DOI:
10.1039/D1TC01026C
(Paper)
J. Mater. Chem. C, 2021,
9, 10697-10704
Terbium(III) bis-phthalocyaninato single-molecule magnet encapsulated in a single-walled carbon nanotube†
Received
5th March 2021
, Accepted 26th March 2021
First published on 27th March 2021
Abstract
In this study, terbium(III) bis-phthalocyaninato single-molecule magnets (TbPc2 SMMs) were encapsulated in the internal nano space of single-walled carbon nanotubes (SWCNTs) for the first time. The magnetic and electronic properties of the TbPc2 SMM–SWCNT hybrids were investigated in detail by using dc and ac magnetic susceptibility measurements, TEM, SEM, STM, STS, etc. By arranging the TbPc2 SMMs in the one-dimensional internal nano spaces of SWCNTs, it is possible to investigate the essential SMM characteristics of TbPc2 without considering ligand field (LF) effects. In addition, it appears that the electron correlation between TbPc2 and the SWCNT can affect the electrotransport and/or electromagnetic properties. Furthermore, since the stable internal nano space of SWCNTs is used, it is thought that the density of SMMs in the SMM–SWCNT hybrid material can be controlled, and the hybrids should be usable as spin valves. Our strategy may pave the way for the construction of SMM–SWCNT hybrid materials.
Introduction
It is no exaggeration to say that modern society is supported by highly functional materials, especially those containing magnets based on nanoscale units. Improving molecular electronics, spintronics, data storage and quantum information processing is important for the further development of an information-oriented society in the future.1–7 In recent years, single-molecule magnets (SMMs) have been prepared as metal complexes by using bottom-up methods.1,8–14 These SMMs exhibit slow magnetic relaxation behaviour with magnetic hysteresis at extremely low temperatures, magnetic bistabilities, quantum tunnelling of the magnetization, and quantum coherence. In 1993, Sessoli and Gatteschi et al. reported the first example of an SMM, [Mn12O12(OAc)16(H2O)4]·2AcOH (abbreviated as Mn12).8,15 Classical magnets exhibit large magnetization via three-dimensional interactions of the electron spins of the metal ions, but for SMMs, uniaxial magnetic anisotropy of one molecule occurs. For SMMs, the degeneracy of the total spin state S is split into (2S + 1) by the ligand field (LF), and double-well potentials occur for the spin sublevel, causing a barrier to spin relaxation. At this time, the split width of the energy levels is defined as Ueff = |D|S2 (D = zero-field splitting constant) (e.g., for Mn12, D = −0.46 cm−1, S = 10, Ueff = 40 cm−1 and coercivity (HC) = 2.2 T@1.5 K) due to the magnetic anisotropy. At low temperatures, SMMs behave like magnets which undergo slow magnetic relaxation. On the other hand, the lanthanoid-type SMMs are different from transition metal SMMs since their SMM behaviours come from the f electrons. Since the orbital angular momentum L does not disappear for f electrons, ground multiplets having a total angular momentum, J, form due to spin–orbit interactions. When the ground multiplet is placed in an LF environment, the degeneration splits into (2J + 1) sublevels. As a result, double-well potentials form, and SMM behaviour is exhibited.8,16 In general, the sublevel splitting in lanthanoid-type SMMs (102–103 cm−1)17 is much larger than that of transition metal-type SMMs (ca. 70 cm−1),18 meaning that Ln type SMMs are more useful in terms of SMM characteristics, such as Ueff.19 Here we focused on a terbium(III) phthalocyaninato double-decker SMM. In 2003, anionic [TbPc2]−·TBA+ (Tb3+ = terbium(III) ion, Pc2− = phthalocyaninato, TBA+ = (C4H9)4N+) was reported as the first single ion magnet (SIM) by Ishikawa et al. (Ueff = 230 cm−1, HC ≈ 0.01 T@0.04 K),16,20 and in the following year, the same group reported neutral TbPc2 in which the π-radical were delocalized on the Pc2− ligands (Pc˙−).21 The peak-top temperature of χ′′ for TbPc2 at an alternating current (ac) frequency of 1500 Hz (ν) has been reported to be about 50 K (Ueff = 410 cm−1), which is high compared to Mn12 SMMs. However, butterfly-type magnetic hysteresis is observed below 1.8 K. This magnetic behaviour is due to the magnetic relaxation via quantum tunnelling of the magnetization (QTM). If QTM is suppressed, magnetic hysteresis with HC can be observed at temperatures much higher than those of conventional SMMs.22 From the viewpoint of suppressing QTM, we were able to suppress QTM and improve the HC of TbPc2 derivatives by utilizing the magnetic dipole interactions between SMMs. A one-dimensional structure was confirmed for TbNcPc in which naphthalocyaninato (Nc2−) and Pc2− are coordinated to the Tb3+ ion, and magnetic hysteresis with HC was observed at a relatively high temperature of 25 K (Ueff = 584 cm−1, HC ≈ 150 mT@1.8 K).23 In other words, the magnetic hysteresis properties were improved by suppressing QTM. In addition, we have compared the SMM properties of crystal samples of TbPc2 (P212121, Pnma) and magnetically diluted TbPc2 samples.24 Diluting the γ-phase (P212121) causes the hysteresis loop in the magnetization (M) versus magnetic field (H) to open. In other words, the SMM characteristics are improved. On the other hand, for the Pnma crystal, the M–H hysteresis loop is open. Thus, for TbPc2, the combination of the orientation of the magnetic anisotropy and the arrangement of the magnetic dipole interactions has a dramatic effect on the performance of SMMs. In order to design SMMs for practical use, Ueff must be increased, and fast QTM processes must be suppressed.
In addition, the internal nano space of carbon nanotubes (CNTs) can be regarded as reaction vessels, which are 1/1000 smaller than that of a microreactor.25 Therefore, a chemical reaction field on a diameter range of 1–3 nm (100 nm to 0.1 mm in length) is expected to have a great influence on reaction chemistry and/or nanoelectronics research in the 21st century.26–29 It has been shown that atoms and molecules can be contained in the nano space of CNTs.30–35 Focusing on the use of the stable one-dimensional nano space of a CNT as a host for host–guest structures, various SMM–CNT hybrid materials have been reported for nanoelectronics and spintronics research.36 In 2011, Del Carmen Giménez-López et al. reported Mn12@MWCNT, where Mn12 with a diameter of 1.6 nm and a height of 1.1 nm was incorporated in a multi-walled CNT (MWCNT) (internal diameter range 5–50 nm), and its magnetic properties.37 It shows that the SMM characteristics decrease after encapsulation in MWCNTs. In 2017, we encapsulated Dy(acac)3(H2O)2 SMMs in MWCNTs by using a capillary method (abbreviated Dy(acac)3(H2O)2@MWCNT).38 Dy(acac)3(H2O)2@MWCNT shows clear ac frequency dependence, but it does not have enhanced SMM properties. In 2009, Kyatskaya et al. reported a TbPc2–SWCNT hybrid material, where a TbPc2 derivative was attached to the outside of a SWCNT, and its magnetic properties.39 In TbPc2–SWCNT hybrid spin valves, the maximum magnetic resistance ratio between the parallel state and the antiparallel state is about 300% at submillikelvin temperatures.40 It suggests that a spin valve with a larger magnetoresistance ratio can be prepared by using SMMs instead of ferromagnets as the spin source. In 2018, we encapsulated DySc2N@C80 SMMs in SWCNT nano-peapods (abbreviated DySc2N@C80@SWCNT) and reported on the magnetic properties.41 From a magnetization (M) vs. magnetic field (H) plot at 1.8 K, a significant improvement in HC was observed after encapsulation in a SWCNT. The improvement in the magnetic properties is thought to be due to the following two factors. First, the one-dimensional chain structure of DySc2N@C80 in a SWCNT works on the magnetic dipole interactions between adjacent DySc2N@C80 molecules, and the dipolar bias suppresses the magnetic relaxation of QTM. Second, charge transfer (CT) between DySc2N@C80 and SWCNT causes perturbations in the crystal field (CF).
Inspired by the previous research, we investigated whether or not QTM could be suppressed using the dipolar bias associated with the one-dimensional TbPc2 SMM chain structure in the internal space of SWCNTs. In addition, since the stable nano space of SWCNTs allows the control of the arrangement of SMMs, detailed investigation of the role of the SMM sequence in spin valve characteristics may be possible. To the best of our knowledge, no examples of TbPc2 SMM encapsulated in a SWCNT have been reported to date. Finally, we investigated the effects of CT and LF (or CF) distortions on the magnetic properties of TbPc2 SMMs encapsulated in the nano space of SWCNTs. If we can take advantage of the TbPc2 SMM characteristics in a SWCNT, highly functional materials can be prepared (Fig. 1).
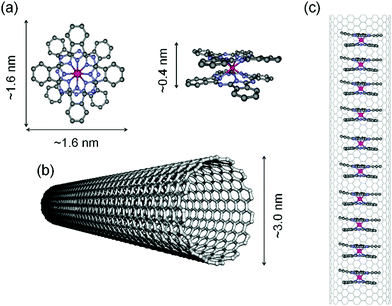 |
| Fig. 1 Chemical structure of TbPc2 SMM and schematic illustration of its encapsulation in SWCNTs. (a) Ball and stick structure of TbPc2. Hydrogen atoms omitted for clarity: top (left) and side (right) view. Colouring scheme: Tb3+, pink; N, cyan; C, grey. (b) Ball and stick structure of SWCNTs. (c) Schematic illustration of the TbPc2 SMM encapsulated in a SWCNTs (abbreviated as TbPc2@SWCNT). | |
Experimental
General procedure for the synthesis of TbPc2
All reagents were purchased from Wako Pure Chemical Industries, Ltd, TCI, Strem Chemicals, Inc. and Sigma-Aldrich Co. LLC. and used without further purification. TbPc2 was prepared following a reported procedure.42 A mixture of 1,2-dicyanobenzene (62.7 mmol), Tb(OAc)3·4H2O (3.92 mmol) and 1,8-diazabicyclo[5,4,0]undec-7-ene (DBU) (33.5 mmol) in 40 mL of 1-hexanol was refluxed for 42 h. The solution was allowed to cool to room temperature and then filtered. The precipitate was washed with n-hexane. Then the precipitate was dried in air. The crude purple product was extracted with ten 200 mL portions of CHCl3. The green extracts were combined, concentrated, and purified using column chromatography (C-200 silica gel, Wako). The eluent was 98
:
2 CH2Cl2/MeOH. The green fraction, which was the first fraction, was collected while being careful not to contaminate with the anionic [TbPc2]− complex, which was the second fraction (blue-green). The green fraction was concentrated, and n-hexane was added until the compound precipitated. The green precipitate was filtered and dried in vacuo. The solid (8 mg) was dissolved in 20 mL of CHCl3 and filtered. n-Hexane was layered on the top of green filtrate. After two weeks, deep green needle-like crystals were obtained in the γ-phase (Fig. S1, ESI†).42 In general TbPc2 exhibits two broad absorption bands characteristic of a radical Pc˙− ligand in the ranges of 450–500 nm (BV: Blue Vibronic) and 900–1000 nm (RV: Red Vibronic) as well as another broad band in the range of 1200–2000 nm due to intramolecular CT (IV: Intervalence Transition) between the two rings (Fig. S2, ESI†).43 In the spectra, only four main absorption bands in the UV-visible region were observed. The three main absorption bands were assigned to the Q (670 and 600 nm) and Soret bands (320 nm). TbPc2 was then encapsulated in a SWCNT of without further purification. ESI-MS: m/z (%): 1183.22423 (100%) [M+] (calcd 1183.22493).
Procedures for the encapsulation of TbPc2 in SWCNTs
In order to encapsulate TbPc2 with a diameter of 1.6 nm and a height of 0.4 nm in a SWCNT, SWCNTs with an average diameter of 2.4 ± 0.6 nm, which were synthesized by using enhanced direct injection pyrolytic synthesis (eDIPS) method, were used.44,45 The SWCNTs were cleaned as follows: The SWCNTs (29.8 mg) were heated in an electric furnace at 550 °C in air for 30 min to remove the carbon shell covering the metal catalyst. Next, the sample was dispersed in 75 mL of a prepared 2% hexadecyltrimethylammonium bromide aqueous solution for 24 h using an additive to remove the metals and metal oxides contained in the SWCNTs, and the mixture was heated in a 300 mL round-bottom flask at 100 °C for 5 days. Then the SWCNTs were collected by using filtration with a membrane filter made of hydrophilic polytetrafluoroethylene and having a pore size of 1 μm. The SWCNTs were dispersed in 50 mL of methanol, and filtration was repeated 3 times. The SWCNTs were placed in an electric furnace and vacuum heated at 1200 °C for 24 h to remove unreacted metals. The yield was 18.7 mg. In Fig. S3 (ESI†), scanning electron microscope (SEM) images before and after cleaning and a transmission electron microscope (TEM) image of the SWCNTs after cleaning are shown together with elemental analysis obtained by using energy dispersive X-ray (EDX) spectrometry. From the results of elemental analysis, the peak derived from Fe, which was used as a catalyst in the synthesis of the SWCNTs, which was present before cleaning, was absent after cleaning. In addition, no peaks indicating impurities were observed in the EDX spectra, but it was not confirmed using TEM that the internal space of the SWCNTs did not contain impurities. In other words, the catalyst was not completely removed using the cleaning procedures (please see the Magnetic properties section).
TbPc2 was encapsulated into SWCNTs (hereinafter abbreviated as TbPc2@SWCNT) using a capillary method as follows. One end of the SWCNT was opened by heating the SWCNTs (18.7 mg) in air at 550 °C for 30 min in a glass tube and then vacuum-heated with a gas burner to remove impurities from the SWCNTs. Next, the synthesized TbPc2 (5.2 mg, 5.0 mg, and 4.9 mg) was placed in three 30 mL sample tubes, 25 mL of methanol was added to each sample tube, and the sample tubes were shaken using an ultrasonic cleaner. The above SWCNTs (7.2 mg, 5.5 mg, 4.4 mg, respectively) were added to the three tubes and dispersed. After 1 week, the samples were collected by filtration, washed with methanol and dried in a vacuum desiccator. The samples were placed in glass tubes, and then the tubes were heated under vacuum at 200 °C for 2 h. Next, three dispersions of C60 (5 mg) in 25 mL of 1,2-dichloroethane were added to the TbPc2@SWCNT solutions (TbPc2@SWCNT (mg)
:
C60 (mg) = 12.2
:
5.3, 9.6
:
4.8, 9.1
:
5.1), and allowed to disperse for 1 h. The ends of the SWCNTs were closed. The material was collected by using filtration, washed with 25 mL of toluene, 1,2-dichloroethane, and chloroform until the filtrate became colourless to remove C60 and TbPc2 on the surface. The product was dried in a vacuum desiccator. The yield was 27.4 mg.
Physical property measurements
Electrospray ionization mass (ESI) spectroscopy was performed at the Research and Analytical Centre for Giant Molecules, Tohoku University. UV-Vis-NIR spectra for solutions of TbPc2 (1 × 10−5 mol dm−3) in CHCl3 in a quartz cell with a pathlength of 1 cm were acquired on a SHIMADZU UV-3100PC at 298 K. Powder X-ray diffraction patterns were collected on a Bruker D2 PHASER with Cu Kα radiation (λ = 1.5406 Å) at 298 K, a diffraction angle, 2θ, range of 5.0°–50.0°, a sampling width of 0.02°, and an irradiation time of 0.5 seconds. TbPc2@SWCNT and SWCNTs were identified by using scanning electron microscopy (SEM), transmission electron microscopy (TEM), and energy dispersive X-ray (EDX) spectrometry. SEM images were acquired on a S-4300 manufactured by Hitachi High-Technologies Corporation. TEM and STEM images were acquired using a JEM-2100F manufactured by JEOL (acceleration voltage; 200 kV) and Titan cubed G2 60-300 manufactured by FEI (acceleration voltage; 60 kV). For TEM and STEM, the samples were prepared by dispersing the samples in 1,2-dichloroethane then dropping them onto Cu grids, followed by heating in a vacuum. For scanning tunneling microscopy (STM), a solution of 1 mg of dry encapsulated SWCNTs in 5 mL of dichloroethane was mixed using an ultrasonicator (100 W) for 1 h. After sonication, the dispersion was drop-casted onto Au(111) substrates, which were flame-annealed in air prior to the deposition. After deposition, the sample was annealed again in air at 200 °C for 2 h to evaporate the extra carbon and residuals. The samples were placed in an ultra-high vacuum (UHV) environment and STM and scanning tunneling spectroscopy (STS) measurements were conducted using a commercial low-temperature STM (Unisoku, Japan) with a base pressure of 10−8 Pa. All measurements were done in a constant current mode at ∼4.6 K with an electrochemically etched W tip (Unisoku, Japan). A lock-in detection technique was used to acquire dI/dV curves. Gwyddion and Origin Pro were used to process the STM images and STS data.
Magnetic property measurements
Magnetic susceptibility measurements were performed on Quantum Design SQUID magnetometers MPMS-3 (Quantum Design, San Diego, CA, USA). Direct current (dc) measurements were performed in the temperature (T) range of 1.8–300 K and in dc magnetic fields (Hdc) of ±70
000 Oe. Alternating current (ac) measurements were performed in an ac frequency (ν) range of 1–1000 Hz with an ac field amplitude of 3 Oe in the presence of an Hdc of zero. Measurements were performed on randomly oriented powder samples of TbPc2@SWCNT (26.9 mg), which were placed in gelatine capsules and fixed to prevent them from moving during measurements. Molar magnetic susceptibilities were not calculated because the content of TbPc2 in the SWCNTs could not be estimated. In Fig. S4 (ESI†), the magnetic susceptibility of SWCNTs (18.68 mg) were determined from χ vs. T plots. It is thought that the magnetic properties are due to magnetic impurities in SWCNTs. In the procedures for the encapsulation of TbPc2 in the SWCNTs, described in the Experimental section, the Fe is used as a catalyst in the synthesis of the SWCNTs.44,45 The presence of trace amounts of Fe, as iron oxide, was confirmed by using EDX after purification of the SWCNTs (Fig. S3, ESI†). The magnetic impurities could not be completely removed during the purification of the SWCNTs, which combined into fibre bundles. However, the magnetic susceptibilities (χ) of the SWCNTs are sufficiently smaller than those of TbPc2@SWCNT (Fig. S7, ESI†). Moreover, no clear frequency dependence was observed in the ac measurements (Fig. S8-2, ESI†). Therefore, the effects of the magnetic impurities are negligible.
Results and discussion
Fig. 2 shows TEM images of TbPc2@SWCNT and EDX and high-angle annular dark field scanning TEM (HAADF-STEM) images (Fig. S5, ESI†). From the TEM image, the internal space of SWCNT was empty before inclusion but contained something after encapsulation. In addition, from the EDX spectrum, a peak corresponding to the energy of the characteristic X-ray emitted from the Tb atom was confirmed. In addition, HAADF-STEM images showed white spots for Tb, indicating that TbPc2 was encapsulated. In other words, some of the substances contained in the SWCNTs were TbPc2 molecules, and TbPc2@SWCNT was prepared. However, TbPc2 is probably in a magnetic diluted state in the SWCNTs. From the STM image, the vertical placement of the π-conjugated plane between TbPc2 and the SWCNT is thought to be due to the presence of CH–π interactions. An X-ray photoelectron spectroscopy (XPS) spectrum of TbPc2@SWCNT is shown in Fig. S6 (ESI†). Each peak was assigned as shown. The broad peak observed in the range of 1200–1300 cm−1 after inclusion was thought to be due to the Tb ion, but it was not confirmed. This is because the amounts of TbPc2 and, thus, the Tb ions in the SWCNTs are relatively low. Furthermore, a peak due to N of phthalocyaninato ligands was observed in the XPS spectrum of TbPc2@SWCNT. However, the amount of TbPc2 molecules cannot be calculated from the intensity ratio of the N peak due to the influence of hexadecyltrimethylammonium bromide used for cleaning the SWCNTs.
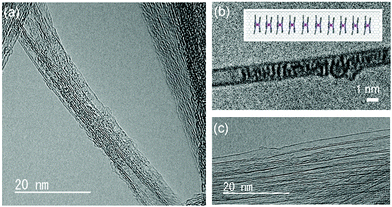 |
| Fig. 2 Structural characterization of TbPc2@SWCNT. Conventional bright field and phase contrast TEM images of TbPc2 encapsulated in SWCNTs. (a) TbPc2 was confirmed to be inside SWCNTs. Tb was detected by using EDX (see Fig. S3, ESI†). (b) TbPc2 were partially stacked one-dimensionally in SWCNTs. (c) Empty SWCNT. | |
Fig. S7a (ESI†) shows the temperature dependence of the dc magnetic susceptibilities (χ) of TbPc2@SWCNT at 1000 Oe. The rise in the χ values of TbPc2@SWCNT at low temperature is thought to be from the spins of TbPc2. In addition, there were no ferromagnetic interactions below 10 K from a χT vs. T plot for TbPc2@SWCNT (Fig. S7b, ESI†). It suggests that TbPc2 is in a diluted state in the SWCNTs. Fig. 3 and Fig. S8 (ESI†) show the frequency (ν) and temperature (T) dependences of the ac magnetic susceptibilities of TbPc2@SWCNT in a zero magnetic field. The ac frequency dependence of TbPc2@SWCNT was confirmed. In a χ′′ vs. T plot, a peak top of T was observed in the range of 30–50 K, which is close to that of pristine TbPc2. In other words, the magnetic relaxation behaviour in TbPc2@SWCNT is not lost. Moreover, the peak temperature position was shifted to the lower temperature side in comparison to that of pristine TbPc2 (Fig. S8, ESI†).21 Since the shift resembles that for diluted samples of pristine TbPc2, it is thought that TbPc2 is in a diluted state in the SWCNTs. Detailed measurements of ac magnetic susceptibilities were performed to compare the SMM properties of TbPc2 before and after encapsulation in SWCNTs. An Arrhenius plot for TbPc2@SWCNT was prepared using the peak top temperature data in Fig. S9 (ESI†) using τ = τ0
exp(Ueff/T) and τ = 1/(2πv) (Table S1, ESI†), and Ueff was estimated to be 430 cm−1 with the frequency factor (τ0) ≈ 2.9 × 10−10 s. This value is similar to that for pristine TbPc2 (Ueff ≈ 410 cm−1 with τ0 ≈ 1.5 × 10−9 s).21 The small difference is due to the dilution conditions. In addition, the Ueff and τ0 values for TbPc2–SWCNT hybrid material are on the same order of magnitude (Ueff ≈ 351 cm−1 with τ0 ≈ 6.2 × 10−8 s).39
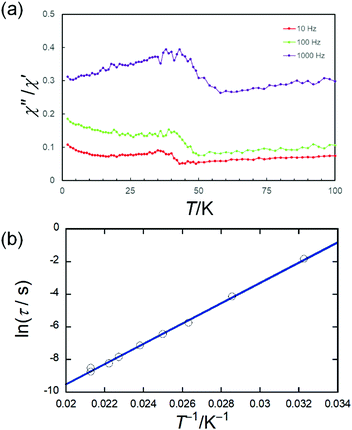 |
| Fig. 3 Frequency (ν) and temperature (T) dependences of the ac magnetic susceptibilities (in-phase (χ′), out-of-phase (χ′′)) of TbPc2@SWCNT. (a) χ′′/χ′ versus T plot at the given ν (10, 100, 1000 Hz) of the ac susceptibility data (Fig. S8, ESI†). We have tried to clarify the existence of peaks by displaying the data as a χ′′/χ′ versus T plot because it is difficult to clearly determine the position of the peaks from χ′′ vs. T plots (Fig. S8-1(b), ESI†). The lines are guides for eyes. In addition, the peak top position in the χ′′/χ′ versus T plot is the same as the peak top position in the χ′′ vs. T plot (from ref. 21). (b) Arrhenius plots for TbPc2@SWCNT, for which the τ values were obtained from Fig. S9 (ESI†). | |
Fig. 4 shows a plot of M vs. H for TbPc2@SWCNT with slight butterfly-type magnetic hysteresis at 1.8 K. This M–H behaviour is similar to the magnetically diluted sample of TbPc2.24 Moreover, the magnetic hysteresis did not improve at high temperature. It is known that the magnetic hysteresis of TbPc2 changes depending on the difference in the dimensionality of the intermolecular interactions.24 Since TbPc2 is in a diluted state in a SWCNT, i.e., the number of TbPc2 molecules in the SWCNTs is small, the interactions between the molecules are suppressed. In the case of Mn12@MWCNT, from the ac magnetic susceptibility measurements, it undergoes two different magnetic relaxation processes.37 The authors conclude that there are two types of Mn12 molecules with axes in different external environments, causing changes in the uniaxial magnetic anisotropy parameter D and the different magnetic relaxation processes. In addition, the Ueff obtained from the peak position of χ′′ has been estimated to be 57 K, which is lower than the activation barrier (Ueff = 72 K) for pristine Mn12.15,37 For Mn12@MWCNT, HC is smaller than that for pristine Mn12. Moreover, the changes in the derivatives of the hysteresis curves near a zero magnetic field increase, meaning that the magnetic relaxation via QTM is promoted. Therefore, they conclude that the SMM characteristics decrease after encapsulated in a MWCNT. In the case of DySc2N@C80@SWCNT, from field-cooled (FC) and zero field-cooled (ZFC) measurements, the blocking temperature (TB = 5 K) does not change before and after encapsulation in a SWCNT.41 On the other hand, from a M–H plot at 1.8 K, a significant improvement in HC has been observed after encapsulation in SWCNTs. This is due to the following two factors. First, the one-dimensional chain structure of DySc2N@C80 in the SWCNT affects the magnetic dipole interactions between adjacent DySc2N@C80 molecules, and the dipolar bias suppresses QTM. Second, charge transfer (CT) between SWCNT and DySc2N@C80 may increase the magnetic moment. In a report in which DySc2N@C80 is included in MOF-177, the effective magnetic moment of DySc2N@C80@MOF-177 has been reported to increase due to the CT from MOF-177 to DySc2N@C80, and they conclude that CT perturbs the crystal field (CF) around the Dy3+ ion, suppresses QTM, and improves HC.46 In case of TbPc2@SWCNT, LF of TbPc2 was not perturbed. In other words, it is possible to investigate the essential SMM characteristics of TbPc2 in the one-dimensional internal nano space of the SWCNTs without considering LF effects.
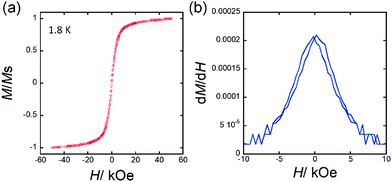 |
| Fig. 4 (a) M–H curve and (b) dM/dH versus H plot for TbPc2@SWCNT at 1.8 K. The vertical axis, M/Ms, shows magnetization values normalized to the saturated magnetization value. The butterfly-type magnetic hysteresis seems to be closed in (a), but it is slightly open which was confirmed by using magnetic field differentiation of M in (b). The lines are guides for eyes. | |
The electronic state of the SWCNTs changes when molecules are encapsulated in them, that is ‘local bandgap engineering’.47 For example, from determination of the electronic state of nano-peapod Gd@C82@SWCNT by using STS at 5 K, it has been reported that the band gap before encapsulation is 0.43 eV but 0.17 eV after encapsulation.47,48 Since the π orbital protruding inside the SWCNT is spatially close to the π orbital of the fullerene and strongly interacts with it, the electronic state of the outer SWCNT and the band gap change.48–50 Therefore, it is possible to modulate the electronic state of SWCNTs with nanometer spatial resolution via encapsulation. In fact, ambipolar field effect transistor (FETs) using the electronic state of Gd@C82@SWCNT have been reported.51 The bandgap is reduced by the inclusion of Gd@C82, and p- and n-type electronic states become accessible by controlling the gate voltage.
In order to characterize the electronic configuration of the SWCNT with a TbPc2 molecule, we performed STM on a Au(111) surface using a cryogenic STM setup. We observed the surface of the SWCNT with atomic scale resolution. Even for identical SWCNTs, one area showed a perfect periodic atom arrangement without disturbances, and the other area showed belt-like protrusions which disturbs the regular periodic structure. A topographical image for an area showing perfect periodicity is shown in Fig. 5(a) together with an STS spectrum obtained at the position of the blue circle in the topographical image shown in Fig. 5(b). The topographical image showed a periodic pattern and lattice size identical to those in our previous report.52 In the STS spectrum, little change was observed even at other positions on the surface in Fig. 5(a). In Fig. 5(b), at both the occupied and unoccupied positions, indicated by arrows, there were staircase-like increases in the conductance, which are characteristic of van Hove singularities (VHS) and originate from the two-dimensional band structure of the CNT.47
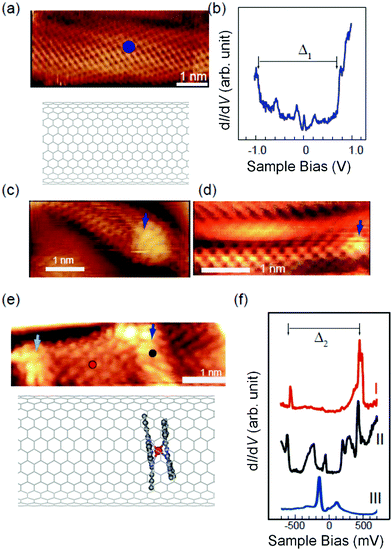 |
| Fig. 5 (a) STM image and (b) STS spectra of empty SWCNT. (c) and (d) STM images of TbPc2@SWCNT. (e) STM image showing empty (red) and filled (black) regions. (f) Comparative STS spectra measured at the red and black points in (e). | |
At different parts of the SWCNTs, we observed protruded areas, which appear like nodules on the tube. The protrusion often had an inner structure which looked like a short belt running perpendicular to the long axis of the SWCNT. Examples of areas with the nodule-like protrusions are shown in Fig. 5(c) and (d). The bright regions are marked by arrows in all figures, which are ∼0.2 Å protruded from the rest of the SWCNT surface. However, there are reports where standing wave features are observed at the end of the SWCNT, which are caused by the forward wave and the backward wave scattered at the cap end.52 Thus, to avoid any confusion, we concentrated on the protrusions in the middle of the tube. The density and the height were considerably different from those of the adsorbates or the defects of the SWCNT. In Fig. 5(c), the protrusion at the arrow had a striped pattern in which the stripes were separated by 2 Å. Note that this feature is not located at the end of the tube, although the right-hand side of the protrusion seems slightly narrow due to the imaging conditions. In Fig. 5(d), a clear atomic structure with a regular periodicity and the disturbance at the protrusion position in the right end were observed. In the magnified image of Fig. 5(e), two areas were protruded sandwiching a pristine region in the middle. There were clear inner structures with spacings similar to those of the SWCNT despite being distorted. We believe that they represent the position of the TbPc2 molecules inside the SWCNT.
The STS spectra measured at the red and black positions of Fig. 5(e) are shown in Fig. 5(f). Plot I have two significant features, which are VHS pairs. The red mark is separated ∼1 nm from the TbPc2 positions. Thus, the effects of the encapsulated TbPc2 are extended at least this far. On the other hand, plot II in Fig. 5(f) contains not only a VHS pair (Δ2) but also several features between them. Those features are caused by the hybridization between the electronic states of the TbPc2 molecule and the SWCNTs. The STS of the TbPc2 molecule adsorbed on Au(111) surface, which is illustrated as plot III in Fig. 5(f) as a reference, has been reported by our group.53 The two features straddling the Fermi level correspond to the highest occupied molecular orbital (HOMO) and lowest unoccupied molecular orbital (LUMO) from the π orbital of the Pc ligand. These two features can split in different chemical environments even for a single molecule, and this has been shown to occur for a TbPc2 molecular film. The model of the adsorption for the TbPc2 molecule inside the SWCNT tube illustrated in Fig. 5(e) shows the asymmetric potential from the substrate of the inner wall of the tube. Therefore, the appearance of split features can be explained by the potential difference among the TbPc2 molecules. Since it is uncertain that the SWCNTs in Fig. 5a and e have the same chirality, it is highly possible that their original DOSs are different. Therefore, a simple comparison is not possible. In other words, the chirality of the SWCNTs must be the same for quantitative experimental investigation. On the other hand, from the information in Fig. 5e and f, it is clear that the DOSs between the part with TbPc2 and the part without it are different, and the electron transport characteristics of SWCNTs can be controlled by encapsulating TbPc2. Thus, it is thought that there are electronic interactions between TbPc2 and SWCNT in the TbPc2@SWCNT. It has been suggested that the electron correlation between TbPc2 and SWCNT can affect the electronic transport properties and/or electromagnetic properties.36,40,54
Conclusions
We encapsulated neutral TbPc2 molecules in SWCNTs by using a capillary method and confirmed the encapsulation by using TEM, STEM, and EDX spectrometry. This is the first report of TbPc2@SWCNT. However, the one-dimensional chain stacking of TbPc2 was not confirmed. It is thought that there are small regions of stacking inside the one-dimensional nano space of SWCNT. TbPc2 has a diameter size of about 2 nm and has a relatively weak affinity for SWCNTs due to its π-conjugated planar structure. Therefore, it does not encapsulate efficiently in one-dimensional stacks in a SWCNT. The frequency dependence due to TbPc2 was shown by using ac magnetic susceptibility measurements, and the activation energy barrier Ueff and pre-exponential factor τ0 estimated from the Arrhenius plot created from the peak top of the imaginary component were determined to be 430 cm−1 and 2.9 × 10−10 s, respectively. The values are consistent with those of pristine TbPc2, suggesting that TbPc2 retains its magnetic properties even in SWCNTs. From this result, it was determined that TbPc2 was in a diluted state in SWCNT. On the other hand, the magnetic hysteresis temperature did not improve after encapsulation, which is because TbPc2 molecules are not stacked in a one-dimensional chain structure. In addition, from the STM/STS, it was determined that there were electronic interactions between the TbPc2 molecules and SWCNTs in TbPc2@SWCNT. Since bandgap modulation was observed due to the encapsulation of TbPc2 in the SWCNTs, these materials should be useful in field effect transistors. We are currently working to improve the encapsulation rate of TbPc2 and the one-dimensional chain structure, which should improve the SMMs properties via ‘local bandgap engineering’. Our work may pave the way for the construction of SMM–CNT hybrid materials, including SMM–CNT spin valves.
Author contributions
M. Y. conceived the idea, coordinated the experimental works. J. S., R. N., Y. K., and T. S. prepared the materials. J. S. and R. N. developed the experimental methodology, the characterization, and analysed the data. J. S., R. N. and K. K. carried out the magnetic measurements and analysed data. F. A. and T. K. carried out the scanning probe microscope measurements and analysed data. K. K., T. K., R. N., B. K. B. and M. Y. discussed the data and wrote the original manuscript. All the authors read and commented on the manuscript.
Conflicts of interest
There are no conflicts to declare.
Acknowledgements
This work was financially supported by a Grant-in-Aid for Young Scientists (B) (grant No. 24750119) and Scientific Research (C) (grant No. 15K05467) and Grant-in-Aid for Scientific Research (S) (Grant No. 20225003, 19H05621) from the Ministry of Education, Culture, Sports, Science, and Technology (MEXT) and CREST(JPMJCR12L3), JST, Japan. A part of this study was supported by Tohoku University Microstructural Characterization Platform in Nanotechnology Platform project sponsored by the MEXT, Japan. M. Y. thanks the 111 Project (B18030) from China for the support.
References
- M. N. Leuenberger and D. Loss, Nature, 2001, 410, 789–793 Search PubMed.
- F. Troiani, A. Ghirri, M. Affronte, S. Carretta, P. Santini, G. Amoretti, S. Piligkos, G. Timco and R. E. P. Winpenny, Phys. Rev. Lett., 2005, 94, 207208 Search PubMed.
- G. Aromí, D. Aguilà, P. Gamez, F. Luis and O. Roubeau, Chem. Soc. Rev., 2012, 41, 537–546 Search PubMed.
- F. Luis, A. Repollés, M. J. Martínez-Pérez, D. Aguilà, O. Roubeau, D. Zueco, P. J. Alonso, M. Evangelisti, A. Camón, J. Sesé, L. A. Barrios and G. Aromí, Phys. Rev. Lett., 2011, 107, 117203 Search PubMed.
-
J. Bartolomée, F. Luis and J. F. Fernández, Molecular magnets: physics and applications, Springer-Verlag/Sci-Tech/Trade, 2013 Search PubMed.
- R. Vincent, S. Klyatskaya, M. Ruben, W. Wernsdorfer and F. Balestro, Nature, 2012, 488, 357–360 Search PubMed.
- F. Troiani and M. Affronte, Chem. Soc. Rev., 2011, 40, 3119–3129 Search PubMed.
- R. Sessoli, D. Gatteschi, A. Caneschi and M. A. Novak, Nature, 1993, 365, 141–143 Search PubMed.
- J. R. Friedman, M. P. Sarachik, J. Tejada and R. Ziolo, Phys. Rev. Lett., 1996, 76, 3830–3833 Search PubMed.
- W. Wernsdorfer and R. Sessoli, Science, 1995, 16, 1315–1325 Search PubMed.
- F. Luis, J. Bartolomé, J. F. Fernández, J. Tejada, J. M. Hernández, X. X. Zhang and R. Ziolo, Phys. Rev. B: Condens. Matter Mater. Phys., 1997, 55, 11448–11456 Search PubMed.
- E. Moreno-Pineda, M. Damjanović, O. Fuhr, W. Wernsdorfer and M. Ruben, Angew. Chem., Int. Ed., 2017, 56, 9915–9919 Search PubMed.
- G. A. Timco, S. Carretta, F. Troiani, F. Tuna, R. J. Pritchard, C. A. Muryn, E. J. L. McInnes, A. Ghirri, A. Candini, P. Santini, G. Amoretti, M. Affronte and R. E. P. Winpenny, Nat. Nanotechnol., 2009, 4, 173–178 Search PubMed.
- C. J. Wedge, G. A. Timco, E. T. Spielberg, R. E. George, F. Tuna, S. Rigby, E. J. L. Mcinnes, R. E. P. Winpenny, S. J. Blundell and A. Ardavan, Phys. Rev. Lett., 2012, 107204 Search PubMed.
- D. Gatteschi and R. Sessoli, Angew. Chem., Int. Ed., 2003, 42, 268–297 Search PubMed.
- N. Ishikawa, M. Sugita, T. Ishikawa, S. Y. Koshihara and Y. Kaizu, J. Am. Chem. Soc., 2003, 125, 8694–8695 Search PubMed.
- F. S. Guo, B. M. Day, Y. C. Chen, M. L. Tong, A. Mansikkamäki and R. A. Layfield, Science, 2018, 362, 1400–1403 Search PubMed.
- C. J. Milios and R. E. P. Winpenny, Struct. Bonding, 2015, 164, 1–110 Search PubMed.
-
C. Benelli and D. Gatteschi, Introduction to molecular magnetism: from transition metals to lanthanides, Wiley, 2015 Search PubMed.
- N. Ishikawa, M. Sugita and W. Wernsdorfer, Angew. Chem., Int. Ed., 2005, 44, 2931–2935 Search PubMed.
- N. Ishikawa, M. Sugita, N. Tanaka, T. Ishikawa, S. Y. Koshihara and Y. Kaizu, Inorg. Chem., 2004, 43, 5498–5500 Search PubMed.
- W. Wernsdorfer, N. Aliaga-Alcalde, D. N. Hendrickson and G. Christou, Nature, 2002, 416, 406–409 Search PubMed.
- K. Katoh, S. Yamashita, N. Yasuda, Y. Kitagawa, B. K. Breedlove, Y. Nakazawa and M. Yamashita, Angew. Chem., Int. Ed., 2018, 57, 9262–9267 Search PubMed.
- T. Yamabayashi, K. Katoh, B. Breedlove and M. Yamashita, Molecules, 2017, 22, 999 Search PubMed.
- S. Iijima, Nature, 1991, 354, 56–58 Search PubMed.
- M. Menon and D. Srivastava, Phys. Rev. Lett., 1997, 79, 4453–4456 Search PubMed.
- S. J. Tans, A. R. M. Verschueren and C. Dekker, Nature, 1998, 393, 49–52 Search PubMed.
- V. I. Preprint, S. W. Emmons and A. M. Leroi, Nature, 1999, 402, 253–254 Search PubMed.
- W. Lu and C. M. Lieber, Nat. Mater., 2007, 6, 841–850 Search PubMed.
- P. M. Ajayan, T. W. Ebbesen, T. Ichihashi, S. Iijima, K. Tanigaki and H. Hiura, Nature, 1993, 362, 522–525 Search PubMed.
- E. Dujardin, T. W. Ebbesen, H. Hiura and K. Tanigaki, Science, 1994, 265, 1850–1852 Search PubMed.
- B. W. Smith, M. Monthioux and D. E. Luzzi, Nature, 1998, 396, 323–324 Search PubMed.
- T. Pichler, H. Kuzmany, H. Kataura and Y. Achiba, Phys. Rev. Lett., 2001, 87, 267401 Search PubMed.
- S. Bandow, M. Takizawa, K. Hirahara, M. Yudasaka and S. Iijima, Chem. Phys. Lett., 2001, 337, 48–54 Search PubMed.
- R. Kitaura, N. Imazu, K. Kobayashi and H. Shinohara, Nano Lett., 2008, 8, 693–699 Search PubMed.
- L. Bogani and W. Wernsdorfer, Nat. Mater., 2008, 7, 179–186 Search PubMed.
- M. del Carmen Giménez-López, F. Moro, A. La Torre, C. J. Gómez-García, P. D. Brown, J. van Slageren and A. N. Khlobystov, Nat. Commun., 2011, 2, 407 Search PubMed.
- R. Nakanishi, M. Yatoo, K. Katoh, B. Breedlove and M. Yamashita, Materials, 2017, 10, 7 Search PubMed.
- S. Kyatskaya, J. R. G. Mascarós, L. Bogani, F. Hennrich, M. Kappes, W. Wernsdorfer and M. Ruben, J. Am. Chem. Soc., 2009, 131, 15143–15151 Search PubMed.
- M. Urdampilleta, S. Klyatskaya, J. P. Cleuziou, M. Ruben and W. Wernsdorfer, Nat. Mater., 2011, 10, 502–506 Search PubMed.
- R. Nakanishi, J. Satoh, K. Katoh, H. Zhang, B. K. Breedlove, M. Nishijima, Y. Nakanishi, H. Omachi, H. Shinohara and M. Yamashita, J. Am. Chem. Soc., 2018, 140, 10955–10959 Search PubMed.
- K. Katoh, Y. Yoshida, M. Yamashita, H. Miyasaka, B. K. Breedlove, T. Kajiwara, S. Takaishi, N. Ishikawa, H. Isshiki, F. Z. Yan, T. Komeda, M. Yamagishi and J. Takeya, J. Am. Chem. Soc., 2009, 131, 9967–9976 Search PubMed.
-
R. Weiss and J. Fischer, The Porphyrin Handbook, Elsevier, 2003, pp. 171–246 Search PubMed.
- T. Saito, S. Ohshima, T. Okazaki, S. Ohmori, M. Yumura and S. Iijima, J. Nanosci. Nanotechnol., 2008, 8, 6153–6157 Search PubMed.
- T. Saito, W. C. Xu, S. Ohshima, H. Ago, M. Yumura and S. Iijima, J. Phys. Chem. B, 2006, 110, 5849–5853 Search PubMed.
- Y. Li, T. Wang, H. Meng, C. Zhao, M. Nie, L. Jiang and C. Wang, Dalton Trans., 2016, 45, 19226–19229 Search PubMed.
- J. Lee, H. Kim, S. J. Kahng, G. Kim, Y. W. Son, J. Ihm, H. Kato, Z. W. Wang, T. Okazaki, H. Shinohara and Y. Kuk, Nature, 2002, 415, 1005–1008 Search PubMed.
- D. J. Hornbaker, S. J. Kahng, S. Misra, B. W. Smith, A. T. Johnson, E. J. Mele, D. E. Luzzi and A. Yazdani, Science, 2002, 295, 828–831 Search PubMed.
- Y. Cho, S. Han, G. Kim, H. Lee and J. Ihm, Phys. Rev. Lett., 2003, 90, 106402 Search PubMed.
- C. L. Kane, E. J. Mele, A. T. Johnson, D. E. Luzzi, B. W. Smith, D. J. Hornbaker and A. Yazdani, Phys. Rev. B: Condens. Matter Mater. Phys., 2002, 66, 235423 Search PubMed.
- T. Shimada, T. Okazaki, R. Taniguchi, T. Sugai, H. Shinohara, K. Suenaga, Y. Ohno, S. Mizuno, S. Kishimoto and T. Mizutani, Appl. Phys. Lett., 2002, 81, 4067–4069 Search PubMed.
- M. Furuhashi and T. Komeda, Phys. Rev. Lett., 2008, 101, 185503 Search PubMed.
- T. Komeda, H. Isshiki, J. Liu, K. Katoh and M. Yamashita, ACS Nano, 2014, 8, 4866–4875 Search PubMed.
- M. Yamashita, Bull. Chem. Soc. Jpn., 2021, 94, 209–264 Search PubMed.
Footnote |
† Electronic supplementary information (ESI) available. See DOI: 10.1039/d1tc01026c |
|
This journal is © The Royal Society of Chemistry 2021 |
Click here to see how this site uses Cookies. View our privacy policy here.