DOI:
10.1039/D1TC00604E
(Paper)
J. Mater. Chem. C, 2021,
9, 11180-11188
A switchable multimode microlaser based on an AIE microsphere†
Received
7th February 2021
, Accepted 21st June 2021
First published on 21st June 2021
Abstract
Switchable multimode microlasers are of great significance to the development of photonic devices with high integration levels. Herein, we demonstrate an acid/alkaline gas-responsive multimode AIEgen@starch microsphere-based microlaser. The aggregation-induced emission (AIE) active fluorescent dye ASCPI was used as the gain medium in this study. ASCPI was weakly emissive in water but became highly emissive when introduced to a starch microsphere as a guest molecule. The resultant ASCPI@starch microsphere worked well as a typical whispering-gallery-mode microlaser. The laser mode wavelengths were size-dependent. Due to the sensitivity of ASCPI to pH, the output of the microlaser could be switched to a shorter wavelength by acetic acid vapor treatment or a longer wavelength by NH3 vapor treatment. This work will provide useful enlightenment for the rational design of effective switchable lasers using AIE materials with simple preparation procedures.
Introduction
Miniaturized lasers have gained increasing interest owing to their applications in chemical and biological sensing,1–4 laser display,5–7 imaging,8 and on-chip optical interconnects.9–12 Wavelength-tuneable lasers with the capability of delivering intense coherent light signals across a broad spectral range open up particularly interesting opportunities for highly integrated photonic devices. In the past few decades, laser switches have already been achieved by regulating the resonant mode via approaches like tuning the size or refractive index of the microcavities.13 Besides, tailoring the gain region of lasing materials has also been developed as a promising alternative approach to switching the lasing wavelength. However, for a traditional semiconductor, its energy band structure is generally fixed, which makes it difficult to dynamically modulate its gain region to realize laser switches. In contrast, organic materials with abundant energy levels and tailorable excited-state processes show great potential for tailoring the gain region, and thus enabling the fabrication of wavelength-switchable microlasers.14–19 Facile fabrication and excellent compatibility of organic materials are also advantageous compared with those of conventional semiconducting materials. To date, wavelength-tunable lasers based on organic materials can be realized by modulating the energy level of an excited state20–24 or mode variation of the optical resonator.15,25–27 The excited-state energy levels of organic gain materials are sensitive to surrounding environments, thereby enabling the development of feasible methods for controllably switching the lasing wavelength via tuning the external stimuli. Previous reports have demonstrated that laser wavelength could be tuned by external stimuli, including humidity,28 temperature,23,29 light,30 vapor,20,21 solvent,31etc. However, reports on gas-responsive micro-/nanolasers are limited.20,21,31
Among organic gain materials, aggregation-induced emission luminogens (AIEgens) are a new type of luminescent materials, which are non-emissive when molecularly dissolved, but induced to luminesce by aggregate formation.32–38 AIEgens used as optical gain materials of microlasers can take advantage of the inherent AIE characteristics to improve lasing performance, including drastic threshold reduction and favorable lasing stability.20,39–41 In addition, these AIEgen-based miniaturized lasers can improve their laser performance with much higher doping concentrations.39 However, there are only a few reports of AIEgen-based miniaturized lasers.39,42 Reports of the environment-responsive emission behavior of AIEgen-based miniaturized lasers are more limited in the literature. The excited-state intramolecular charge transfer (ICT)43–46 effect endows AIEgens with high sensitivity to the microenvironment. Therefore, AIEgens with ICT effect may be ideal candidates to fabricate robust wavelength-switchable microlasers.
Herein, we report a switchable multimode microlaser based on the ASCPI@starch microsphere. The cationic AIEgen, ASCPI, was originally weakly emissive in water solution due to its AIE and ICT properties. When mixed with starch granules, ASCPI could readily bind to the starch. The host–guest interaction restricted the intramolecular motions of the AIE molecules and strong fluorescence was triggered with blue-shifted emission color. An acidic gas could protonate ASCPI, which would consequently weaken the electron density of the donor system and therefore lead to a blue-shift of the gain region. In contrast, an alkaline gas (NH3) provided a more polar environment for these ICT chromophores and thus led to a red-shifted gain behavior. As a result, we discovered a method of tuning lasing wavelength in the starch microparticles based on the acid/base-responsive AIEgen. These results offer a novel understanding of the stimulated emission of AIEgens and provide useful enlightenment for the rational design of miniaturized lasers with desired performance.
Experimental section
AIEgen preparation
AIEgen (ASCPI) was synthesized according to the previous literature.47
Extraction process of potato starch granules
Potato starch granules were extracted from a fresh potato purchased at a local market. The potato was sliced into flakes, soaked in pure water, and vigorously shaken for 30–60 min. Potato starch granules were collected by centrifuging the solution (4000 rpm for 5 min), followed by further washing and purification through three centrifugation cycles with pure water. Finally, the as-prepared fresh potato starch granules were collected and dried in an oven at 40 °C.
Preparation of ASCPI@starch
0.4 mg of starch granules were immersed in 1 mL of pure water and sonicated for 5 s. Then 10 μL of ASCPI (10−3 M in DMSO) was dropped into the starch/H2O solution, shaken by hand and left at ambient temperature for 24 h. The mixture was centrifuged and washed with pure water several times until no characteristic emission was observed in the supernatant upon excitation, and then dried in an oven at 40 °C.
Measurements
The absorption and fluorescence spectra were measured with a PerkinElmer Lambda-950 spectrophotometer and an Edinburgh FLS-1000 instrument, respectively. The time-resolved photoluminescence (TRPL) was measured with an Edinburgh FLS-1000 spectrofluorometer system equipped with an EPLED-360. The absolute luminescence quantum yield was measured on an Edinburgh FLS 980 fluorescence spectrophotometer equipped with an integrating sphere (0.1 nm step size, 0.3 second integration time, and 5 repeats). The morphology of the microsphere was examined with scanning electron microscopy (SEM, FEI Nova NanoSEM450). X-ray diffraction measurements were conducted on a D/max-2550 PC X-ray diffractometer with Cu Kα radiation. Bright-field optical images and fluorescence microscopy images were taken using an inverted fluorescence microscope (Nikon Ti-U), by exciting the samples with a mercury lamp. Lasing measurements were performed on a home-built far-field microphotoluminescence system. The microsphere was locally excited with a focused 400 nm femtosecond laser (fs-laser), which was generated from the second harmonic of the fundamental output of a regenerative amplifier (Solstice, Spectra-Physics, 800 nm, 100 fs, and 1 kHz).
Results and discussion
A typical AIEgen sensitive to pH was chosen as a model molecule to demonstrate the concept. Fig. 1a shows the chemical structure of the AIEgen, 4-(4-(1-cyano-2-(4-(dimethylamino)phenyl)vinyl)phenyl)-1-methylpyridin-1-ium iodide (ASCPI). ASCPI was synthesized according to a previous method.47 As illustrated in Fig. 1b, a typical AIE property was observed. Its dilute solution in DMSO exhibited weak photoluminescence (PL) with an emission peak at 674 nm. Upon addition of non-polar 1,4-dioxane as a poor solvent for ASCPI into the DMSO solution with a fraction of 90 vol%, bright red PL with a emission maximum at 650 nm was observed. In addition, the αAIE value of ASCPI (Fig. 1c) was calculated to be 9.3 when fd (1,4-dioxane fraction) was equal to 90%, suggesting a typical AIE feature for this molecule. Moreover, ASCPI demonstrated a large Stokes shift (Δν = 192 nm), which can be attributed to an excited-state ICT between the electron donor and the electron acceptor. The ICT effect was also confirmed by its solvatochromic effects44,45 (Fig. S1, ESI†). ASCPI presented varied emission spectra in different solvents with wavelength changing from 666 nm to 688 nm, indicating a typical charge transfer characteristic in the excited state (Fig. S1b, ESI†).
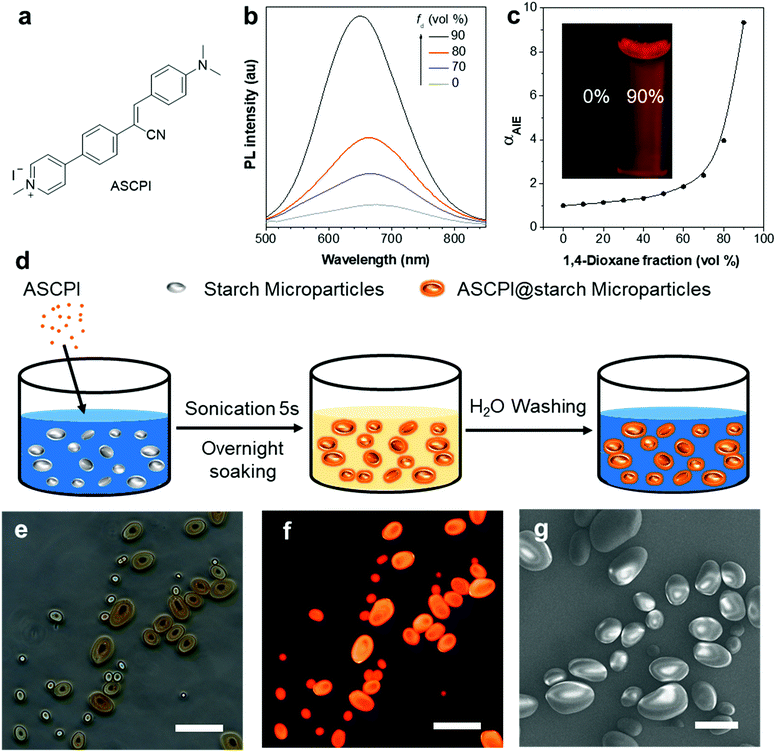 |
| Fig. 1 (a) Molecular structure of ASCPI. (b) PL spectra of ASCPI in DMSO/1,4-dioxane mixtures with different 1,4-dioxane fractions (fd = 0, 70%, 80%, and 90%). (c) Plot of relative emission peak intensity of ASCPI versus fd of DMSO/1,4-dioxane mixtures, where αAIE = I/I0, I = emission intensity and I0 = emission intensity in DMSO solution. Inset: fluorescence images of ASCPI in DMSO/1,4-dioxane mixtures with different fd taken under 365 nm UV irradiation from a hand-held UV lamp at room temperature. Conditions: solution concentration: 10−5 M; excitation wavelength: 440 nm. (d) Illustration of the fabrication of ASCPI@starch microparticles. (e) Bright-field microscopy image, (f) fluorescence microscopy image, and (g) SEM image of the ASCPI@starch microparticles. Scale bar: 50 μm. | |
Starch granules usually have spherical, ellipsoidal, or polygonal shapes,48,49 which can function as whispering gallery mode (WGM) resonators providing optical feedback for laser oscillations.25,50–53 In this study, we explored the possibility of fabricating an AIEgen-based microlaser using starch granules as the WGM resonators. The synthetic procedure of ASCPI@starch microparticles is illustrated in Fig. 1d. Starch granules were sonicated in water. ASCPI was then added into the starch/H2O solution, shaken by hand and left at ambient temperature for 24 h. The obtained ASCPI@starch particles were centrifuged and washed with pure water. The doping concentration of ASCPI was 1.1 wt% in the starch microparticles. As shown in the bright-field image (Fig. 1e), micro-sized ASCPI@starch particles with an ellipsoidal shape could be obtained. ASCPI was weakly emissive in water (Fig. S2, ESI†). This was possibly attributed to both its AIE activity and ICT property. Since this probe carried a positive charge, it was loosely packed in water. The relatively active intramolecular motions weakened its emission. Moreover, due to its ICT property, its emission was further quenched by water. Therefore, its emission in water was rather weak. When interacting with starch granules, the dye bound to the starch and the strong host–guest interaction between the starch and dye not only restricted the intramolecular motions of the AIEgen but also changed its microenvironment. Therefore, the fluorescence of ASCPI in the starch granules was turned on (Fig. S2, ESI†). As shown in Fig. 1f, bright orange-red fluorescence of the particles could be observed under UV excitation, revealing that the ASCPI molecules were successfully encapsulated by starch granules. The scanning electron microscopy (SEM) image (Fig. 1g) showed that these ASCPI@starch microparticles possessed perfect ellipsoidal shapes and ultra-smooth surfaces, possibly triggering WGM lasing at a low threshold.
We then tested the optically pumped laser behaviours of these ASCPI@starch microparticles. Here the ASCPI@starch microparticle with dimensions of 10.7 μm (major axis) × 8.89 μm (minor axis) was excited by a femtosecond pulsed laser beam (λ = 400 nm, and 200 fs) with increasing fluence. At low pump fluence, the ASCPI@starch microparticle exhibited a broad emission, which was peaked at about 636 nm, corresponding to spontaneous emission (Fig. 2a). At higher pump fluence, the emission spectrum of the ASCPI@starch microparticle exhibited a set of sharp peaks at about 680 nm accompanied by a dramatically enhanced intensity, which indicated stimulated emission. The corresponding PL peak intensity versus pump fluence (Fig. 2b) revealed a clear threshold behaviour at about 68.5 mJ cm−2, which further confirmed the lasing action in the microparticles. Above the lasing threshold, the full width at half-maximum (FWHM) at 682 nm dramatically narrowed down to ∼0.4 nm, revealing a sharp increase of temporal coherence. We also recorded the lasing intensities of the ASCPI@starch microlasers with the excitation fluence of 1.2 Pth to evaluate the stability of the prepared microlasers. The ASCPI@starch microparticles were continuously illuminated with a pulsed laser beam excitation (duration time, 100 fs; repetition rate, 1 kHz). As shown in Fig. S3 (ESI†), sustained operation of the microlaser was achieved after scanning 7000 times, accompanied by a moderate decrease in lasing intensity. This reduction of lasing intensity with increasing scanning times could be ascribed to the bleaching of dye molecules.54,55
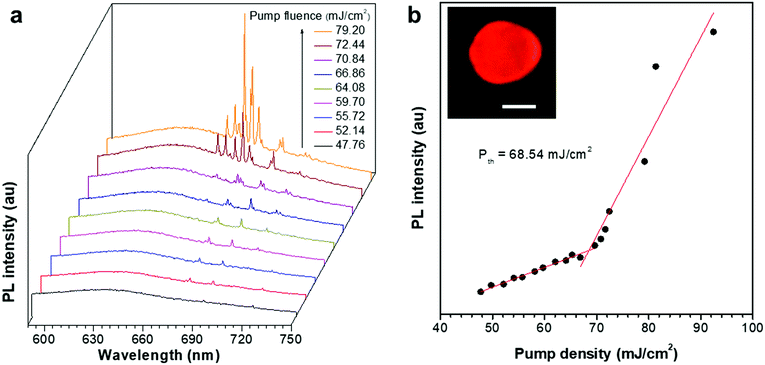 |
| Fig. 2 (a) PL spectra recorded at the edge of an ASCPI@starch microparticle as a function of pump density. (b) Plot of PL peak intensity as a function of pump density. Inset: fluorescence image of the ASCPI@starch microparticle (major axis 10.7 μm × minor axis 8.89 μm) under 400 nm pulse laser excitation. Scale bar is 10 μm. | |
The lasing spectra of the ASCPI@starch microparticles with different diameters were further studied to investigate the microcavity effects. Fig. 3a illustrates the PL spectra and the corresponding images of the three ASCPI@starch microparticles with different dimensions. The mode spacing (Δλm) between two adjacent lasing modes decreased as the microparticle circumference (L) increased from 44.6 to 186 μm (Fig. 3a). For WGM resonance, Δλm and L satisfied the equation of Δλm = λ2/ngL,25 where λ was the wavelength of guided light, and ng was the group refractive index. As shown in Fig. 3b, the plot of Δλm and 1/L showed a clear linear relationship. By fitting the plot, the group refractive index ng was identified with a value of ∼1.58 at λ = 682 nm (Fig. 3b), which was consistent with the intrinsic refractive index of the starch polymer (1.53).56 These microcavity effect results verified the WGM-type cavity resonance in the ASCPI@starch microparticles. The simulated electric field intensity distribution shown in Fig. 3c further confirmed the existence of WGM in the microparticles.
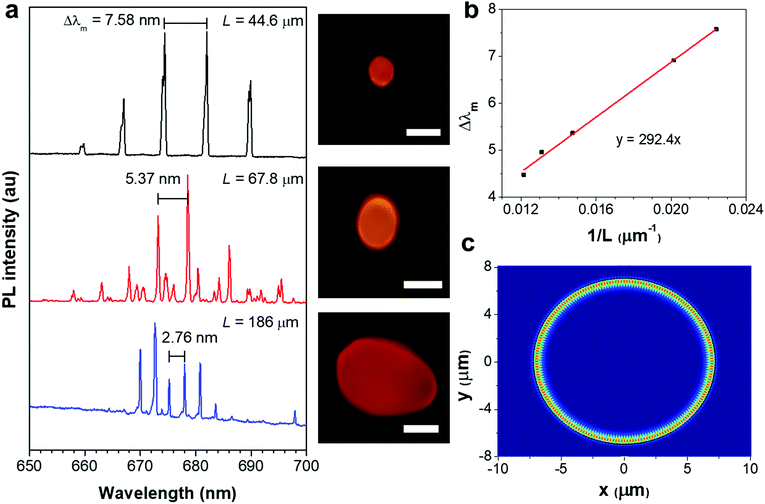 |
| Fig. 3 (a) PL spectra of the ASCPI@starch microparticles with three different sizes. Corresponding fluorescence images are depicted as insets. All scale bars are 20 μm. (b) Relationship between Δλm and 1/L of the ASCPI@starch microparticles. L is the cavity length. The red line is a fitting to the function Δλm = λ2/ngL, where λ = 682 nm. (c) Simulated field intensity distribution (λ, 682 nm) in an ASCPI@starch microparticle with length of 44.6 μm. | |
As the lasing wavelength is related to the energy gap of the optical gain material, it is possible to control the gain region by tuning the energy levels of AIEgens. The ASCPI@starch microparticle exhibited a broad emission with the wavelength peak at 638 nm (Fig. 4a). After long-time exposure to HAc, the fluorescence peak of the ASCPI@starch microparticle exhibited a blue-shift from 638 to 633 nm along with a decrease of photoluminescence quantum yield (PLQY) from 14.7% to 10.6%. In addition, the photoluminescence of the ASCPI@starch microparticle showed a bathochromic shift of the maximum emission wavelength from 638 to 646 nm (Fig. 4a) and the PLQY decreased from 14.7% to 7.6% in an NH3 atmosphere. This suggested that the external acid/alkaline gas in the atmosphere could tune the energy levels of AIEgens in the ASCPI@starch microparticles. In addition, the fluorescence lifetime of ASCPI@starch microparticles was also investigated to elucidate the inherent excited state property. As shown in Fig. 4b, the fluorescence lifetime of ASCPI@starch microparticles was 1.41 ns. Upon exposure to HAc and NH3, however, the fluorescence lifetimes of the ASCPI@starch microparticles decreased to 1.03 and 1.30 ns, respectively. According to the equation knr = (1 − φ)/τ, where φ was the PLQY, knr was the non-radiative decay rate constant, and τ was the fluorescence lifetime,57 the non-radiative decay rate constant (knr) of the ASCPI@starch microparticle increased after exposure to HAc and NH3 (Table S1, ESI†). This revealed that the intramolecular rotational motions of AIEgens non-radiatively dissipated the excited-state energy in loose packings of microparticles in a HAc or NH3 atmosphere. To gain more insights into the photophysical mechanism of wavelength switching in the HAc or NH3 atmosphere, the frontier molecular orbital distributions and energy levels of ASCPI were investigated by density functional theory (DFT) using the M062X/def2vp method (Fig. 4c).58 According to the calculation results, the energy gap of protonated ASCPI was 5.50 eV via combining with protonic acid, which was higher than that in a vacuum (3.26 eV), showing good agreement with the corresponding optical spectra after the treatment with HAc vapours. However, the energy gap of ASCPI was 2.85 eV in the NH3 environment, which was lower than that in a vacuum (3.26 eV) due to the ICT effect. Therefore, a red-shifted PL was observed in the optical spectra (Fig. 4a). Fourier transform infrared spectra (FTIR) of ASCPI, ASCPI + HAc and ASCPI + NH3 H2O were also recorded. As illustrated in Fig. S4 (ESI†), the FTIR transmittance spectra of the ASCPI showed a C
N stretching vibrational band at 2200 cm−1, which proved that a cyano structure was contained in the ASPCI compound. In addition, the C
O stretching vibrational band at 1710 cm−1 was observed after treatment with acetic acid, demonstrating that a complex of ASCPI@HAc was formed. Moreover, an obvious shift of the benzene skeleton vibration from 1562 cm−1 to 1574 cm−1 was observed. This may be attributed to the conformational changes due to the ICT effect in the conjugation system after treatment with ammonia.
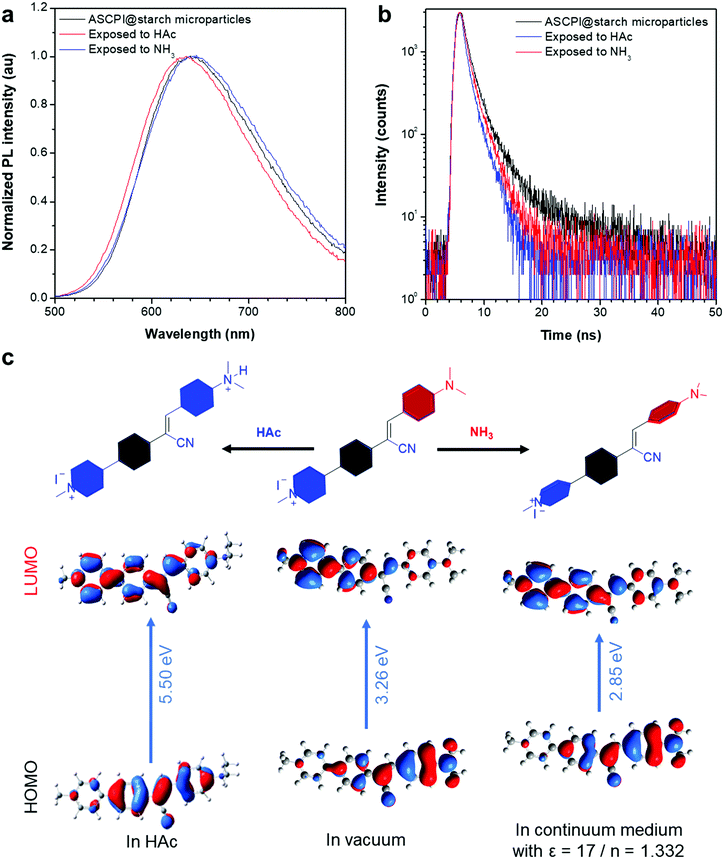 |
| Fig. 4 (a) PL spectra of the ASCPI@starch microparticles, and ASCPI@starch microparticles in HAc and in NH3 atmospheres. (b) PL decay profiles of the ASCPI@starch microparticles without/with exposure to HAc and NH3. (c) Pictorial representations of the HOMOs and LUMOs in the ASCPI, ASCPI–HAc complex and ASCPI in an NH3 atmosphere. | |
Inspired by the HAc/NH3-responsive emission, we measured the lasing spectra in response to the variation of the HAc or NH3 atmosphere (Fig. 5a). Fig. 5b showed the lasing spectra of a typical ASCPI@starch microsphere (as shown in the dotted portion of Fig. 5c) with alternating plugs of air, HAc and NH3 gases. The lasing wavelength of the ASCPI@starch microsphere was tuned from about 679 to 676 nm when the microsphere was exposed to HAc gas (Fig. 5b). Once the ASCPI@starch microsphere was exposed to NH3 gas, the lasing wavelength was changed to about 691 nm (Fig. 5b). Additionally, as the HAc or NH3 vapor in the surrounding medium evaporated in air after 10 min, the PL images of the ASCPI@starch microsphere did not show obvious color change due to the weak fluorescence wavelength switching (Fig. 5c). More importantly, compared with the fluorescence wavelength switching, the microlaser used as a sensor possessed higher sensitivity to HAc and NH3 gas stimuli, which could be ascribed to the narrower FWHM in the lasing spectra (Fig. 4a and 5b). To further confirm the adaptability of the HAc/NH3-responsive stimulated lasing properties, ASCPI@starch microspheres with different sizes were investigated under an external HAc or NH3 atmosphere (Fig. S5 and S6, ESI†). As shown in Fig. S5 (ESI†), about 3 nm of blue-shift was observed after the exposure of the ASCPI@starch microsphere to HAc gas. On the other hand, the other sizes of the ASCPI@starch microspheres exhibited about a 7 nm bathochromic-shift after exposure of the microspheres to NH3 gas (Fig. S6, ESI†). Overall, stimulated laser emission responsive to HAc/NH3 gas could be observed in the AIEgen@starch microspheres.
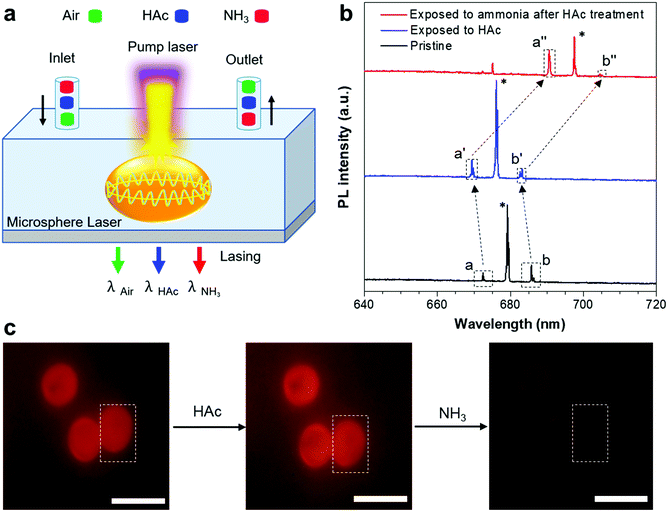 |
| Fig. 5 (a) Schematic diagram of the ASCPI@starch microsphere laser measurement under exposure to different atmospheres. (b) Wavelength shift of lasing modes in the ASCPI@starch microsphere under alternate exposure to HAc, NH3 and air, respectively. (c) Fluorescence images of an ASCPI@starch microsphere under alternate exposure to HAc, NH3 and air. Scale bars: 20 μm. | |
To eliminate the influence of the starch resonator in these switching processes, the X-ray diffraction (XRD) patterns of the ASCPI@starch microspheres treated with acetic acid or ammonia were also investigated, as shown in Fig. S7 (ESI†). The XRD pattern of the ASCPI@starch microparticles contained a series of peaks at 5.86, 17.2, 22.5 and 24.0°, which was in agreement with those of A-type starches.59 It was also found that the diffraction peaks at 2θ = 17.2, 22.5 and 24.0° of the ASCPI@starch microparticles had no obvious changes after treatment with acetic acid or ammonia, suggesting that the structure of A-type starches could be maintained without influence from the presence of NH3 or HAc. In addition, the diffraction peak at 2θ = 5.86° disappeared, because the long-range ordered structure of A-type starches was destroyed during HAc treatment. These data implied that starch used as a WGM resonator had little effect on lasing wavelength switching even with treatment with acid or alkali.
Conclusions
In summary, we report an AIEgen-based switchable microlaser. This microlaser could be easily fabricated by mixing the AIE active dye ASCPI and starch granules. In the confined environment after binding to the host molecule, starch, the emission of the AIE dye ASCPI is greatly enhanced. We demonstrate that the resultant AIEgen@starch microspheres function as typical WGM lasers. More interestingly, manipulation of the laser emission can be achieved by HAc/NH3 gas treatments. The protonic acid gas could weaken the electron density of the donor system and leads to blue-shifted laser behavior. On the other hand, alkaline gas (NH3) provides a more polar environment for these ICT chromophores and thus leads to red-shifted lasing. This work presents a simple method for the fabrication of efficient AIEgen-based switchable microlasers. It is expected that more AIE-based microlasers and AIE-microlaser-based chemo- or bio-sensors will be developed in the near future.
Author contributions
S. Chen. Y. S. Zhao. P. Lai and H. Gao conceived the idea and designed the study. F. Song carried out SEM, XRD, UV, PL, PLQY and lifetime experiments. C. Zhang, H. Dong and Y. Fan preformed the lasing measurements. M.-Y. Wu synthesized the ASPCI compound. H. Gao carried out ASCPI@starch microsphere synthesis. G. Shan carried out the DFT calculations. S. Chen and Y. S. Zhao supervised this work. All the authors were involved in discussion of the results and manuscript preparation. F. Song, H. Gao, C. Zhang, S. Chen, and P. Lai drafted the manuscript with input and comments from all the authors.
Conflicts of interest
The authors declare no competing financial interest.
Acknowledgements
We would like to thank Dr. Chuen Kam and Dr Engui Zhao for critical reading of the manuscript. C. Zhang acknowledges the financial support from the National Natural Science Foundation of China (Grant No. 51903238). H. Gao gratefully acknowledges the financial support from the Fundamental Research Funds of Beihang University (Grant No. KG16007908). S. Chen and P. Lai acknowledge the support from the Innovation and Technology Commission (ITS/022/18, ITC, HK). S. Chen acknowledges the start-up funding from the Ming Wai Lau Centre for Reparative Medicine, Karolinska Institutet.
Notes and references
- R.-M. Ma, S. Ota, Y. Li, S. Yang and X. Zhang, Nat. Nanotechnol., 2014, 9, 600–604 CrossRef CAS PubMed.
- L. He, Ş. K. Özdemir, J. Zhu, W. Kim and L. Yang, Nat. Nanotechnol., 2011, 6, 428–432 CrossRef CAS PubMed.
- M. Humar and S. Hyun Yun, Nat. Photonics, 2015, 9, 572–576 CrossRef CAS PubMed.
- G.-Q. Wei, X.-D. Wang and L.-S. Liao, Laser Photonics Rev., 2020, 14, 2000257 CrossRef CAS.
- F. Fan, S. Turkdogan, Z. Liu, D. Shelhammer and C. Z. Ning, Nat. Nanotechnol., 2015, 10, 796–803 CrossRef CAS PubMed.
- Z. Liu, L. Yin, H. Ning, Z. Yang, L. Tong and C.-Z. Ning, Nano Lett., 2013, 13, 4945–4950 CrossRef CAS PubMed.
- Z. Zhou, J. Zhao, Y. Du, K. Wang, J. Liang, Y. Yan and Y. S. Zhao, Angew. Chem., Int. Ed., 2020, 59, 11814–11818 CrossRef CAS PubMed.
- A. Khalatpour, A. K. Paulsen, C. Deimert, Z. R. Wasilewski and Q. Hu, Nat. Photonics, 2021, 15, 16–20 CrossRef CAS.
- L. Zhao, Y. Gao, M. Su, Q. Shang, Z. Liu, Q. Li, Q. Wei, M. Li, L. Fu, Y. Zhong, J. Shi, J. Chen, Y. Zhao, X. Qiu, X. Liu, N. Tang, G. Xing, X. Wang, B. Shen and Q. Zhang, ACS Nano, 2019, 13, 10085–10094 CrossRef CAS PubMed.
- Y. Mi, Y. Zhong, Q. Zhang and X. Liu, Adv. Opt. Mater., 2019, 7, 1900544 CrossRef.
- X. Yang, Z. Shan, Z. Luo, X. Hu, H. Liu, Q. Liu, Y. Zhang, X. Zhang, M. Shoaib, J. Qu, X. Yi, X. Wang, X. Zhu, Y. Liu, L. Liao, X. Wang, S. Chen and A. Pan, ACS Nano, 2020, 14, 3397–3404 CrossRef CAS PubMed.
- H. Dong, C. Zhang, X. Liu, J. Yao and Y. S. Zhao, Chem. Soc. Rev., 2020, 49, 951–982 RSC.
- A. Camposeo, P. Del Carro, L. Persano and D. Pisignano, Adv. Mater., 2012, 24, OP221–OP225 CrossRef CAS PubMed.
- H. Dong, C. Zhang and Y. S. Zhao, Adv. Opt. Mater., 2019, 7, 1900037 CrossRef.
- J. Zhao, Y. Yan, C. Wei, W. Zhang, Z. Gao and Y. S. Zhao, Nano Lett., 2018, 18, 1241–1245 CrossRef CAS PubMed.
- C. Zhang, C.-L. Zou, H. Dong, Y. Yan, J. Yao and Y. S. Zhao, Sci. Adv., 2017, 3, e1700225 CrossRef PubMed.
- Y. Lv, Z. Xiong, H. Dong, C. Wei, Y. Yang, A. Ren, Z. Yao, Y. Li, S. Xiang, Z. Zhang and Y. S. Zhao, Nano Lett., 2020, 20, 2020–2025 CrossRef CAS PubMed.
- C.-C. Yan, X.-D. Wang and L.-S. Liao, ACS Photonics, 2020, 7, 1355–1366 CrossRef CAS.
- J.-J. Wu, X.-D. Wang and L.-S. Liao, ACS Photonics, 2019, 6, 2590–2599 CrossRef CAS.
- Y. Lv, Z. Xiong, Z. Yao, Y. Yang, S. Xiang, Z. Zhang and Y. S. Zhao, J. Am. Chem. Soc., 2019, 141, 19959–19963 CrossRef CAS PubMed.
- Z. Gao, W. Zhang, Y. Yan, J. Yi, H. Dong, K. Wang, J. Yao and Y. S. Zhao, ACS Nano, 2018, 12, 5734–5740 CrossRef CAS PubMed.
- H. Dong, C. Zhang, X. Lin, Z. Zhou, J. Yao and Y. S. Zhao, Nano Lett., 2017, 17, 91–96 CrossRef CAS PubMed.
- H. Dong, Y. Wei, W. Zhang, C. Wei, C. Zhang, J. Yao and Y. S. Zhao, J. Am. Chem. Soc., 2016, 138, 1118–1121 CrossRef CAS PubMed.
- W. Zhang, Y. Yan, J. Gu, J. Yao and Y. S. Zhao, Angew. Chem., Int. Ed., 2015, 54, 7125–7129 CrossRef CAS PubMed.
- Y. Wei, X. Lin, C. Wei, W. Zhang, Y. Yan and Y. S. Zhao, ACS Nano, 2017, 11, 597–602 CrossRef CAS PubMed.
- P. Görrn, M. Lehnhardt, W. Kowalsky, T. Riedl and S. Wagner, Adv. Mater., 2011, 23, 869–872 CrossRef PubMed.
- M. Karl, J. M. E. Glackin, M. Schubert, N. M. Kronenberg, G. A. Turnbull, I. D. W. Samuel and M. C. Gather, Nat. Commun., 2018, 9, 1525 CrossRef PubMed.
- Q. Huang, H. Xu, M. Li, Z. Hou, C. Lv, X. Zhan, H. Li, H. Xia, H. Wang and H. Sun, J. Lightwave Technol., 2018, 36, 819–824 CAS.
- L. Zhao, Y. Wang, Y. Yuan, Y. Liu, S. Liu, W. Sun, J. Yang and H. Li, Opt. Commun., 2017, 402, 181–185 CrossRef CAS.
- C. Qiao, C. Zhang, Z. Zhou, H. Dong, Y. Du, J. Yao and Y. S. Zhao, Angew. Chem., Int. Ed., 2020, 59, 15992–15996 CrossRef CAS PubMed.
- Y. Wei, H. Dong, C. Wei, W. Zhang, Y. Yan and Y. S. Zhao, Adv. Mater., 2016, 28, 7424–7429 CrossRef CAS PubMed.
- J. Luo, Z. Xie, J. W. Y. Lam, L. Cheng, H. Chen, C. Qiu, H. S. Kwok, X. Zhan, Y. Liu, D. Zhu and B. Z. Tang, Chem. Commun., 2001, 1740–1741 RSC.
- J. Mei, N. L. C. Leung, R. T. K. Kwok, J. W. Y. Lam and B. Z. Tang, Chem. Rev., 2015, 115, 11718–11940 CrossRef CAS PubMed.
- Z. Zhao, H. Zhang, J. W. Y. Lam and B. Z. Tang, Angew. Chem., Int. Ed., 2020, 59, 9888–9907 CrossRef CAS PubMed.
- B. Liu and B. Z. Tang, Angew. Chem., Int. Ed., 2020, 59, 9788–9789 CrossRef CAS PubMed.
- H. Zhang, Z. Zhao, A. T. Turley, L. Wang, P. R. McGonigal, Y. Tu, Y. Li, Z. Wang, R. T. K. Kwok, J. W. Y. Lam and B. Z. Tang, Adv. Mater., 2020, 32, 2001457 CrossRef CAS PubMed.
- J. Yang, M. Fang and Z. Li, Aggregate, 2020, 1, 6–18 CrossRef.
- F. Song, Z. Zhao, Z. Liu, J. W. Y. Lam and B. Z. Tang, J. Mater. Chem. C, 2020, 8, 3284–3301 RSC.
- W. Liu, H. Yu, R. Hu, T. Xu, Y. Lun, J. Gan, S. Xu, Z. Yang and B. Z. Tang, Small, 2020, 16, 1907074 CrossRef CAS PubMed.
- C. Wei, S.-Y. Liu, C.-L. Zou, Y. Liu, J. Yao and Y. S. Zhao, J. Am. Chem. Soc., 2015, 137, 62–65 CrossRef CAS PubMed.
- G.-Q. Wei, Y.-C. Tao, J.-J. Wu, Z.-Z. Li, M.-P. Zhuo, X.-D. Wang and L.-S. Liao, J. Phys. Chem. Lett., 2019, 10, 679–684 CrossRef PubMed.
- J. Gierschner, S. Varghese and S. Y. Park, Adv. Opt. Mater., 2016, 4, 348–364 CrossRef CAS.
- Z. Zhao, H. Su, P. Zhang, Y. Cai, R. T. K. Kwok, Y. Chen, Z. He, X. Gu, X. He, H. H. Y. Sung, I. D. Willimas, J. W. Y. Lam, Z. Zhang and B. Z. Tang, J. Mater. Chem. B, 2017, 5, 1650–1657 RSC.
- S. Liu, X. Zhou, H. Zhang, H. Ou, J. W. Y. Lam, Y. Liu, L. Shi, D. Ding and B. Z. Tang, J. Am. Chem. Soc., 2019, 141, 5359–5368 CrossRef CAS PubMed.
- F. Song, Z. Xu, Q. Zhang, Z. Zhao, H. Zhang, W. Zhao, Z. Qiu, C. Qi, H. Zhang, H. H. Y. Sung, I. D. Williams, J. W. Y. Lam, Z. Zhao, A. Qin, D. Ma and B. Z. Tang, Adv. Funct. Mater., 2018, 28, 1800051 CrossRef.
- Z. Zhao, C. Chen, W. Wu, F. Wang, L. Du, X. Zhang, Y. Xiong, X. He, Y. Cai, R. T. K. Kwok, J. W. Y. Lam, X. Gao, P. Sun, D. L. Phillips, D. Ding and B. Z. Tang, Nat. Commun., 2019, 10, 768 CrossRef CAS PubMed.
- C. Y. Y. Yu, W. Zhang, R. T. K. Kwok, C. W. T. Leung, J. W. Y. Lam and B. Z. Tang, J. Mater. Chem. B, 2016, 4, 2614–2619 RSC.
- Y. Tan, K. Xu, L. Li, C. Liu, C. Song and P. Wang, ACS Appl. Mater. Interfaces, 2009, 1, 956–959 CrossRef CAS PubMed.
-
X. Xu, R. G. F. Visser and L. M. Trindade, in Starch Polymers, ed. P. J. Halley and L. Avérous, Elsevier, Amsterdam, 2014, pp. 79–102 Search PubMed.
- J. Yang Zhao, L. Chen, J. Li, H.-H. Chen, X. Zhang and H. Zhou, IOP Conf. Ser.: Mater. Sci. Eng., 2020, 461, 012092 CrossRef.
- H. H. Mai, T. T. Nguyen, K. M. Giang, X. T. Do, T. T. Nguyen, H. C. Hoang and V. D. Ta, Soft Matter, 2020, 16, 9069–9073 RSC.
- X. Liu, S. T. Ha, Q. Zhang, M. de la Mata, C. Magen, J. Arbiol, T. C. Sum and Q. Xiong, ACS Nano, 2015, 9, 687–695 CrossRef CAS PubMed.
- V. D. Ta, R. Chen and H. D. Sun, Adv. Mater., 2012, 24, OP60–OP64 CrossRef CAS PubMed.
- Y. Fan, C. Zhang, Y. Du, C. Qiao, K. Wang, Y. Hou, J. Yao and Y. S. Zhao, Adv. Funct. Mater., 2021, 2103031 CrossRef CAS.
- D. Nilsson, S. Balslev, M. M. Gregersen and A. Kristensen, Appl. Opt., 2005, 44, 4965–4971 CrossRef CAS PubMed.
- I. Capron, P. Robert, P. Colonna, M. Brogly and V. Planchot, Carbohydr. Polym., 2007, 68, 249–259 CrossRef CAS.
- Q. Feng, Y. Li, L. Wang, C. Li, J. Wang, Y. Liu, K. Li and H. Hou, Chem. Commun., 2016, 52, 3123–3126 RSC.
- Y. Zhao and D. G. Truhlar, Theor. Chem. Acc., 2008, 120, 215–241 Search PubMed.
- Y. Nishiyama, J.-l. Putaux, N. Montesanti, J.-L. Hazemann and C. Rochas, Biomacromolecules, 2010, 11, 76–87 CrossRef CAS PubMed.
Footnote |
† Electronic supplementary information (ESI) available. See DOI: 10.1039/d1tc00604e |
|
This journal is © The Royal Society of Chemistry 2021 |