, Gd, Er) were synthesized similarly except using a different LnCl3·6H2O. A typical procedure for the synthesis of Dy2(amp2H2)2(C2O4)(H2O)2·10H2O (MDAF-4Dy) is given below. A mixture of amp2H4 (13.6 mg, 0.025 mmol), DyCl3·6H2O (18.8 mg, 0.05 mmol) and H2C2O4 (3.1 mg, 0.025 mmol) in 6 mL of water was sealed in a Teflon-lined autoclave and heated under autogenous pressure at 95 °C for 2 days. After cooling to room temperature, rhombic colourless crystals of MDAF-4Dy were collected, washed with water and then air-dried. The purity was confirmed by the PXRD measurement (Fig. S1 in ESI†). Yield: 47.3% (based on amp2H4). Elemental anal. calcd (%) for Dy2C62H72O28P4: C, 44.37; H, 4.08. Found (%): C, 44.19; H, 4.16. IR (KBr, cm−1): 3606(m), 3428(m), 3062(w), 3033(w), 2923(w), 1643(s), 1477(m), 1452(m), 1427(w), 1346(w), 1313(s), 1255(w), 1236(w), 1190(m), 1126(m), 1060(s), 1016(m), 966(w), 935(w), 798(w), 767(m), 733(w), 692(m), 646(m), 619(w), 570(m), 536(m), 486(w), 443(m).
For Gd2(amp2H2)2(C2O4)(H2O)2·9H2O (MDAF-4Gd), yield: 40.5% (based on amp2H4). Elemental anal. calcd (%) for Gd2C62H70O27P4: C, 44.17; H, 4.19. Found (%): C, 44.35; H, 4.24. IR (KBr, cm−1): 3605(m), 3428(m), 3063(w), 3033(w), 2924(w), 1637(s), 1477(m), 1452(m), 1427(w), 1348(w), 1312(s), 1255(w), 1236(w), 1189(m), 1126(m), 1061(s), 1015(m), 966(w), 935(w), 799(w), 768(m), 734(w), 691(m), 646(m), 619(w), 571(m), 526(m), 486(w), 448(m).
For Er2(amp2H2)2(C2O4)(H2O)2·7H2O (MDAF-4Er), yield: 42.1% (based on amp2H4). Elemental anal. calcd (%) for Er2C62H66O25P4: C, 44.60; H, 3.98. Found (%): C, 44.52; H, 3.66. IR (KBr, cm−1): 3606(m), 3428(m), 3062(w), 3033(w), 2924(w), 1637(s), 1477(m), 1452(m), 1427(w), 1350(w), 1313(s), 1255(w), 1236(w), 1190(m), 1126(m), 1060(s), 1016(m), 966(w), 935(w), 798(w), 767(m), 733(w), 691(m), 646(m), 619(w), 570(m), 528(m), 487(w), 445(m).
Single-crystal structure determination
Suitable single crystals of MDAF-4Ln were selected and mounted on the loops. The single-crystal data sets were collected at 193 K using a Bruker D8 Venture diffractometer with Mo-Kα radiation (λ = 0.71073 Å) for MDAF-4Dy, MDAF-4Gd and MDAF-4Er. The numbers of collected and observed independent [I > 2σ(I)] reflections were 12
449 and 6160 (Rint = 0.0407) for MDAF-4Dy, 10
334 and 5906 (Rint = 0.0357) for MDAF-4Gd, and 10656 and 5780 (Rint = 0.0591) for MDAF-4Er, respectively. The data were integrated using the Siemens SAINT program,48 and adsorption corrections were applied. The structures were solved by a direct method and refined on F2 by full-matrix least-squares using SHELXTL.49 All non-hydrogen atoms were refined using anisotropic thermal parameters. All hydrogen atoms bound to carbon were refined isotropically in the riding mode. The hydrogen atoms of the coordinated water molecule (O1W) were detected via experimental electron density. The O–H bond distances and the H–O–H angle were restricted, and then, the water molecule was made into a rigid group using the AFIX 6 instruction. The O3 and O6 atoms from the phosphonate groups are protonated due to the elongated P–O bonds and charge balance. The AFIX 83 instruction was used to add the hydrogen atoms to the O3 and O6 atoms. In all cases, residual electron densities in the solvent-accessible void were treated with the PLATON/SQUEEZE program50 owing to the heavily disordered solvent molecules. The solvent-accessible voids calculated from the SQUEEZE results are 429 (MDAF-4Dy), 418 (MDAF-4Gd), and 398 (MDAF-4Er) Å3 per unit, which can amount to around ten lattice water molecules (ca. 40 Å3 per H2O).
Results and discussion
Crystal Structure of MDAF-4Ln
Compounds MDAF-4Ln are isostructural as confirmed by their PXRD patterns and single crystal data (Table 1, Fig. S1, ESI†). Hence, MDAF-4Dy is selected as a representative for a detailed structural description. Dy2(amp2H2)2(C2O4)(H2O)2·10H2O (MDAF-4Dy) crystallises in the triclinic space group P
and the asymmetric unit consists of one DyIII ion, two kinds of amp2H22− ligands (each with half occupancy), 0.5 C2O42−, one coordinated water molecule and five lattice water molecules (Fig. S3, ESI†). The lattice water molecules were heavily disordered and hence were treated with the PLATON/SQUEEZE program. The number of lattice water molecules was determined based on elemental and thermal analyses. As shown in Fig. 1a, the DyIII ion is eight-coordinated by five O atoms from four amp2H22− ligands (O1A, O2A, O2, O4A, and O5), two O atoms from the chelated C2O42− (O7, O8B), and one O atom from the coordinated water molecule (O1W). The Dy–O bond distances are in the range from 2.288(3) to 2.568(3) Å, and the O–Dy–O bond angles vary from 59.03(10) to 152.38(11)° (Table S2, ESI†). The geometry of the {DyO8} core is close to a distorted triangular dodecahedron with a D2d symmetry, according to the continuous shape measure (CShM = 0.944)51 analysis (Table S3, ESI†).
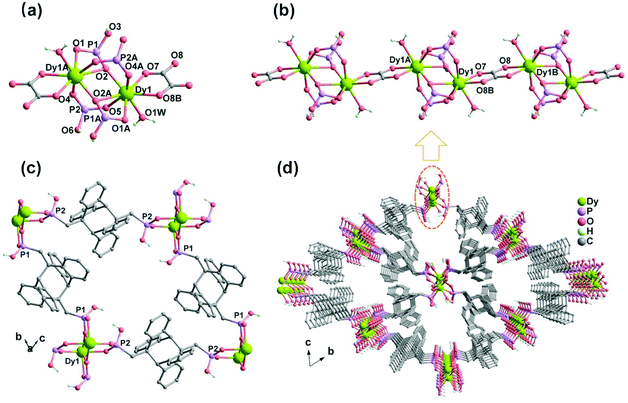 |
| Fig. 1 Crystal structure of MDAF-4Dy. (a) Dimeric units with atomic numbering scheme; (b) a fragment of the chain composed of dysprosium dimeric units and oxalate linkages; (c) rhombic window of the Dy2-diantracene layer; (d) open framework structure viewed along the a-axis. The disordered lattice water molecules are squeezed. All H atoms except those in coordinated water and phosphonate oxygen atoms are omitted for clarity. | |
Two crystallographically different amp2H22− ligands are found. One serves as a hexadentate ligand with each phosphonate group providing two oxygen donors (O1, O2) to chelate and bridge the Dy atoms (Scheme 1a). The other acts as a tetradentate ligand with each phosphonate group offering two oxygen donors (O4 and O5) to bridge the Dy atoms (Scheme 1b). The remaining phosphonate oxygen atoms (O3 and O6) are protonated. Consequently, the equivalent Dy atoms are linked by two μ3-O2 and two O4–P2–O5 units forming {Dy2O2(OPO)2} dimers (Fig. 1a). These dimers are connected by oxalate ligands forming infinite chains running along the a-axis (Fig. 1b). The Dy⋯Dy distances over the μ3-O2 and oxalate bridges within the chain are 3.891 and 6.169 Å, respectively. The dimers of {Dy2O2(OPO)2} are also connected by dianthracene groups, forming layers in the bc plane which contain rhombic windows composed of four {Dy2O2(OPO)2} dimers and four amp2H22− ligands (Fig. 1c). The shortest Dy⋯Dy distance over the dianthracene ligand is 13.742 Å. The layers are cross-linked by oxalate ligands into a 3D open framework structure with channels generated along the a-axis (Fig. 1d). The lattice water molecules reside in the channels. Notably, all the protonated phosphonate oxygen atoms line on the channel wall.
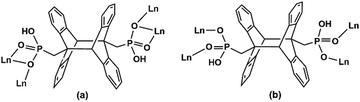 |
| Scheme 1 Coordination modes of amp2H22−. | |
Compounds MDAF-4Gd and MDAF-4Er also crystallize in the triclinic system space group P
(Table 1). They show similar 3D framework structures composed of Ln-chains and amp2H22− linkers. The unit cell volume follows the order: MDAF-4Gd > MDAF-4Dy > MDAF-4Er, attributed to the lanthanide contraction effect. The Ln–O distances and O–Ln–O angles are in the ranges of 2.330(3)–2.578(3) Å and 58.67(10)–152.41(11) ° for MDAF-4Gd, and 2.275(4)–2.534(4) Å and 59.72(14)–151.64(14) ° for MDAF-4Er, respectively (Table 2).
Table 1 Crystallographic data for MDAF-4Dy, MDAF-4Gd and MDAF-4Er
|
MDAF-4Dy
|
MDAF-4Gd
|
MDAF-4Er
|
R
1 = Σ||Fo| − |Fc||/Σ|Fo|, wR2 = [Σw(Fo2 − Fc2)2/Σw(Fo2)2]1/2.
|
Empirical formula |
C31H26DyO9P2 |
C31H26GdO9P2 |
C31H26ErO9P2 |
Temperature (K) |
193(2) |
193(2) |
193(2) |
F
W
|
766.96 |
761.71 |
771.72 |
Crystal system |
Triclinic |
Triclinic |
Triclinic |
Space group |
P![[1 with combining macron]](https://www.rsc.org/images/entities/char_0031_0304.gif) |
P![[1 with combining macron]](https://www.rsc.org/images/entities/char_0031_0304.gif) |
P![[1 with combining macron]](https://www.rsc.org/images/entities/char_0031_0304.gif) |
a (Å) |
9.5729(6) |
9.6423(8) |
9.4986(19) |
b (Å) |
13.826(1) |
13.8366(13) |
13.736(3) |
c (Å) |
14.8032(10) |
14.8249(13) |
14.760(3) |
α (°) |
63.398(2) |
63.687(2) |
63.610(5) |
β (°) |
75.488(2) |
75.327(2) |
75.449(6) |
γ (°) |
88.549(2) |
88.631(3) |
88.768(6) |
V (Å3) |
1687.4 (2) |
1706.2(3) |
1660.8 (6) |
Z
|
2 |
2 |
2 |
ρ
calcd (g cm−3) |
1.509 |
1.483 |
1.543 |
F (000) |
760.0 |
756.0 |
764.0 |
Goodness of fit |
1.024 |
1.037 |
1.032 |
R
1, wR2 [I > 2σ(I)]a |
0.0334, 0.0732 |
0.0358, 0.0820 |
0.0406, 0.1011 |
R
1, wR2 (all data)a |
0.0413, 0.0771 |
0.0432, 0.0847 |
0.0458, 0.1041 |
CCDC number |
2065604
|
2065605
|
2065606
|
Table 2 Selected structural parameters for compounds MDAF-4Ln
|
MDAF-4Dy
|
MDAF-4Gd
|
MDAF-4Er
|
C–C (central) [Å] |
1.609, 1.636 |
1.613, 1.651 |
1.608, 1.642 |
Ln–O (P) [Å] |
2.288–2.568 |
2.330–2.578 |
2.275–2.534 |
Ln–O (C2O42−) [Å] |
2.352, 2.404 |
2.375, 2.431 |
2.320, 2.389 |
Ln–O (H2O) [Å] |
2.446 |
2.461 |
2.418 |
O–Ln–O (°) |
59.03–152.38 |
58.67–152.41 |
59.72–151.64 |
Ln–O–Ln (°) |
105.92 |
106.17 |
107.28 |
Ln⋯Ln (within dimer) [Å] |
3.891 |
3.929 |
3.876 |
Ln⋯Ln (over dimers) [Å] |
6.169 |
6.222 |
6.114 |
Ln⋯Ln (over amp2H22−) [Å] |
13.742–15.595 |
13.773–15.651 |
13.645–15.568 |
It is worth mentioning that a few lanthanide oxalatophosphonates with 3D framework structures were previously reported. They are composed of Ln-oxalate chains52–54 or layers with phosphonate linkers,55,56 or lanthanide phosphonate layers with oxalate pillars.57 These structures are completely different from those of MDAF-4Ln, where the lanthanide chains containing {Ln2O2(OPO)2} dimers and oxalate linkages are isolated by the bulky dianthracene groups. Hence, MDAF-4Ln provide a new type of structure among lanthanide oxalatophosphonates. In addition, this structure is also distinct from the known Ln-dianthracene MOF (CD-MOF-161)35 in which dimers of {Yb2O(OCO)2} are interconnected by dianthracene dicarboxylate linkers.
Gas sorption properties
Knowing that the structures of MDAF-4Ln are porous with the pore-walls lined with hydrophilic P–OH moieties, we conjecture that the activated sample of MDAF-4Ln would show affinity to alkaline gas. To validate this assumption, MDAF-4Dy, activated at 100 °C under vacuum for 4 h, was chosen for gas sorption measurements including N2, H2, CO2, NH3 and H2O. The N2 adsorption/desorption at 77 K showed a type II isotherm with a loading of 3.98 cm3 g−1 at P/P0 = 0.98 (Fig. S4, ESI†), indicating that the interactions between nitrogen and the framework are very weak. The activated MDAF-4Dy can also adsorb H2 at 77 K with hysteresis, but the capacities were low, with the uptake of 1.16 cm3 g−1 at P/P0 = 0.98 (Fig. S4, ESI†). Similarly, the CO2 adsorption at 298 K was not high with a maximum uptake of 26.68 cm3 g−1 at P/P0 = 0.98. By contrast, a rapid and remarkably enhanced adsorption was observed for NH3 at 298 K with the uptakes of 78.37 cm3 g−1 (5.99 mol mol−1) at P/P0 = 0.02 and 129.07 cm3 g−1 (5.76 mmol g−1, 9.87 mol mol−1) at P/P0 = 0.98 (Fig. 2). The adsorption capacity of NH3 is about 5 times higher than that of CO2 at 298 K and is comparable to those found for other MOFs.58 The strong adsorption capability of MDAF-4Dy toward ammonia may be explained by favourable interaction between alkaline NH3 and acidic P–OH on the channel walls, which is supported by the significant hysteresis of the ammonia adsorption/desorption isotherm. After desorption, there remained 68.31 cm3 g−1 (5.22 mol mol−1) of ammonia at P/P0 = 0.001. This value is only slightly lower than the expected value of 6.0 mol mol−1 if the four P–OH groups per molecular formula are each strongly hydrogen-bonded to NH3 and the two vacant sites of the Dy atoms are also filled with NH3.
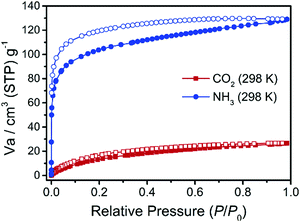 |
| Fig. 2 Adsorption (filled) and desorption (open) isotherms of CO2 and NH3 at 298 K for MDAF-4Dy. | |
Significant hysteresis was also observed for water isotherm. In this case, the adsorption curve shows a gradual increase below P/P0 = 0.09, and then a sharp increase until P/P0 = 0.12, indicating a “gate opening” effect. The total adsorption capacity at P/P0 = 1.01 is 158.24 cm3 g−1 (or 12.10 mmol mmol−1) (Fig. S5, ESI†), in agreement with the presence of two coordinated and ten lattice water molecules in the pristine compound. PXRD measurements confirmed that the original structure of MDAF-4Dy was maintained after activation and adsorption/desorption measurements (Fig. S6, ESI†).
Thermo-induced phase transitions of MDAF-4Ln
Compounds MDAF-4Ln contain lattice and coordinated water molecules as well as a thermo-responsive dianthracene component in the framework skeleton. It is therefore necessary to investigate their thermal properties. Fig. 3 shows the thermogravimetric (TG) and differential scanning calorimetry (DSC) curves of MDAF-4Ln. For MDAF-4Dy, a weight loss of 12.8% is observed on heating to 150 °C, corresponding to the removal of ten lattice and two coordinated water molecules (calcd 12.9%). This is followed by a plateau until 210 °C, above which a steep weight loss is found, indicating the decomposition of the organic components and the collapse of the framework structure. The DSC profile shows an endothermic band in the range 30–150 °C peaking at 100 °C with an enthalpy of 249.4 kJ mol−1 per formula unit (Fig. 3b), in accordance with the release of water molecules. The IR spectra confirmed that the O–H vibration peaks at 3428 cm−1 disappeared at 150 °C (Fig. S7, ESI†). Therefore, the fully dehydrated sample of MDAF-4Dy was obtained after thermal treatment at 150 °C for 10 min, which is named MDAF-4Dy-150. This phase can convert back to the pristine one by immersing in water for 1 d (Fig. S8–S10, ESI†), indicating that the framework structure of MDAF-4Dy is maintained during the dehydration process. Interestingly, an exothermic peak appears at 190 °C in the DSC plot, ascribed to the de-dimerization of the dianthracene ligand. The enthalpy is −109.4 kJ mol−1 per formula unit (or −54.7 kJ mol−1 per amp2H22−). Variable temperature IR spectra revealed that peaks at 1281 and 881 cm−1 emerged above 180 °C, characteristic of C–H vibrations of de-dimerized anthracene rings (Fig. 4).59 The new phase, named MDAF-4Dy-210, was obtained by heating MDAF-4Dy to 210 °C and keeping it at this temperature for 10 min. This new phase shows a different PXRD pattern from that of the pristine one (Fig. S10, ESI†).
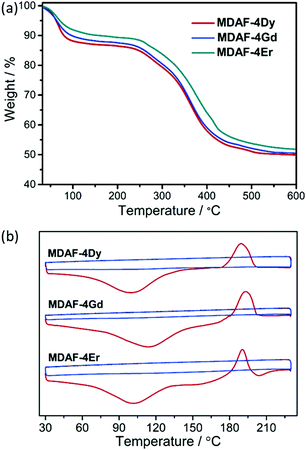 |
| Fig. 3 (a) TG curves and (b) DSC curves of MDAF-4Ln with a heating rate of 5 °C min−1. Colour codes in (b): red for the first cycle, blue for the second cycle. | |
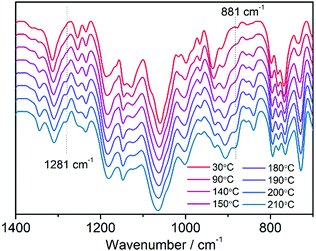 |
| Fig. 4
In situ variable temperature infrared spectra of MDAF-4Dy measured in the range of 30–210 °C. | |
For MDAF-4Gd and MDAF-4Er, their TG and DSC curves are similar to those of MDAF-4Dy. They lost the lattice and coordinated water molecules in the temperature range of 30–150 °C (obs. 11.8% and 9.9%, calcd. 11.7% and 9. 8%) (Fig. 3a). The enthalpies are 170.2 kJ mol−1 for MDAF-4Gd and 141.8 kJ mol−1 for MDAF-4Er per formula unit (Fig. 3b). Upon further heating, an exothermic peak appeared at 193 °C for MDAF-4Gd and at 191 °C for MDAF-4Er, associated with the de-dimerization of the dianthracene ligand. The enthalpies are −107.7 and −105.8 kJ mol−1 per formula unit, respectively, consistent with that for MDAF-4Dy. Apparently, all three compounds of MDAF-4Ln underwent two consecutive phase transitions upon heating: first, the release of all water molecules and then, the de-dimerization of the dianthracene moieties. The thermo-induced phase transformations will cause significant changes to their physical properties.
Thermo-triggered change of photophysical properties
Fig. 5a shows the solid-state UV-vis absorption spectra of MDAF-4Dy, MDAF-4Dy-150 and MDAF-4Dy-210, translated from the diffuse reflectance spectra using the equation F(R) = (1 − R)2/2R. MDAF-4Dy and MDAF-4Dy-150 show several absorption bands at 210–260 nm, 260–300 nm and 300–450 nm, originating from the n → π* and π → π* transitions of the amp2H22− and oxalate ligands (Fig. 5a and Fig. S11, ESI†). While for MDAF-4Dy-210, the peak intensities at the 200–300 nm region are remarkably reduced, which is associated with the de-dimerization of the dianthracene groups. The absorption band at 300–450 nm is also red-shifted to 300–485 nm for MDAF-4Dy-210, in agreement with the higher degree of conjugation of anthracene than dianthracene groups. Besides, they all show additional weak peaks around 756 nm, ascribed to the f–f transition of the DyIII ion from the 6H15/2 to 6F3/2 state.60
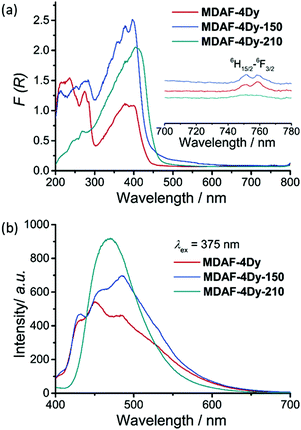 |
| Fig. 5 (a) UV-vis absorption spectra and (b) the steady-state emission spectra excited at 375 nm for MDAF-4Dy, MDAF-4Dy-150 and MDAF-4Dy-210. Inset: An enlarged view of the UV-vis absorption spectra of three compounds at 700–780 nm. | |
Upon excitation at 375 nm, all three compounds show photoluminescence in the visible region. The emission profiles of MDAF-4Dy and MDAF-4Dy-150 are quite similar showing three peaks at 430, 450 and 485 nm (Fig. 5b), attributed to the vibrational manifold of the π* → π transition of dianthracene. The lifetimes are 3.35–4.33 ns and 1.96–2.69 ns for MDAF-4Dy and MDAF-4Dy-150, respectively (Fig. S12, S13 and Table S6, ESI†). The quantum yields (QY) are 1.22% for MDAF-4Dy and 1.49% for MDAF-4Dy-150. The smaller QY of the former could be due to the presence of water molecules in MDAF-4Dy which facilitate vibrational quenching by O–H oscillators. After de-dimerization, MDAF-4Dy-210 shows a red-shift and broad emission peak at 470 nm (QY = 7.11%), attributed to the formation of an excimer of the anthracene groups with staggered π−π stacking interactions (average life time τav = 1.84 ns) (Fig. 5b and Fig. S14, Table S6, ESI†).36,61
For compounds MDAF-4Gd and MDAF-4Er, only the pristine samples were subjected to photophysical property studies. Their absorption spectra in the visible region are similar to those of MDAF-4Dy (Fig. S15 and S16, ESI†). For MDAF-4Er, characteristic Er3+ f–f absorption bands were observed peaking at 488 (4I15/2 → 4F7/2), 521 (4I15/2 → 2H11/2), 542 (4I15/2 → 4S3/2), 653 (4I15/2 → 4F9/2) and 804 nm (4I15/2 → 4I9/2) (Fig. S16a, ESI†).60,62 The emission profiles of the two compounds in the visible region are analogous to those of MDAF-4Dy (Fig. S15b and S16b, ESI†). The lifetimes are 5.31–5.49 ns for MDAF-4Gd, and 4.42–6.33 ns for MDAF-4Er, comparable to those for MDAF-4Dy. The quantum yields are 11.85% for MDAF-4Gd, and 1.11% for MDAF-4Er (Fig. S17, S18 and Table S6, ESI†). As the quantum yields of MDAF-4Dy and MDAF-4Er are much lower than that of MDAF-4Gd in the visible region, an efficient energy transfer from the dianthracene ligand to DyIII or ErIII ion could occur. Indeed, emission in the NIR region was observed for MDAF-4Er around 1536 and 1568 nm (Fig. S19, ESI†), corresponding to the 4I13/2 → 4I15/2 transition of the ErIII ion.
Thermo-triggered change of magnetic properties
The magnetic properties of MDAF-4Ln, MDAF-4Dy-150 and MDAF-4Dy-210 were studied. At 300 K, the χMT values (per Ln2 unit) are 28.70, 28.02 and 28.00 cm3 K mol−1 for MDAF-4Dy, MDAF-4Dy-150 and MDAF-4Dy-210, respectively, 15.70 cm3 K mol−1 for MDAF-4Gd and 22.81 cm3 K mol−1 for MDAF-4Er (Fig. S20–S22, ESI†), which agree well with the spin-only value of 28.34 cm3 K mol−1 expected for two DyIII ions (6H15/2, S = 5/2, L = 5, gJ = 4/3), 15.76 cm3 K mol−1 expected for two GdIII ions (8S7/2, S = 7/2, L = 0, gJ = 2), and 22.96 cm3 K mol−1 for two ErIII ions (4I15/2, S = 3/2, L = 6, gJ = 6/5). The presence of weak antiferromagnetic (AF) interactions between the lanthanide centres in MDAF-4Ln is confirmed by the continuous decreasing of the χMT values of MDAF-4Gd upon cooling with a small negative Weiss constant (−0.79 K). Therefore, the progressive decrease of χMT in MDAF-4Dy and MDAF-4Er upon cooling is due to the combination of the depopulation of the mJ sublevels and weak Ln⋯Ln AF interactions. Furthermore, the unsaturation of the magnetization and the non-superimposition of the M vs. H/T plots support the existence of magnetic anisotropy and/or lower-lying excited states (Fig. S20 and S23, ESI†).63
To examine the magnetic dynamics of MDAF-4Dy before and after thermal treatment, ac susceptibility measurements were performed. For MDAF-4Dy, both the in-phase (χ′) and out-of-phase (χ′′) components are frequency-dependent under zero dc field (Fig. 6a and Fig. S24, ESI†), characteristic of the SMM behaviour. The Cole–Cole plots can be fit using a generalized Debye model to extract the relaxation time (τ).64 The α values, which are the coefficients that determine the width of the distribution of relaxation times, fall in the range of 0.27–0.55 in the temperature range of 2–12 K (Table S7, ESI†). The broad range of α values suggests that more than one relaxation process could operate at low temperatures. The best fit of the ln
τ vs. T−1 curve can be achieved by considering the Orbach and Quantum tunnelling processes using eqn (1),65 where Ueff represents the energy barrier (Fig. 6d). The parameters are Ueff = 41.5 K (28.8 cm−1), τ0 = 2.2 × 10−5 s and τQTM = 0.06 s.
| 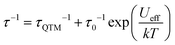 | (1) |
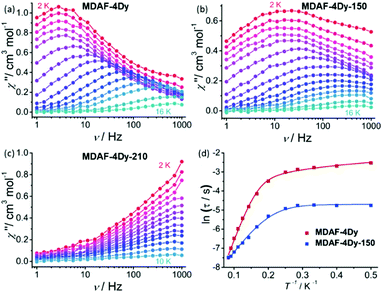 |
| Fig. 6 Frequency dependence of the χ′′ signals measured under zero dc field for (a) MDAF-4Dy, (b) MDAF-4Dy-150 and (c) MDAF-4Dy-210 at the depicted temperatures. (d) Plots of ln τ vs. T−1 for MDAF-4Dy and MDAF-4Dy-150. | |
To better understand the magnetic properties of MDAF-4Dy, complete-active-space self-consistent field (CASSCF) calculations on an individual DyIII fragment of complex MDAF-4Dy on the basis of X-ray determined geometries have been carried out with MOLCAS 8.466 and SINGLE_ANISO67 programs (see the ESI† for details). The calculated energy levels (cm−1), g (gx, gy, gz) tensors, and the predominant mJ values of the lowest eight KDs of one type of individual DyIII fragment are shown in Table S10 (ESI†). The ground KD is mostly composed of the mJ = ±15/2 state (Table S11, ESI†). The first excited KD is mixed by several mJ states severely, resulting in a large transversal magnetic moment (Fig. 7). The transversal magnetic moment in the ground KD of MDAF-4Dy is only 0.10 × 10−1μB which is small enough for the relaxation to proceed through the first excited state. However, the experimental effective Ueff is much smaller than the calculated energy gap between the lowest two KDs of an individual DyIII fragment, attributed to the possible unfavourable effects of anharmonic phonons,68 Raman magnetic relaxation, quantum tunneling of the magnetization (QTM), and so on, on the energy barrier. Although the magnetic anisotropy of compound MDAF-4Dy mainly comes from individual DyIII, the DyIII–DyIII interactions have a considerable influence on its slow magnetic relaxation process.
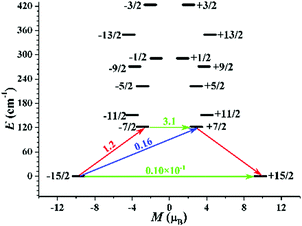 |
| Fig. 7 Magnetization blocking barrier for individual DyIII fragments in MDAF-4Dy. The thick black lines represent the KDs of the individual DyIII fragment as a function of their magnetic moment along the magnetic axis. The green lines correspond to the diagonal matrix elements of the transversal magnetic moment. The blue line represents the Orbach relaxation process. The path shown by the red arrows represents the most probable path for magnetic relaxation in the corresponding compounds. The numbers at each arrow stand for the mean absolute value of the corresponding matrix element of transition magnetic moment. | |
The magnetic susceptibilities of MDAF-4Dy were fit by the program POLY_ANISO67 using the exchange parameters from Table 3. As the calculated ground gz value of individual DyIII fragment is close to 20, the DyIII–DyIII exchange interactions can be approximately regarded as the Ising type during the fitting (Fig. 8). All parameters were calculated with respect to the pseudo-spin
= 1/2 of the DyIII ions. The total coupling parameters
total (dipolar and exchange) were included in fitting the magnetic susceptibilities. The calculated and experimental χMT versus T plots of MDAF-4Dy are shown in Fig. S31 (ESI†), where the fit is close to the experiment at low temperatures, but it has some deviation at high temperatures.69 The negative values of the total coupling parameter
total (Table 3) are indicative of the AF DyIII–DyIII interactions in MDAF-4Dy within the Lines model.70 The exchange energies E (cm−1), the energy differences between each exchange doublets Δt (cm−1) and the gz for the lowest eight exchange doublets are all shown in Table S12 (ESI†), where the gz value of the ground exchange state is 0.000, which confirms the AF interactions between the DyIII ions.
Table 3 Fitted exchange coupling constant
exch, the calculated dipole–dipole interaction
dip and the total
total between the DyIII ions in MDAF-4Dy. The intermolecular interaction zJ′ was fitted to 0.01 cm−1
MDAF-4Dy
|
dip
|
exch
|
total
|
1
|
−0.38 |
−0.75 |
−1.13 |
2
|
−2.78 |
−2.00 |
−4.78 |
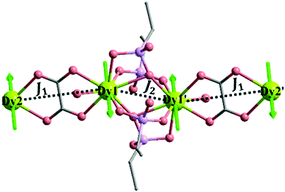 |
| Fig. 8 Scheme of the DyIII–DyIII interactions in MDAF-4Dy. The green arrows represent the calculated orientations of the local main magnetic axes on DyIII ions in the ground KDs. | |
Thermal treatment of MDAF-4Dy led to dramatic changes in magnetic dynamics. For MDAF-4Dy-150, frequency-dependent ac signals were again observed under zero dc field (Fig. 6b and Fig. S25, ESI†), characteristic of the SMM behaviour. However, the χ′′ signals become broader with the peaks moving to higher frequencies. The broad ac signals suggest the presence of multiple relaxation processes. By considering both the Orbach and the Quantum tunnelling processes, the best fit of the ln
τ vs. T−1 curve was achieved using eqn (1), giving parameters of Ueff = 24.0 K (16.7 cm−1), τ0 = 7.3 × 10−5 s and τQTM = 0.009 s. The ac susceptibilities of the rehydrated sample of MDAF-4Dy-150-re were tested, which gave identical profiles to those of MDAF-4Dy (Fig. S26, ESI†), and similar parameters of Ueff = 39.7 K, τ0 = 2.1 × 10−5 s and τQTM = 0.05 s. The results confirm that the dehydration/rehydration processes of MDAF-4Dy are reversible.
For MDAF-4Dy-210, frequency-dependent χ′′ signals were also observed under zero external field, but without maximum appearing below 1 kHz. The situation was not improved by applying an external field of 500 Oe (Fig. 6c and Fig. S27, ESI†). Therefore, the energy barrier of this phase cannot be estimated.
From the above results, it is clear that all three Dy phases exhibit SMM behaviour under zero dc field. However, the energy barriers decrease in the following order: MDAF-4Dy (41.5 K) > MDAF-4Dy-150 (24.0 K) > MDAF-4Dy-210 (not available). The energy barrier of MDAF-4Dy-150 is about half of that of the pristine compound, indicating that the relaxation is accelerated after dehydration. This can be a result of the symmetry reduction of the Dy coordination sphere due to the removal of coordinated water which decreases the magnetic anisotropy. Moreover, the relaxation rate of MDAF-4Dy-210 is even faster because only tails of χ′′ signals were observed at 2 K below 1 kHz. The dissociation of the dianthracene groups in the MOF skeleton may play nontrivial roles in affecting the local geometry of the Dy ions and hence the magnetic dynamics.
For Er compound, only the magnetic properties of pristine MDAF-4Er were investigated. Unlike MDAF-4Dy, MDAF-4Er showed frequency-dependent ac signals only after a dc field was applied. Slow relaxation of magnetization was clearly observed under an optimum field of 1 kOe below 4 K (Fig. S29, ESI†). By assuming that there is only one characteristic relaxation process with one energy barrier and one time constant, the energy barrier can be extracted from eqn (2).71
| 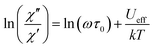 | (2) |
A linear fit of the ln(χ′′/χ′) versus T−1 curve allows the extraction of the energy barrier (Ueff) and pre-exponential factor (τ0) which are 7.4 K and 3.2 × 10−6 s, respectively (Fig. S29e, ESI†). It is worth mentioning that field-induced slow magnetization relaxation was also observed in a few Er-MOFs with energy barriers below 13.0 K.22,72,73
Conclusions
In this study, we report three isostructural Ln-dianthracene compounds Ln2(amp2H2)2(C2O4)(H2O)2·xH2O (MDAF-4Ln) with three-dimensional open framework structures. The structure is composed of Ln-chains and dianthracence linkages. Although dominant AF interactions were observed between the magnetic centres, MDAF-4Dy showed SMM behaviour under zero dc field, which was further rationalized by theoretical calculations. More interestingly, MDAF-4Dy experienced two consecutive phase transitions: first, the loss of all lattice and coordinated water molecules and then, the dissociation of dianthracene groups of amp2H22−. The thermo-induced structural transformations were accompanied by significant changes in its single-molecule magnetic behaviour and luminescence properties. MDAF-4Dy is the first example of the Ln-dianthracene MOFs showing responsive magnetic and luminescence behaviour. This work provides a new route for designing smart magneto-optical bifunctional MOFs for future applications.
Conflicts of interest
There are no conflicts to declare.
Acknowledgements
This work was supported by grants from the National Key R&D Program of China (2017YFA0303203 and 2018YFA0306004) and the National Natural Science Foundation of China (21731003, 21973046).
Notes and references
-
(a) G. M. Espallargas and E. Coronado, Chem. Soc. Rev., 2018, 47, 533–557 Search PubMed;
(b) E. Coronado, Nat. Rev. Mater., 2020, 5, 87–104 Search PubMed.
-
(a) J. D. Rinehart and J. R. Long, Chem. Sci., 2011, 2, 2078–2085 Search PubMed;
(b) Y.-S. Meng, S.-D. Jiang, B.-W. Wang and S. Gao, Acc. Chem. Res., 2016, 49, 2381–2389 Search PubMed;
(c) C. Gao, A. Genoni, S. Gao, S. Jiang, A. Soncini and J. Overgaard, Nat. Chem., 2020, 12, 213–219 Search PubMed.
-
(a) J. C. G. Bünzli and C. Piguet, Chem. Soc. Rev., 2005, 34, 1048–1077 Search PubMed;
(b) X. Yang, X. Lin, Y. Zhao, Y.-S. Zhao and D. Yan, Angew. Chem., Int. Ed., 2017, 56, 7853–7857 Search PubMed.
-
(a) D. N. Woodruff, R. E. P. Winpenny and R. A. Layfield, Chem. Rev., 2013, 113, 5110–5148 Search PubMed;
(b) Z.-H. Zhu, M. Guo, X.-L. Li and J.-K. Tang, Coord. Chem. Rev., 2019, 378, 350–364 Search PubMed;
(c) H. Tian and L.-M. Zheng, Acta Chim. Sin., 2020, 78, 34–55 Search PubMed.
-
(a) R. J. Blagg, C. A. Muryn, E. J. L. McInnes, F. Tuna and R. E. P. Winpenny, Angew. Chem., Int. Ed., 2011, 50, 6530–6533 Search PubMed;
(b) J. D. Rinehart, M. Fang, W. J. Evans and J. R. Long, J. Am. Chem. Soc., 2011, 133, 14236–14239 Search PubMed.
- N. Ishikawa, M. Sugita, T. Ishikawa, S.-Y. Koshihara and Y. Kaizu, J. Am. Chem. Soc., 2003, 125, 8694–8695 Search PubMed.
- M. A. AlDamen, J. M. Clemente-Juan, E. Coronado, C. Martí-Gastaldo and A. Gaita-Ariño, J. Am. Chem. Soc., 2008, 130, 8874–8875 Search PubMed.
- S.-D. Jiang, B.-W. Wang, H.-L. Sun, Z.-M. Wang and S. Gao, J. Am. Chem. Soc., 2011, 133, 4730–4733 Search PubMed.
- Y.-C. Chen, J.-L. Liu, L. Ungur, J. Liu, Q.-W. Li, L.-F. Wang, Z.-P. Ni, L. F. Chibotaru, X.-M. Chen and M.-L. Tong, J. Am. Chem. Soc., 2016, 138, 2829–2837 Search PubMed.
- P.-B. Jin, Y.-Q. Zhai, K.-X. Yu, R. E. P. Winpenny and Y.-Z. Zheng, Angew. Chem., Int. Ed., 2020, 59, 9350–9354 Search PubMed.
- C. A. P. Goodwin, F. Ortu, D. Reta, N. F. Chilton and D. P. Mills, Nature, 2017, 548, 439 Search PubMed.
- F.-S. Guo, B. M. Day, Y.-C. Chen, M.-L. Tong, A. Mansikkamäki and R. A. Layfield, Science, 2018, 362, 1400–1403 Search PubMed.
- C. A. Gould, K. R. McClain, J. M. Yu, T. J. Groshens, F. Furche, B. G. Harvey and J. R. Long, J. Am. Chem. Soc., 2019, 141, 12967–12973 Search PubMed.
- K. Liu, X. Zhang, X. Meng, W. Shi, P. Cheng and A. K. Powell, Chem. Soc. Rev., 2016, 45, 2423–2439 Search PubMed.
- D. Zeng, M. Ren, S.-S. Bao, J.-S. Feng, L. Li and L.-M. Zheng, Chem. Commun., 2015, 51, 2649–2652 Search PubMed.
- Z. Chen, B. Zhao, P. Cheng, X.-Q. Zhao, W. Shi and Y. Song, Inorg. Chem., 2009, 48, 3493–3495 Search PubMed.
- Y.-X. Ren, X.-J. Zheng, L.-C. Li, D.-Q. Yuan, M. An and L.-P. Jin, Inorg. Chem., 2014, 53, 12234–12236 Search PubMed.
- M. Chen, E. C. Sañudo, E. Jiménez, S.-M. Fang, C.-S. Liu and M. Du, Inorg. Chem., 2014, 53, 6708–6714 Search PubMed.
- L. Zhang, S. Guan, Y. Fan, C. Du, D. Zhao and B. Liu, Z. Kristallogr. – Cryst. Mater., 2019, 234, 33–41 Search PubMed.
- C. Bai, C.-T. Li, H.-M. Hu, B. Liu, J.-D. Li and G. Xue, Dalton Trans., 2019, 48, 814–817 Search PubMed.
- C. Zhang, X. Ma, P. Cen, X. Jin, J. Yang, Y.-Q. Zhang, J. Ferrando-Soria, E. Pardo and X. Liu, Dalton Trans., 2020, 49, 14123–14132 Search PubMed.
- J. M. Seco, I. Oyarzabal, S. Pérez-Yáñez, J. Cepeda and A. Rodríguez-Diéguez, Inorg. Chem., 2016, 55, 11230–11248 Search PubMed.
- S. Mohapatra, B. Rajeswaran, A. Chakraborty, A. Sundaresan and T. K. Maji, Chem. Mater., 2013, 25, 1673–1679 Search PubMed.
- X. Zhang, V. Vieru, X. Feng, J.-L. Liu, Z. Zhang, B. Na, W. Shi, B.-W. Wang, A. K. Powell, L. F. Chibotaru, S. Gao, P. Cheng and J. R. Long, Angew. Chem., Int. Ed., 2015, 54, 9861–9865 Search PubMed.
- F. Ma, J. Xiong, Y.-S. Meng, J. Yang, H.-L. Sun and S. Gao, Inorg. Chem. Front., 2018, 5, 2875–2884 Search PubMed.
- Y. Xin, J. Wang, M. Zychowicz, J. J. Zakrzewski, K. Nakabayashi, B. Sieklucka, S. Chorazy and S. I. Ohkoshi, J. Am. Chem. Soc., 2019, 141, 18211–18220 Search PubMed.
- F. Ma, R. Sun, A.-H. Sun, J. Xiong, H.-L. Sun and S. Gao, Inorg. Chem. Front., 2020, 7, 930–938 Search PubMed.
- G. Huang, G. Fernandez-Garcia, I. Badiane, M. Camarra, S. Freslon, O. Guillou, C. Daiguebonne, F. Totti, O. Cador, T. Guizouarn, B. Le Guennic and K. Bernot, Chem. – Eur. J., 2018, 24, 6983–6991 Search PubMed.
-
(a) O. Cador, B. Le Guennic and F. Pointillart, Inorg. Chem. Front., 2019, 6, 3398–3417 Search PubMed;
(b) Z. Zhu, X.-L. Li, S. Liu and J. Tang, Inorg. Chem. Front., 2020, 7, 3315–3326 Search PubMed.
- G. Cosquer, M. Morimoto, M. Irie, A. Fetoh, B. K. Breedlove and M. Yamashita, Dalton Trans., 2015, 44, 5996–6002 Search PubMed.
- P.-F. Shi, B. Zhao, G. Xiong, Y.-L. Hou and P. Cheng, Chem. Commun., 2012, 48, 8231–8233 Search PubMed.
- I. Oyarzabal, B. Fernández, J. Cepeda, S. Gómez-Ruiz, A. J. Calahorro, J. M. Seco and A. Rodríguez-Diéguez, CrystEngComm, 2016, 18, 3055–3063 Search PubMed.
- A. A. García-Valdivia, A. Zabala-Lekuona, A. Goñi-Cárdenas, B. Fernández, J. A. García, J. F. Quílez del Moral, J. Cepeda and A. Rodríguez-Diéguez, Inorg. Chim. Acta, 2020, 509, 119687 Search PubMed.
-
(a) X.-D. Huang, Y. Xu, K. Fan, S.-S. Bao, M. Kurmoo and L.-M. Zheng, Angew. Chem., Int. Ed., 2018, 57, 8577–8581 Search PubMed;
(b) X.-D. Huang, J.-G. Jia, M. Kurmoo, S.-S. Bao and L.-M. Zheng, Dalton Trans., 2019, 48, 13769–13779 Search PubMed;
(c) X.-D. Huang, G.-H. Wen, S.-S. Bao, J.-G. Jia and L.-M. Zheng, Chem. Sci., 2021, 12, 929–937 Search PubMed.
- G. Collet, T. Lathion, C. Besnard, C. Piguet and S. Petoud, J. Am. Chem. Soc., 2018, 140, 10820–10828 Search PubMed.
- Q. Zou, S.-S. Bao, X.-D. Huang, G.-H. Wen, J.-G. Jia, L.-Q. Wu and L.-M. Zheng, Chem. – Asian J., 2021 DOI:10.1002/asia.202100283.
-
(a) J.-C. Liu, X.-D. Huang, Q. Zou, S.-S. Bao, X.-Z. Wang, J.-Y. Ma and L.-M. Zheng, J. Mater. Chem. C, 2020, 8, 7369–7377 Search PubMed;
(b) Q. Zou, J.-C. Liu, X.-D. Huang, S.-S. Bao and L.-M. Zheng, Chin. Chem. Lett., 2021, 32, 1519–1522 Search PubMed;
(c) J.-C. Liu, Q. Zou, X.-D. Huang, S.-S. Bao and L.-M. Zheng, Eur. J. Inorg. Chem., 2021, 1565–1570 Search PubMed.
-
A. Clearfield and K. Demadis, Metal Phosphonate Chemistry: From Synthesis to Applications, The Royal Society of Chemistry, 2012 Search PubMed.
- J.-G. Mao, Coord. Chem. Rev., 2007, 251, 1493–1520 Search PubMed.
-
(a) S.-S. Bao, G. K. H. Shimizu and L.-M. Zheng, Coord. Chem. Rev., 2019, 378, 577–594 Search PubMed;
(b) G.-G. Weng and L.-M. Zheng, Sci. China: Chem., 2020, 63, 619–636 Search PubMed;
(c) S.-S. Bao, M.-F. Qin and L.-M. Zheng, Chem. Commun., 2020, 56, 12090–12108 Search PubMed.
-
A. D. G. Firmino, F. Figueira, J. P. C. Tomé, F. A. A. Paz and J. Rocha, Coord. Chem. Rev., 2018, 355, 133–149 Search PubMed.
- F.-N. Shi, F. A. Almeida Paz, P. Ribeiro-Claro and J. Rocha, Chem. Commun., 2013, 49, 11668–11670 Search PubMed.
-
(a) J. A. Groves, P. A. Wright and P. Lightfoot, Inorg. Chem., 2005, 44, 1736 Search PubMed;
(b) J. P. S. Mowat, J. A. Groves, M. T. Wharmby, S. R. Miller, Y. Li, P. Lightfoot and P. A. Wright, J. Solid State Chem., 2009, 182, 2769–2778 Search PubMed.
- C. Serre, N. Stock, T. Bein and G. Férey, Inorg. Chem., 2004, 43, 3159 Search PubMed.
- D.-K. Cao, S.-Z. Hou, Y.-Z. Li and L.-M. Zheng, Cryst. Growth Des., 2009, 9, 4445–4449 Search PubMed.
- R. Fu, S. Hu and X. Wu, Cryst. Growth Des., 2014, 14, 6197–6204 Search PubMed.
-
O. Kahn, Molecular Magnetism, Wiley-VCH, New York., 1993 Search PubMed.
- SAINT, Program for Data Extraction and Reduction, Siemens Analytical X-ray Instruments, Madison, WI, 1994–1996.
- SHELXTL (version 5.0), Reference Manual, Siemens Industrial Automation, Analytical Instruments, Madison, WI, 1995.
- A. L. Spek, J. Appl. Crystallogr., 2003, 36, 7–13 Search PubMed.
- M. Pinsky and D. Avnir, Inorg. Chem., 1998, 37, 5575–5582 Search PubMed.
- X. Li, T. Liu, Q. Lin and R. Cao, Cryst. Growth Des., 2010, 10, 608–617 Search PubMed.
- L. Zhang, S. Xu, Y. Zhou, X. Zheng, C. Yu, Z. Shi, S. U. Hassan and C. Chen, CrystEngComm, 2011, 13, 6511–6519 Search PubMed.
- Y. Zhao, C.-Q. Jiao, Z.-G. Sun, Y.-Y. Zhu, K. Chen, C.-L. Wang, C. Li, M.-J. Zheng, H. Tian, S.-H. Sun and W. Chu, Cryst. Growth Des., 2012, 12, 3191–3199 Search PubMed.
- T.-H. Yang, D.-K. Cao, Y.-Z. Li and L.-M. Zheng, J. Solid State Chem., 2010, 183, 1159–1164 Search PubMed.
- L. Liu, Z.-G. Sun, N. Zhang, Y.-Y. Zhu, Y. Zhao, X. Lu, F. Tong, W.-N. Wang and C.-Y. Huang, Cryst. Growth Des., 2010, 10, 406–413 Search PubMed.
- Y. Zhu, Z. Sun, Y. Zhao, J. Zhang, X. Lu, N. Zhang, L. Liu and F. Tong, New J. Chem., 2009, 33, 119–124 Search PubMed.
- D. W. Kang, S. E. Ju, D. W. Kim, M. Kang, H. Kim and C. S. Hong, Adv. Sci., 2020, 7, 2002142 Search PubMed.
- D. J. De Frees, M. D. Miller, D. Talbi, F. Pauzat and Y. Ellinger, Astrophys. J., 1993, 408, 530–538 Search PubMed.
- K. B. Yatsimirskii and N. K. Davidenko, Coord. Chem. Rev., 1979, 27, 223–273 Search PubMed.
- X.-D. Huang, M. Kurmoo, S.-S. Bao, K. Fan, Y. Xu, Z.-B. Hu and L.-M. Zheng, Chem. Commun., 2018, 54, 3278–3281 Search PubMed.
- Y. Xu, S.-S. Bao, X.-D. Huang and L.-M. Zheng, Cryst. Growth Des., 2018, 18, 4045–4053 Search PubMed.
- K. L. M. Harriman, J. L. Brosmer, L. Ungur, P. L. Diaconescu and M. Murugesu, J. Am. Chem. Soc., 2017, 139, 1420–1423 Search PubMed.
- K. S. Cole and R. H. Cole, J. Chem. Phys., 1941, 9, 341–351 Search PubMed.
- G. Brunet, R. Marin, M.-J. Monk, U. Resch-Genger, D. A. Gálico, F. A. Sigoli, E. A. Suturina, E. Hemmer and M. Murugesu, Chem. Sci., 2019, 10, 6799–6808 Search PubMed.
- F. Aquilante, J. Autschbach, R. K. Carlson, L. F. Chibotaru, M. G. Delcey, L. D. Vico, I. F. Galván, N. Ferré, L. M. Frutos, L. Gagliardi, M. Garavelli, A. Giussani, C. E. Hoyer, G. Li Manni, H. Lischka, D. Ma, P. Å. Malmqvist, T. Müller, A. Nenov, M. Olivucci, T. B. Pedersen, D. Peng, F. Plasser, B. Pritchard, M. Reiher, I. Rivalta, I. Schapiro, J. Segarra-Martí, M. Stenrup, D. G. Truhlar, L. Ungur, A. Valentini, S. Vancoillie, V. Veryazov, V. P. Vysotskiy, O. Weingart, F. Zapata and R. Lindh, J. Comput. Chem., 2016, 37, 506–541 Search PubMed.
-
(a) L. F. Chibotaru, L. Ungur and A. Soncini, Angew. Chem., Int. Ed., 2008, 47, 4126–4129 Search PubMed;
(b) L. Ungur, W. Van den Heuvel and L. F. Chibotaru, New J. Chem., 2009, 33, 1224–1230 Search PubMed;
(c) L. F. Chibotaru, L. Ungur, C. Aronica, H. Elmoll, G. Pilet and D. Luneau, J. Am. Chem. Soc., 2008, 130, 12445–12455 Search PubMed.
- A. Lunghi, F. Totti, R. Sessoli and S. Sanvito, Nat. Commun., 2017, 8, 14620 Search PubMed.
- S. K. Langley, D. P. Wielechowski, V. Vieru, N. F. Chilton, B. Moubaraki, B. F. Abrahams, L. F. Chibotaru and K. S. Murray, Angew. Chem., Int. Ed., 2013, 52, 12014–12019 Search PubMed.
- M. E. Lines, J. Chem. Phys., 1971, 55, 2977–2984 Search PubMed.
- P. Mondal, B. Dey, S. Roy, S. P. Bera, R. Nasani, A. Santra and S. Konar, Cryst. Growth Des., 2018, 18, 6211–6220 Search PubMed.
- A. J. Calahorro, I. Oyarzabal, B. Fernández, J. M. Seco, T. Tian, D. Fairen-Jimenez, E. Colacio and A. Rodríguez-Diéguez, Dalton Trans., 2016, 45, 591–598 Search PubMed.
- L. H. G. Kalinke, D. Cangussu, M. Mon, R. Bruno, E. Tiburcio, F. Lloret, D. Armentano, E. Pardo and J. Ferrando-Soria, Inorg. Chem., 2019, 58, 14498–14506 Search PubMed.
Footnote |
† Electronic supplementary information (ESI) available. CCDC 2065604–2065606. For ESI and crystallographic data in CIF or other electronic format see DOI: 10.1039/d1tc01027a |
|
This journal is © The Royal Society of Chemistry 2021 |