Highly pure pentacene crystals grown by physical vapor transport: the critical role of the carrier gas†
Received
3rd October 2020
, Accepted 30th November 2020
First published on 6th January 2021
Abstract
The impurities in commercial pentacene products prohibit systematic and accurate studies on pentacene. We report that highly pure pentacene crystals can be obtained in high yield by using a physical vapor transport process with hydrogen (H2) carrier gas. While other popular carrier gases including argon and nitrogen gas result in dihydropentacene–pentacene cocrystals as a major product, only H2 carrier gas results in pure pentacene crystals. This happens by effectively suppressing the disproportionation reaction of pentacene, which largely owes to its reaction with dihydropentacene, a popular impurity in pentacene. The efficient formation of highly pure pentacene crystals directly from commercial raw precursors will contribute to systematic studies on pentacene as well as development of novel strategies for the growth of high purity organic semiconducting materials.
1. Introduction
The development of organic semiconductors for fundamental research and successful applications requires high purity target organic molecules.1–7 Unfortunately, many potential organic semiconductor molecules, including the commercial products labelled with high purity numbers, suffer from unavoidable impurity species.8–10 Pentacene is one of the most popular organic semiconductor materials, which has been widely studied as a p-type organic semiconductor.8,11–16 Researches based on pentacene have also been suffering from the presence of impurities. Moreover, it is difficult to find the optimal electrical and optical properties of pentacene from the literature due to unavoidable impurities present in most commercially available pentacene chemicals.5,13–15 For the last few decades, there have been many efforts to remove pentacene impurities, which focused either on the conversion of pentacene derivative impurities back to pentacene17 or the removal of impurities.8,13,14 For example, in 2004, the successful removal of pentacenequinone (PQ) from commercial pentacene was demonstrated by using a vacuum sublimation process.14 In 2005 and 2007, Roberson et al. proposed and Northrop et al. identified a pentacene disproportionation reaction, which results in the loss of pentacene and the production of other important impurities, such as dihydropentacene (DHP) and peripentacene (PP) during the physical vapor transport (PVT) process.8,18 While PQ can be removed by vacuum sublimation, and PP is less notorious, thanks to its low vapor pressure, DHP is the last obstacle impurity to tackle. Thus, the exclusion of DHP from pentacene disproportionation reaction could be the golden key to achieve high purity pentacene.
The PVT process is a powerful and popular method for both purification and crystallization of organic molecules either under vacuum or with the flow of carrier gas, depending on the purpose.9,19–24 One important condition that is required while using a carrier gas is that no chemical reaction should occur between the target molecule and the carrier gas, or among the target molecules during the process, especially upon heating to evaporate target molecules, which explains the popular usage of non-reactive noble gases for this purpose. However, many organic molecules undergo undesired reactions not with the carrier gas, but amongst themselves including impurities that are originally present in target molecules. Not only does this occurrence disturb the purification of target molecules but also produces undesired side products. Especially in the case of pentacene, Roberson et al. and Northrop et al. revealed that a rapid disproportionation reaction of pentacene is initiated by catalyzed DHP when starting materials are vaporized during the PVT process.8,18 To obtain high purity pentacene, the pentacene disproportionation reaction needs to be prohibited. For this, we focused on the reduction of collisions between vaporized pentacene (pentacenevapor) and vaporized DHP present in commercially available pentacene (DHPvapor) by which the population of pentacene decreases while more DHPs are newly produced during the PVT process (DHPPVT) that can further react with pentacenevapor (Scheme 1). Therefore, our strategy is to block the undesired disproportionation reaction by providing a PVT environment that allows a low chance for pentacenevapor and DHPvapor to collide with each other. It can be achieved by employing carrier gases with highly mobile and less convective thermofluid motion like hydrogen (H2) and helium (He) carrier gases rather than carrier gases inducing less mobile and high convective thermofluid motion like argon (Ar) and nitrogen (N2) carrier gases. Although there are several previous works reporting no significant influence of carrier gases,8,14 the results are not conclusive because most of them used mixed carrier gases in different ratios, rather than a single type of carrier gas.
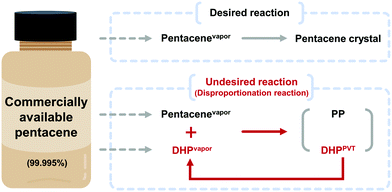 |
| Scheme 1 Schematic of what happens from commercial pentacene powder during PVT process. Undesired disproportionation reaction occurs between vaporized pentacene (pentacenevapor) and vaporized original DHP (DHPvapor) upon heating. Through the reaction, other DHPPVTs are continuously generated while pure pentacene is continuously removed. | |
Herein, we report the clear and obvious difference between H2 and Ar carrier gas on purifying pentacene by the PVT method. We focused on the suppression of the disproportionation reaction of pentacene, mainly caused by the reaction between pentacenevapor and DHPvapor in the vapor phase, which is the key source of continuous generation of impurities in pentacene. For this, we were concerned with the mobility effect of carrier gas, as the mobility of carrier gas affects the probability of the disproportionation reaction. As a carrier gas, Ar, H2, N2, and He gases were examined. Indeed, it reveals that H2 and He efficiently suppress the disproportionation reaction of pentacene and produce highly pure pentacene crystals (pentaceneH2). On the contrary, the same treatments using Ar or N2 carrier gas result in impurities containing DHP–pentacene cocrystals (pentaceneAr) as a major product.
2. Results and discussion
Analysis of commercial pentacene powder
Before designing the experiments, the original impurities in the commercial pentacene powder (Sigma-Aldrich, 99.995%) used in this study were analyzed by electron ionization mass spectrometry (EI-MS) and laser desorption/ionization mass spectrometry (LDI-MS). The mass spectrum of the commercial pentacene shows peaks with m/z values at 278, 279, and 280, which correspond to pentacene and impurities of 6-pentacenyl radical (6PR), and DHP, respectively (Fig. S1 and S2, ESI†). The calculated total impurity ratio (DHP and 6PR) based on the mass data is 1.33%. As the unstable radical form, 6PR is considered as the H+ eliminated form of DHP during the laser ionization process;18 it is confirmed that the major impurity of the commercial pentacene is DHP. In addition, when pentacene powder is heated for 60 minutes below its sublimation temperature in an inert environment, the peak intensities of DHP and 6PR increase in the mass spectrum (Fig. S3, ESI†), which implies that the thermal treatment generates a large amount of DHP and prevents the formation of high purity pentacene films or crystals. To find a way to exclude DHP during pentacene crystallization by PVT, we performed systematic studies on various carrier gases that have distinct physical properties.
PVT process with Ar carrier gas
We conducted pentacene recrystallization first using Ar gas. The PVT experimental setup is shown in Scheme 2 and the detailed experimental method is described in the supplementary information. On conducting the PVT process at 623 K for 13 minutes, wire crystals were obtained as a major product with minor disk crystals on the SiO2/Si substrate (Fig. 1a and b). The MS results of the produced crystals showed remarkably increased intensities of 6PR and DHP at m/z of 279 and 280, respectively (Fig. 1c), compared to the original pentacene powder (Fig. S2, ESI†). This result implies that the recrystallization of pentacene by PVT under Ar condition involves the drastic formation of DHP. Single-crystal X-ray diffraction (SXRD) analysis also reveals that the wire crystal has the monoclinic (P21/n) space group, forming a herringbone packing structure with the mixture of DHP and pentacene. The asymmetric unit is built from non-planar DHP and planar pentacene molecules at a 2
:
1 ratio, which agrees with the previously reported DHP–pentacene cocrystal (Fig. 1d and e).12 This major DHP–pentacene cocrystal is denoted as pentaceneAr. The large amount of DHP in the products implies the further generation of DHP (DHPPVT) by the continuous reaction between pentacenevapor and DHPvapor, which results in the formation of pentaceneAr, rather than pure pentacene crystals.
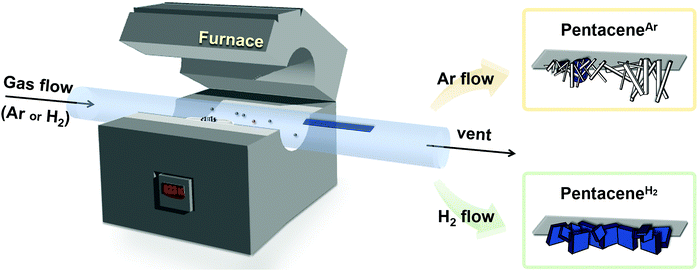 |
| Scheme 2 Schematic illustration of the PVT process and obtained crystals. | |
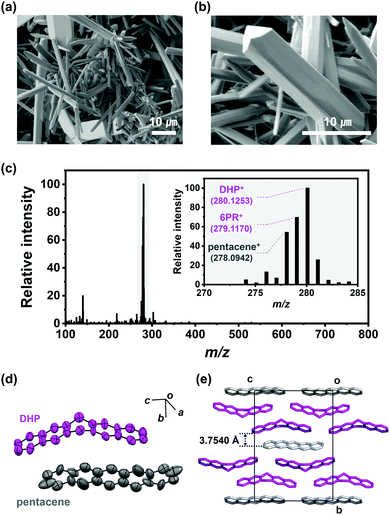 |
| Fig. 1 Characterization of pentaceneAr. (a and b) SEM images of pentaceneAr at (a) low-magnification and (b) high-magnification. (c) Full scan mass spectrum and the corresponding inset zoom scan of pentaceneAr, showing drastic increase in impurities (6PR and DHP). (d) The ORTEP view of the asymmetric unit with 50% thermal ellipsoids consisting of pentacene (grey) and DHP (magenta) and (e) their herringbone packing structure viewed along the a-axis. H atoms are omitted for clarity. | |
PVT process with H2 carrier gas
Contrary to Ar carrier gas, only rectangular-shaped crystals (pentaceneH2) were obtained uniformly under H2 gas at flow rates higher than 200 sccm (Fig. 2a and b). The pentaceneH2 turned out to be composed only of pentacene according to the MS data (Fig. 2c). The peaks of m/z = 279 and 280 correspond to the isotopes of pentacene according to the isotope calculation results (Fig. S4, ESI†). From the SXRD results, pentaceneH2 has a triclinic (P
) space group having C–H/π interactions between adjacent pentacene molecules with a distance of 2.873 Å, and a closely packed herringbone stacking structure was confirmed (Fig. 2d and e). This agrees well with the previously reported pentacene crystal structure.25,26
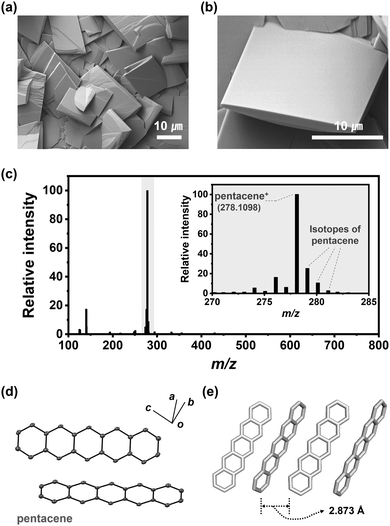 |
| Fig. 2 Characterization of pentaceneH2. (a and b) SEM images of pentaceneH2 at (a) low-magnification and (b) high-magnification. (c) Full scan mass spectrum and the corresponding inset zoom scan of pentaceneH2, showing only isotopic pattern of pentacene at m/z 278. (d) The ORTEP view of the asymmetric unit with 50% thermal ellipsoids and (e) their packing structure along the a-axis. It interacts with head-to-head pentacene molecules with the interplanar distance of 2.873 Å. | |
It is also verified that H2 does not react with pentacene during the PVT process, as no H radical species dissociated from H2 that would react with DHP. This was also confirmed from deuterium gas control experiments.
Physical behavior of carrier gases during the PVT process
During the PVT process, carrier gases are known to undergo various fluidic motions in a quartz tube. Different thermofluid motion characteristics derived from different physical properties of carrier gases affect the chemical and physical phenomena during the PVT process. The molecular weight of the gas is highly related to the mobility and flow pattern that affect vapor phase reactions. Mobility is determined by the average velocity of the gas before and after collisions between molecules. The root mean square velocity of H2 (2776 m s−1) is about 4.5 times higher than that of Ar (623.7 m s−1) at 623 K (Fig. S5, ESI†). The fast-moving H2 molecules reduce the collision between pentacenevapor and DHPvapor, which enables efficient suppression of the disproportionation reaction. Besides the mobility effect of the carrier gas, its thermofluid motion, either in a convection mode or laminar flow mode, also affects the collision of the vaporized molecules. From a macroscopic view, the flow type, especially the degree of thermal convection, also affects the chemical reactions in the PVT. Rayleigh number, determined by the viscosity of the carrier gas, provides transport information in accordance with the degree of buoyancy-driven convective flow during the PVT process by means of the temperature gradient and flowing carrier gas molecules.21,27–29 It is calculated that H2 with its low viscosity has a Rayleigh number about 50 times smaller than Ar (Fig. S6, ESI†). This implies that H2 gives laminar flow driven by minimized convection. In a less convective environment, less chance is given to the vaporized molecules to meet each other by transporting them quickly to the crystallization zone via laminar flow (Fig. 3a, bottom). Thus, it suppresses the formation of DHPPVT and results in pure pentacene crystals. In the case of the Ar carrier gas, on the other hand, the relatively high degree of convection provides a greater chance of collision between pentacenevapor and DHPvapor as convection helps vaporized molecules to stay together longer (Fig. 3a, top), by which the disproportionation reaction is promoted, resulting in a large amount of pentaceneAr.
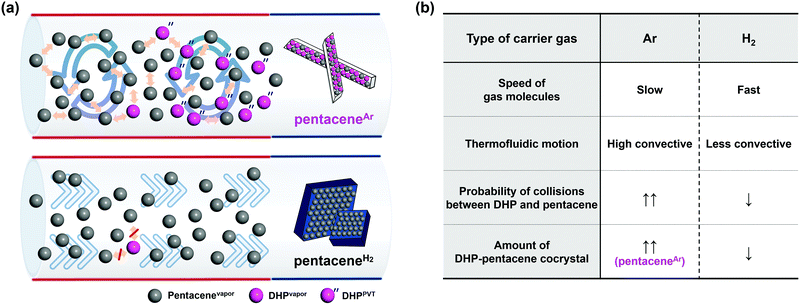 |
| Fig. 3 Schematics and summary of the proposed mechanism under Ar and H2 flow. (a) Proposed mechanism of carrier gas effect in Ar (top) and H2 environments (bottom). Ar effect gives a high convection environment and enough chances for the disproportionation reaction between pentacenevapor (grey) and DHPvapor (magenta) molecules. It brings about the formation of a large amount of DHPPVT (magenta with double quotation mark (″)) and pentaceneAr. On the other hand, H2 effect in pentacene recrystallization effectively suppresses the disproportionation reaction and facilitates pure pentacene crystallization (pentaceneH2). (b) Phenomena and results occurring in the quartz tube under each gas flow during PVT process. | |
The properties and effects of carrier gases are summarized in Fig. 3b. The remaining material in the crucible after the reaction was weighed and analyzed by mass spectrometry to confirm the composition and amount of residual impurities. As a result, it is confirmed that the remaining material in a crucible is a mixture of DHP, 6PR, and PP with unreacted pentacene. While a significantly large amount of impurities was observed in an Ar environment, it was rarely observed in an H2 environment. The detailed percentage change of impurities before and after the reaction is calculated (Fig. S7, ESI†). Furthermore, the large amount of PP in the Ar condition indicates the high degree of disproportionation reaction in the Ar carrier gas environment (Fig. S8, ESI†).
Confirmation of the carrier gas effect on the PVT process
In addition to Ar and H2, the same PVT processes were conducted using N2 and He carrier gases to confirm the carrier gas effect. When N2 was used for comparison with Ar, which has a relatively higher molecular weight, a considerable amount of wire-shaped DHP–pentacene cocrystals were produced. The SXRD and MS data indicate that these crystals are identical to pentaceneAr (Fig. S9, ESI†). Like Ar, the low mobility of N2 increases the chance for the disproportionation reaction of pentacene. On the other hand, similar to H2, pure pentacene crystals were mainly obtained when He was used (Fig. S10, ESI†). These results prove that the physical properties of the carrier gas highly affect the reaction of pentacene and DHP impurity, and eventually determine the purity of pentacene crystals obtained through the PVT process.
Photoluminescence (PL) properties of pentaceneAr and pentaceneH2
Although there have been many studies on the PL properties of pentacene, their authenticity is still under debate due to the presence of unavoidable impurities both in the crystal phase and in the solution phase.13,30–33 However, the successful formation of highly pure pentaceneH2 now allows us to clarify the PL property of pentacene. The PL spectra and images of pentaceneAr and pentaceneH2 were measured at 298 K (Fig. 4). In contrast to the intense pink PL of pentaceneAr with high luminescent singlet excitons at 600 nm, pentaceneH2 showed no PL. Furthermore, the PL properties of each crystal in anhydrous cyclohexane were confirmed to be absent (Fig. S11, ESI†). While pentaceneAr solution is still emissive, pentaceneH2 solution is non-emissive. The absence of emission corresponds to the singlet fission between adjacent molecules. Through the singlet fission phenomenon, pentaceneH2 has a high decay rate of singlet excitons into two triplet ones.34–37 From these results, it is clearly revealed that highly pure pentacene does not exhibit PL at room temperature (298 K). When the PL property of pentaceneH2 was measured at 194 K, its PL intensity was still low, but several clear peaks in the range of 620 nm to 880 nm were observed (Fig. S12, ESI†). The dominant PL peaks of pentaceneH2 observed at 696 and 719/733 nm matched well with the results from pentacene single crystal measured at 10 K, and each peak corresponds to singlet (S1) to singlet transition (S0) and recombination of self-trapped excitons, respectively.38 Combined with the SXRD and PL analysis results, we conclude that highly intensive emission of pentacene films or crystals at room temperature, if reported, could have originated from impurities like DHP–pentacene cocrystal.30,33
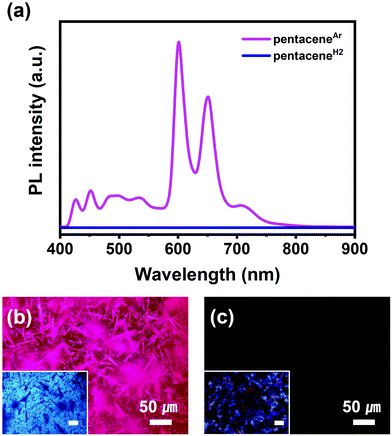 |
| Fig. 4 PL measurement of pentaceneAr and pentaceneH2. (a) PL spectra of pentaceneAr (magenta) and pentaceneH2 (blue) measured at 298 K. (b and c) PL images of (b) pentaceneAr and (c) pentaceneH2 (inset images in (b) and (c) are optical microscopy (OM) images of pentaceneAr and pentaceneH2, respectively. The scale bar of insets is 50 µm). | |
Electrical properties of pentaceneAr and pentaceneH2
The electrical properties of each crystal were examined from field-effect transistor (FET)-type electronic devices (Fig. S13, ESI†). The thin crystal was successfully obtained by modifying the flow rate of the carrier gas from 200 sccm to 800 sccm (Fig. S14, ESI†). Single crystals of pentaceneAr and pentaceneH2 were directly transferred onto SiO2/Si substrates containing pre-patterned Au source and drain electrodes. PentaceneAr shows an insulating property with resistivity higher than 106 Ω cm (Fig. 5a), which is ascribed to the low degree of π-electron delocalization of DHP. On the other hand, highly pure pentaceneH2 shows a clear p-type semiconducting behavior with an average hole mobility of 2.48 cm2 V−1 s−1, which is comparable to previous studies (Fig. 5b and c).8,39–41 These results imply that the growth of highly pure pentacene crystals is essential to elucidate their intrinsic optical and electrical properties.
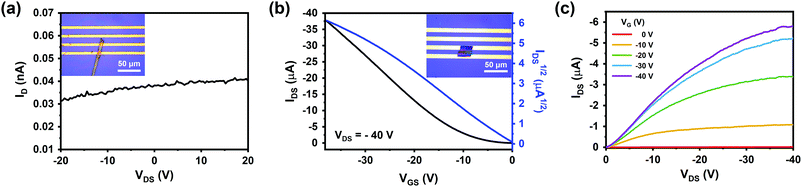 |
| Fig. 5 Electrical properties of pentaceneAr and pentaceneH2. (a) I–V curve of insulating pentaceneAr. (b and c) (b) Transfer and (c) output curves of pentaceneH2 indicating p-type semiconducting behavior, respectively. Inset images in (a) and (b) are OM images of pentaceneAr and pentaceneH2 onto the substrate pre-patterned with Au contacts. | |
Versatility test
To examine the versatility of PVT using H2 gas for the formation of highly pure pentacene crystals, we examined the above-mentioned processes using another pentacene source (TCI, P0030), and the same carrier gas effect was confirmed (Fig. S15–S17, ESI†). In addition, to confirm the carrier gas effect of PVT at low-pressure, we also conducted the same processes at low pressure (>101 torr). The result was same as the case at atmospheric pressure (Fig. S18, ESI†).
3. Conclusions
With the goal of obtaining high purity pentacene crystals, we studied the effect of carrier gases during the PVT process on the formation of pentacene crystals and revealed that pure pentacene crystals were obtained using H2 carrier gas through the efficient suppression of disproportionation reaction. This is believed to be due to the high mobility and minimized degree of convection of H2, by which the collisions between pentacene and DHP are significantly suppressed. Control studies using four different carrier gases (Ar, N2, H2, and He) proved that H2 and He resulted in highly pure pentacene crystals, while Ar and N2 resulted in DHP–pentacene cocrystals. In addition, we confirmed high PL with insulating properties for pentaceneAr, while no PL with good p-type semiconducting properties for pentaceneH2.
4. Methods
Physical vapor transport (PVT) process
The PVT process was performed using various carrier gases (Ar, H2, N2, and He). 30 mg of pentacene powder purchased from Sigma-Aldrich or TCI (Japan) was placed at the center of a horizontal tube-type furnace. A piece of SiO2/Si substrate was attached in a quartz protection tube at the end of the heating zone to collect the resulting crystals efficiently. The vaporization temperature was 623 K after flushing the quartz tube using each carrier gas. The growth time was 13 min after the furnace reached 623 K (flow rate of all carrier gases = 200 sccm (fixed)). The furnace lid was opened to cool down after 13 min, wire-shaped pentaceneAr and plate-shaped pentaceneH2 were obtained when Ar (or N2), and H2 (or He) were employed, respectively.
Characterization
For structural analysis, single-crystal X-ray diffraction (SXRD) and powder X-ray diffraction (PXRD) patterns were obtained from the 2D and 5D beamlines at the Pohang Accelerator Laboratory (PAL). We note that all the two theta values are converted with the conventional wavelength (CuKα = 1.541841 Å) for a better comparison with references. The morphologies of each crystal were observed using a scanning electron microscope (SEM, Hitachi, S-3400N) and their chemical compositions were characterized by electron ionization (EI-MS, Varian, 320MS), high-resolution mass spectrometry (HR-MS, JEOL, JMS-700) and laser desorption/ionization mass spectrometry (LDI-MS, AB Sciex Pte. Ltd, 4700 Proteomics Analyzer) without a matrix additive in matrix-assisted LDI-MS. Also, PL spectra and images were obtained using a fluorescence microscope combined with a spectrometer equipped with a filter set (exciter BP 330–380 nm; beam splitter 400 nm; emitter LP 410 nm, Semrock). Electrical measurements were carried out using a vacuum chamber probe station (MS TECH, M6VC) and a semiconductor analyzer (Keithley, 4200A-SCS). The temperature during the measurement was controlled by a hot chuck controller (MS TECH, MST-1000H) equipped in the probe station.
Single crystal X-ray diffraction structural characterization
All pentacene crystals (pentaceneH2) and DHP–pentacene cocrystals (pentaceneAr) were coated with paratone-N oil and the diffraction data were measured at 100 K with asynchrotron radiation (λ = 0.90000 Å) using a Rayonix MX225HS CCD detector at the BL2D SMC beamline (PAL, Republic of Korea) equipped with a silicon (111) double crystal monochromator. The PAL BL2D-SMDC42 program was used for data collection (sample to detector distance of 66 mm, ω scan; ω = 1°, exposure time of 1 s per frame for pentaceneH2 and ω = 2°, exposure time of 3 s per frame for pentaceneAr) and HKL3000sm (version 716.7) was used for cell refinement, reduction and absorption correction.43,44 The crystal structures were solved by the intrinsic phasing method with SHELXT,45 and refined by full-matrix least squares refinement using the SHELXL-2018/3 program.46 The positions of all non-hydrogen atoms were refined with anisotropic displacement factors. Non-water hydrogen atoms were placed using a riding model and their positions were constrained relative to their parent atoms using the appropriate HFIX command in SHELXL-2018/3.
(1) PentaceneH2.
C22H14, Mr = 278.33, crystal dimensions 0.085 × 0.058 × 0.001 mm3, triclinic, P
, a = 6.2410(12) Å, b = 7.6680(15) Å, c = 14.407(3) Å, α = 76.99(3) °, β = 87.99(3) °, γ = 84.55(3) °, V = 668.7(2) Å3, T = −173 °C, Z = 2, rcal = 1.382 g cm−3, µ = 0.13 mm−1, 1485 unique reflections out of 2432 with I > 2s(I), 199 parameters, 1.837° < q < 29.890°, R1 = 0.0543, wR2 = 0.1338, GOF = 1.115. CCDC deposit number 170187.
(2) PentaceneAr.
C66H46, Mr = 893.03, crystal dimensions 0.151 × 0.009 × 0.009 mm3, monoclinic, P21/n, a = 6.1460(12) Å, b = 21.636(4) Å, c = 15.976(3) Å, β = 93.31(3) °, V = 2120.9(7) Å3, T = −173 °C, Z = 2, rcal = 1.314 g cm−3, µ = 0.12 mm−1, 1630 unique reflections out of 2776 with I > 2s(I), 281 parameters, 2.009° < q < 28.334°, R1 = 0.0917, wR2 = 0.2221, GOF = 0.986. CCDC deposit number 200763.
Conflicts of interest
There are no conflicts to declare.
Acknowledgements
This work was supported by the Veteran Researcher Grant (No. 2019R1A2C2004259) funded by the National Research Foundation of Korea (NRF) and Samsung Electronics. Powder and single-crystal X-ray diffraction measurements were conducted at 5D and 2D beamlines of the Pohang Accelerator Laboratory (PAL, South Korea).
References
- A. Facchetti, Mater. Today, 2007, 10, 28–37 CrossRef CAS.
- S. E. Root, S. Savagatrup, A. D. Printz, D. Rodriquez and D. J. Lipomi, Chem. Rev., 2017, 117, 6467–6499 CrossRef CAS.
- Y. Wang, L. Sun, C. Wang, F. Yang, X. Ren, X. Zhang, H. Dong and W. Hu, Chem. Soc. Rev., 2019, 48, 1492–1530 RSC.
- C. Wang, H. Dong, L. Jiang and W. Hu, Chem. Soc. Rev., 2018, 47, 422–500 RSC.
- H. F. Haneef, A. M. Zeidell and O. D. Jurchescu, J. Mater. Chem. C, 2020, 8, 759–787 RSC.
- J. Pflaum, J. Niemax and A. K. Tripathi, Chem. Phys., 2006, 325, 152–159 CrossRef CAS.
- H. Jiang and W. Hu, Angew. Chem., Int. Ed., 2020, 59, 1408–1428 CrossRef CAS.
- L. B. Roberson, J. Kowalik, L. M. Tolbert, C. Kloc, R. Zeis, X. Chi, R. Fleming and C. Wilkins, J. Am. Chem. Soc., 2005, 127, 3069–3075 CrossRef CAS.
- R. Zeis, C. Besnard, T. Siegrist, C. Schlockermann, X. Chi and C. Kloc, Chem. Mater., 2006, 18, 244–248 CrossRef CAS.
- C. Chen, Z. Chi, K. C. Chong, A. S. Batsanov, Z. Yang, Z. Mao, Z. Yang and B. Liu, Nat. Mater., 2020 DOI:10.1038/s41563-020-0797-2.
- C. D. Dimitrakopoulos, A. R. Brown and A. Pomp, J. Appl. Phys., 1996, 80, 2501–2508 CrossRef CAS.
- C. C. Mattheus, J. Baas, A. Meetsma, J. L. de Boer, C. Kloc, T. Siegrist and T. T. M. Palstra, Acta Crystallogr., Sect. E: Struct. Rep. Online, 2002, 58, o1229–o1231 CrossRef CAS.
- R. He, X. Chi, A. Pinczuk, D. V. Lang and A. P. Ramirez, Appl. Phys. Lett., 2005, 87, 211117 CrossRef.
- O. D. Jurchescu, J. Baas and T. T. M. Palstra, Appl. Phys. Lett., 2004, 84, 3061–3063 CrossRef CAS.
- J. E. Northrup and M. L. Chabinyc, Phys. Rev. B: Condens. Matter Mater. Phys., 2003, 68, 041202 CrossRef.
- S.-Z. Weng, W.-S. Hu, C.-H. Kuo, Y.-T. Tao, L.-J. Fan and Y.-W. Yang, Appl. Phys. Lett., 2006, 89, 172103 CrossRef.
- C. Pramanik and G. P. Miller, Molecules, 2012, 17, 4625–4633 CrossRef CAS.
- B. H. Northrop, J. E. Norton and K. N. Houk, J. Am. Chem. Soc., 2007, 129, 6536–6546 CrossRef CAS.
- C. Reese and Z. Bao, Mater. Today, 2007, 10, 20–27 CrossRef CAS.
- X. Xue, Z. Zhou, B. Peng, M. M. Zhu, Y. J. Zhang, W. Ren, Z. G. Ye, X. Chen and M. Liu, RSC Adv., 2015, 5, 79249–79263 RSC.
- R. A. Laudise, C. Kloc, P. G. Simpkins and T. Siegrist, J. Cryst. Growth, 1998, 187, 449–454 CrossRef CAS.
- J. S. Bangsund, T. R. Fielitz, T. J. Steiner, K. Shi, J. R. Van Sambeek, C. P. Clark and R. J. Holmes, Nat. Mater., 2019, 18, 725–731 CrossRef CAS.
- H. Wang, Y. Zhao, Z. Xie, H. Wang, B. Wang and Y. Ma, CrystEngComm, 2014, 16, 4539–4545 RSC.
- A. R. Ullah, A. P. Micolich, J. W. Cochrane and A. R. Hamilton, Proc. SPIE, 2007, 6800, 680005 CrossRef.
- R. B. Campbell, J. M. Robertson and J. Trotter, Acta Crystallogr., 1961, 14, 705–711 CrossRef CAS.
- C. C. Mattheus, A. B. Dros, J. Baas, G. T. Oostergetel, A. Meetsma, J. L. D. Boer and T. T. M. Palstra, Synth. Met., 2003, 138, 475–481 CrossRef CAS.
-
R. B. Bird, W. E. Stewart and E. N. Lightfoot, Transport Phenomena, John Wiley and Sons, NY, 2nd edn, 2002 Search PubMed.
- S. Musso, S. Porro, M. Rovere, M. Giorcelli and A. Tagliaferro, J. Cryst. Growth, 2008, 310, 477–483 CrossRef CAS.
-
D. M. McEligot, D. A. Denbow and H. D. Murphy, Transient natural convection in heated inclined tubes, Los Alamos, NM, 1990 Search PubMed.
- A. Sharma, A. Khan, Y. Zhu, R. Halbich, W. Ma, Y. Tang, B. Wang and Y. Lu, Nano Lett., 2019, 19, 7877–7886 CrossRef CAS.
- Yu. P. Piryatinski and M. V. Kurik, Ukr. J. Phys., 2011, 56, 1048–1055 CAS.
- Yu. P. Piryatinski, M. Furier and V. Nazarenko, Semicond. Phys., Quantum Electron. Optoelectron., 2001, 4, 142–145 CrossRef CAS.
- J. E. Park, M. Son, M. Hong, G. Lee and H. C. Choi, Angew. Chem., Int. Ed., 2012, 51, 6383–6388 CrossRef CAS.
- A. A. Bakulin, S. E. Morgan, T. B. Kehoe, M. W. B. Wilson, A. W. Chin, D. Zigmantas, D. Egorova and A. Rao, Nat. Chem., 2016, 8, 16–23 CrossRef CAS.
- M. W. B. Wilson, A. Rao, B. Ehrler and R. H. Friend, Acc. Chem. Res., 2013, 46, 1330–1338 CrossRef CAS.
- P. M. Zimmerman, F. Bell, D. Casanova and M. Head-Gordon, J. Am. Chem. Soc., 2011, 133, 19944–19952 CrossRef CAS.
- J. R. Tritsch, W.-L. Chan, X. Wu, N. R. Monahan and X.-Y. Zhu, Nat. Commun., 2013, 4, 2679 CrossRef.
- T. Aoki-matsumoto, K. Furuta, T. Yamada, H. Moriya, K. Mizuno and A. H. Matsui, Int. J. Mod. Phys. B, 2001, 15, 3753–3756 CrossRef CAS.
- J. Dong, P. Yu, S. A. Arabi, J. Wang, J. He and C. Jiang, Nanotechnology, 2016, 27, 275202 CrossRef.
- J. Y. Lee, S. Roth and Y. W. Park, Appl. Phys. Lett., 2006, 88, 252106 CrossRef.
- S. Lee, S.-J. Kang, G. Jo, M. Choe, W. Park, J. Yoon, T. Kwon, Y. Ho Kahng, D.-Y. Kim, B. Hun Lee and T. Lee, Appl. Phys. Lett., 2011, 99, 083306 CrossRef.
- J. W. Shin, K. Eom and D. Moon, J. Synchrotron Radiat., 2016, 23, 369–373 CrossRef.
- Z. Otwinowski, D. Borek, W. Majewski and W. Minor, Acta Crystallogr., Sect. A: Found. Crystallogr., 2003, 59, 228–234 CrossRef.
- Z. Otwinowski and W. Minor, Methods Enzymol., 1997, 276, 307–326 CAS.
- G. M. Sheldrick, Acta Crystallogr., Sect. A: Found. Adv., 2015, 71, 3–8 CrossRef.
- G. M. Sheldrick, Acta Crystallogr., Sect. C: Struct. Chem., 2015, 71, 3–8 Search PubMed.
Footnote |
† Electronic supplementary information (ESI) available. See DOI: 10.1039/d0tc04698a |
|
This journal is © The Royal Society of Chemistry 2021 |
Click here to see how this site uses Cookies. View our privacy policy here.