Precision tuning of rare-earth-doped upconversion nanoparticles via droplet-based microfluidic screening†
Received
9th September 2020
, Accepted 10th November 2020
First published on 19th November 2020
Abstract
Rare-earth-doped upconversion nanoparticles (UCNPs) show great promise in a range of applications, including biological imaging and sensing, solar cells and security inks. Although their emission color can be tuned widely as a function of host matrix and dopant composition, the high dimensionality of the associated parameter space and the sensitivity of emission to these parameters make optimization and precision tuning difficult. Herein, we present a new time-efficient synthetic route to NaYF4:Yb,Er,Tm UCNPs and a high-throughput microfluidic reactor to synthesize and precisely tune the emission characteristics of the particles in situ and in real time. We synthesize a range of particles with optimized emission intensity and wide color distribution by changing the doping degree of sensitizer Yb to provide for green-orange tunability, and the ratio of Tm–Er to give green–blue tunability. With the two tunable dimensions, we realize true white light emitting UCNPs based on optimized red, green and blue (RGB) emission ratios from a single composition NaYF4:Yb,Er,Tm nanocrystal—a demanding task for such materials—with CIE 1931 coordinates of (0.29, 0.34) and doping degrees of 60% Yb, 0.45% Er, and 1.05% Tm. Finally, we demonstrate the efficacy of these materials in a thin film format through the fabrication of an anti-counterfeit device.
1 Introduction
White light-emitting materials have attracted enormous attention for the development of energy efficient and high performance lighting and displays.1–3 Materials of interest include polymers, small molecules, quantum dots and rare-earth-doped upconversion nanoparticles (UCNPs).4–8 Whilst white light emission is relatively easy to achieve by mixing different colors of emitter in a heterogeneous system, such an approach suffers from problems associated with phase separation of the different emitting bodies, unwanted energy transfer, low stability and/or the need for multiple excitation wavelengths.9,10 Accordingly, new materials that can emit red-green-blue (RGB) colors from a single entity are highly sought after.
Rare-earth (RE) UCNPs, typically co-doped with sensitizer ions of ytterbium (Yb3+) and activator ions of X3+ (X = Er, Tm, and/or Ho) in the same nanocrystal host, can yield non-linear anti-Stokes luminescence via sequential absorption of two or more low energy near-infrared (NIR) photons.11,12 UCNPs are free of reabsorption, emit with narrow peaks and are photostable, with tunable lifetimes and low toxicity.13,14 Long-wavelength NIR excitation leads to less scatter, minimizes photodamage and autofluorescence of biological samples and allows deep tissue penetration.15 Standout applications of UCNPs include biological imaging and therapy,16 photonic devices,17 luminescence thermometry,18 biosensing,19 and anti-counterfeiting.20–22 Regarding white light emission, it is possible to tune rare-earth UCNPs to act as single entity white light emitters, overcoming the previously noted problems encountered in heterogeneous populations. For example, Sivakumar et al. demonstrated a white light thin film made from RE3+-doped LaF3 nanoparticles, which could be excited using a single NIR source.4 Subsequently, Wang et al. reported white upconversion rare-earth oxide materials Tm2O3 and Yb2O3, which achieved pure white light emission using single source excitation.23 More recently, Zhang et al. designed a core–shell–shell nanostructure incorporating several RE3+ within a single nanocrystal, and whose emission colors could be tuned by changing the excitation power density for white light.9 Additionally, Wang and co-workers have reported a Yb3+/Er3+/Tm3+ tridoped hexagonal β-NaYF4 microrod for white-light lasing at room temperature.24 Despite these successes, the precision tuning of emission characteristics across a wide range and using a single nanocrystal composition is still a challenge, and there is much potential still to be unlocked.
During the last decade, several UCNP synthesis methods have been proposed,25 including hydrothermal, thermal decomposition, and thermal co-precipitation reactions.26–28 However, these typically require harsh reaction conditions (i.e. high temperatures and/or high pressures), and extended reaction times. Moreover, bulk reaction approaches to nanocrystal synthesis typically necessitate large investments of time and materials to effectively map an inherently complex reaction parameter space. This is especially problematic for UCNPs, as their properties are extremely sensitive to the exact preparation conditions, in particular the doping degree of the sensitizers and activators, and thus they would benefit hugely from a more comprehensive characterization and optimization process. Further, we propose that the development of UCNPs will drastically benefit from a more efficient, economic and greener strategy for fast parameter screening, with a view to optimizing properties for advanced applications.
Since the pioneering works of nearly two decades ago,29–31 great strides have been made in establishing the field of microfluidic-based nanoparticle synthesis. In the field of rare-earth UCNPs, previous reports have demonstrated the utility of continuous flow microfluidic approaches.32–34 However, such methods are not suitable for high-throughput screening because of difficulties in controlling cross-contamination between solutions run with different reaction parameter sets. In contrast, droplet-based microfluidic reactors have engendered a time-efficient high-throughput screening approach for some years now,35 due to efficient heat and mass transfer within droplets, and the ability to analyze products and tune reactions in real-time.36 However, rare-earth UCNPs have not benefitted from this approach due to their restrictively long reaction times and the difficulty in preparing single compositional element sources (i.e. precursors that deliver only one of the elements that form the final NC). Unlike in common bulk reactions where the RE3+, Na+ and F− are premixed in one solution, in the proposed high-throughput screening method it is imperative that each of the compositional elements can be delivered by a separate syringe to allow fine control of reaction stoichiometry. However, this isolation of precursors causes solubility issues, which necessitates redesigning the reaction in terms of solvent, reagents and ligands.
In this paper, we detail a new synthetic approach for rare-earth UCNPs that allows for facile parametric space mapping and fine tuning of RGB emission under NIR excitation. Focussing on Yb–Er–Tm tri-doped NaYF4 UCNPs, we first develop a novel reaction formulation that enables time-efficient screening, where each compositional element can be delivered in a separate precursor for precise tuning of elemental ratios (in terms of F− and Na+ for the host matrix, Yb3+ as a sensitizer, and Er3+ and Tm3+ as the activators). Second, and to achieve rapid and efficient parameter mapping and product optimization, we show for the first time the use of a high-throughput droplet-based microfluidic reactor with inline emission analysis for rapid lanthanide-doped UCNP synthesis and compositional tuning. This approach was used to scan and tune the RGB emission attainable from a single NaYF4:Yb,Er,Tm nanocrystal composition (as opposed to a mixed population) by optimizing elemental ratios, engendering tunable true white light emission based on optimized RGB intensity ratios under single excitation. These developments encompass a new strategy for rapid UCNP synthesis, characterization and optimization. Expansion of this approach will pave the way for advanced applications of these promising materials.
2 Results and discussion
2.1 Experimental design
We developed a droplet-based microfluidic platform (see Fig. 1a) for reaction parameter screening of NaYF4:Yb,Er,Tm UCNPs using integrated real-time inline infrared laser (980 nm) excitation and spectral reading (see ESI† for full details). Briefly, seven syringes were used to deliver the reaction components, with four syringes filled with Re3+ precursors at equal molar concentrations, two syringes with the Na+ and F− precursors, and a final syringe with the carrier oil. The four Re3+ precursors were initially mixed in a seven-port manifold, before being delivered into a second manifold to be mixed with the Na+ and F− precursors and the oil to segment the flow into droplets. This arrangement allows precise control of the ratios between the RE3+ regents, as well between the Na+ and RE3+, and RE3+ to F−. Formed droplets then travelled through a heating module, where the droplets are conveyed through PTFE tubing around a heated copper rod. The formed UCNPs were monitored in situ with an inline photoluminescence system, requiring a minimum time of 3 minutes to collect data per reaction parameter set.
 |
| Fig. 1 (a) Schematic of the droplet-based microfluidic platform with inline infrared laser (980 nm) PL detection for NaYF4:Yb,Er,Tm UCNP synthesis. The microfluidic platform incorporates syringe pumps, a heating controller, and a real-time fluorescence detection system together. (b) Photograph of the heating loops around a 2 cm diameter heating rod. (c) Temperature distribution of the flow at the beginning of the heater, with temperatures of the heater and surrounding air at 220 °C and 25 °C, respectively. (d) Variation of the temperature of the reaction solution as a function of heating time. | |
The ability to rapidly scan precursor ratios allows for fast, accurate, reproducible and efficient parameter screening.37 In this regard, it should be noted that droplet-based microfluidic systems benefit from rapid heat and mass transfer, which ensures prompt mixing and temperature equilibration on ultra-short timescales.38 To assess the temperature equilibration time in our reactor, we conducted computational fluid dynamic (CFD) simulations of the heat transfer from the heating rod (Fig. 1b) into the reaction solution (Fig. 1c and Fig. S1, ESI†). At a total flow rate 80 μL min−1, the reaction solution reaches the target temperature (i.e. room temperature to 220 °C) after 3 mm of travel, and within 500 ms (Fig. 1d). This significantly improves upon the heat transfer efficiency obtained in flask-based systems, and reduces reaction times for UCNPs synthesis. Further details on the simulations are provided in the ESI.†
To tune or optimize the properties of NaYF4:Yb,Er,Tm UCNPs by reaction parameter screening, the precursor ratios between each constituent element must be controllable, meaning that each element must to be delivered in a separate precursor. This is not typically the case in bulk reactions, where Na+, RE3+, and F− are combined within a single precursor.26–28 In our new strategy, we are able to deliver each element in a separate precursor. Furthermore, instead of using 1-octadecene, we employed TEGDME as the solvent for all precursors due to its high boiling point (275 °C) and excellent solubility for F−, Na+, and RE3+. Finally, it should be noted that modulation of droplet residence times in the heated zone allows for control over the total reaction time.
2.2 Parameter optimization and color tuning for NaYF4:Yb,Er
In NaYF4:Yb,Er nanocrystals, NaYF4 is the host lattice for lanthanide ions, Yb3+ acts as a sensitizer to absorb the near-infrared laser light, whilst Er3+ is the activator for green and red emission. Optimization of emission intensity and tuning of spectral profile (i.e. color) can be achieved by varying the precursor ratios for the host lattice, sensitizer and activators, along with the reaction temperature. Accordingly, we assessed the following ratios in this work: R1 = Na/RE; R2 = F/RE; R3 = Yb/RE and R4 = Er/RE. R1 (Na/RE) and R2 (F/RE), which are the critical ratios for the host lattice NaYF4. While screening these two parameters, we initially set the RE doping to typical values reported in the literature (molar percentages of 80% Y, 18% Yb, and 2% Er).33,39 As shown in Fig. 2a and b, the PL intensity of the formed NaYF4:Yb,Er nanocrystals was relatively weak when R1 was lower than 1.2, but rapidly increased to a maximum at R1 = 1.8.
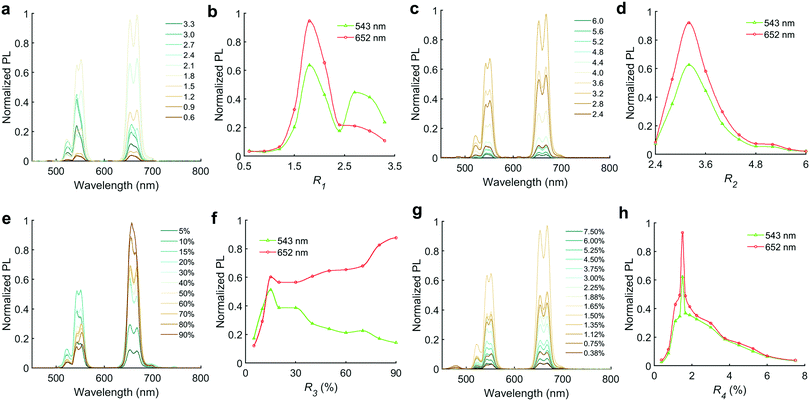 |
| Fig. 2 Optimization of reagent molar ratios R1–R4 for NaYF4:Yb,Er nanocrystals. (a and b) Effect of the Na/RE molar ratio (R1) on PL. (c and d) Effect of the F/RE molar ratio (R2) on PL. (e and f) Effect of the Yb/RE molar ratio (R3) on PL. (g and h) Effect of Er/RE molar ratio (R4) on the PL of UCNPs. All reactions were performed at 230 °C for 48 seconds. To index the green and red color, peaks at 543 nm and 652 nm were chosen, respectively. | |
To better compare the green and red emission from NaYF4:Yb,Er, the PL peaks at 652 nm (4F9/2 → 4I15/2) and 543 nm (4S3/2 → 4I15/2) were chosen as indices (see Fig. 5c). The optimized PL intensity occurs when R1 lies between 1.5 and 2.5 (peak at 1.8), where both the green and red light obtain their maximum intensity, with the intensity at 652 nm always being higher than at 543 nm. However, when R1 is larger than 2.5, the PL intensity at 543 nm exceeds the PL intensity at 652 nm. This switching of dominance between the two peaks allows facile tuning of the green to red emission ratio via modulation of the Na/RE molar ratio (R1).
The PL intensity of UCNPs was then optimized using an R2 (F/RE) scan, from 2.4 to 6.0, uncovering an optimal range of 3.2–3.6 (Fig. 2c and d). Interestingly this ratio is less than the 4
:
1 stoichiometry of NaYF4. Indeed, the most popular methods for NaYF4:Yb,Er synthesis (Table 1) all use a stoichiometry of 4 or higher.
Table 1 Reaction parameters of different methods for synthesis of NaYF4:Yb,Er UCNPs (R1 = Na/RE; R2 = F/RE; R3 = Yb/RE; R4 = Er/RE)
Precursors |
Solvent |
R
1 Na/RE |
R
2 F/RE |
R
3 Yb/RE (%) |
R
4 Er/RE (%) |
T (°C) |
Time (min) |
Phase |
Ref. |
Time used for high-throughput screening of emission characteristics.
|
Na(CF3COO) |
ODE/OA/OM |
1 |
4 |
20 |
2 |
250 |
45 |
Cubic |
28
|
RE(CF3COO)3 |
ODE/OA/OM |
1.8 |
4.8 |
20 |
2 |
330 |
30 |
Hexagonal |
|
OM |
2 |
5 |
20 |
2 |
330 |
60 |
Hexagonal |
42
|
NaF, RE-oleate |
ODE |
4.8 |
4.8 |
17 |
3 |
260 |
360 |
Hexagonal |
43
|
4.8 |
4.8 |
17 |
3 |
210 |
360 |
Cubic |
RE-oleate |
ODE/OA |
9.5 |
4 |
18 |
2 |
300 |
30 |
Hexagonal |
39
|
NaOH |
ODE/OA |
2.5 |
4 |
18 |
2 |
280 |
30 |
Cubic |
NH4F |
ODE/OA |
2.5 |
4 |
18 |
2 |
115–155 |
8–9 |
Cubic |
33
|
300 |
30 |
Hexagonal |
RE(CO3)3 |
OM |
17.6 |
4.4 |
11.5 |
2.2 |
280 |
120 |
Cubic/hexagonal/NaF |
44
|
Na2CO3 |
NH4F |
RE(III)-2-Ethylhexanoate |
TEGDME |
1.5–2.1 |
3.2–3.6 |
15 |
1.5 |
200–240 |
0.1–4a |
Cubic/hexagonal |
This work |
Na-2-Ethylhexanoate |
CsF |
Ratios R3 (Yb/RE) and R4 (Er/RE) control the rare-earth element doping (i.e. percentage versus total RE content) for NaYF4:Yb,Er. From the R3 scan we observed divergent behaviour for the red and green PL peaks (Fig. 2e and f). The PL from both red and green increases up to 15% doping, where they both form a local maximum. However, above 15%, green emission decreases steadily whereas the red emission increases again. This phenomenon means that the red to green intensity ratio may be adjusted via control of Yb content, R3. After normalizing the PL at 543 nm (Fig. 3a), it is evident that the red emission increases with Yb3+ doping concentration (from 5% to 90%) due to the influence of the cross-relaxation process.24,40 To achieve higher intensity emission from UCNPs, the activator doping ratio of Er3+ was optimized in Fig. 2g and h, where the optimum value of R4 was found to be 1.5%.
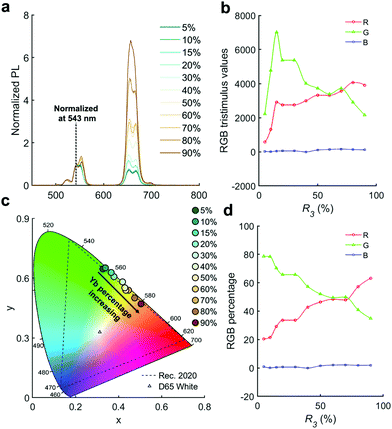 |
| Fig. 3 Emission color of NaYF4:Yb,Er UCNPs was tuned by changing the Yb doping degree. (a) Normalized spectra of NaYF4:Yb,Er UCNPs with Yb doping degree (R3) ranging from 5% to 90%. (b) Plot of the CIE1931 color coordinates of the NaYF4:Yb,Er UCNPs with different values of R3. (c) The RGB tristimulus values of the NaYF4:Yb,Er with different Yb molar ratios (R3). (d) The RGB percentage of UCNPs with different Yb molar ratios (R3). | |
Fig. 3c presents a CIE chromaticity diagram, where the CIE chromaticity coordinates vary from x = 0.3332, y = 0.6503 (green) to x = 0.5020, y = 0.4710 (orange) by increasing the doping ratio of Yb3+ from 5 to 90%. To obtain a better understanding of color evolution, spectra were converted into RGB tristimulus values (Fig. 3b) based on the CIE colour matching functions.41 The RGB percentages are shown in Fig. 3d. With increasing Yb3+ content, the percentage of red emission smoothly increases from 21 to 65%. In contrast, the percentage of green emission decreases from 79 to 34%. The blue emission is near zero across the whole range. Overall, we see that the emission color from NaYF4:Yb,Er UCNPs can be tuned from green to orange by changing the doping ratio of Yb3+ in RE.
After scanning precursor ratios for emission color tuning, we conducted a study of the impact of reaction temperature and residence time, with a view to further optimizing reaction parameters and understanding the influence of these factors on UCNP properties (Fig. 4). For temperature optimization, we used the optimal ratios established previously, i.e. R1 = 1.8, R2 = 3.2, R3 = 15%, and R4 = 1.5%, respectively, with a total reaction time of 48 seconds. In the range of 160 to 240 °C, there was a continual increase in PL from effectively zero at 160 °C (Fig. 4b). It is evident that the PL has not reached a global maximum by 240 °C, however this was the highest accessible temperature using the current reactor set-up, since temperatures higher than this led to gas evolution from the reaction solution (the b.p. of TEGDME is 275 °C). For the reaction time study, the temperature was set at 230 °C (to ensure sufficient PL intensity, whilst prohibiting gas evolution).
 |
| Fig. 4 Temperature and residence time optimization for NaYF4:Yb,Er nanocrystals. (a and b) Effect of the reaction temperature on PL. (c and d) Effect of residence time on PL. All reactions were performed with R1 = 1.8, R2 = 3.2, R3 = 14%, and R4 = 10%. To index the green and red color, peaks at 543 nm and 652 nm were chosen, respectively. | |
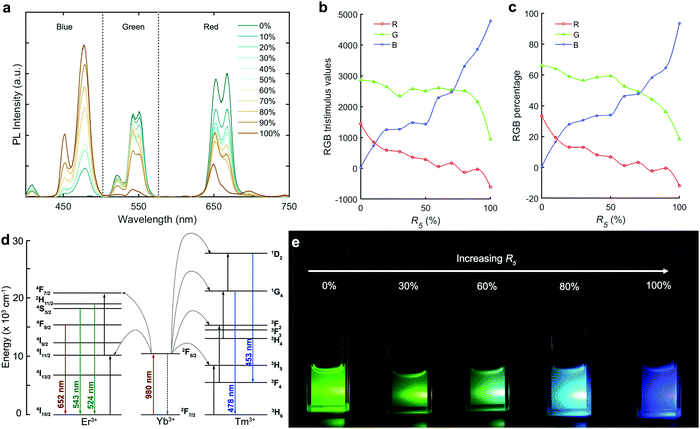 |
| Fig. 5 Synthesis of color-tunable emission from NaYF4:Yb,Er,Tm UCNPs. (a) Effect of the Tm molar ratio (R5) on the PL of UCNPs. (b) The RGB tristimulus values of the UCNPs with different Tm molar ratio of R5. (c) The RGB percentage of UCNPs with different Tm molar ratio of R5. (d) Energy level scheme and color origins in upconversion. (e) Luminescence of nanocrystals in ethanol. Images were captured separately in the dark, with laser excitation at 980 nm (power density of 4 W cm−2). | |
In this work we sought to minimize the required reaction time in order to increase the experimental throughput and the rapidity of optimization. As shown in Fig. 4c and d, the PL of the formed UCNPs increases with the residence time, approaching a plateau by ca. 4 minutes. Further, it was imperative to prove that the PL spectral shape (i.e. color) did not change substantially at longer reaction times versus the short reaction time to be used in our reactor. To confirm this, we ran a further bulk experiment to demonstrate that the PL (judged by the RGB%) was consistent between a 4 minute reaction at 230 °C (as used in the microfluidic reactor) and a longer ‘standard’ reaction (we chose 60 minutes and 270 °C as representative) in the flask, which would justify our premise of using the microfluidic reactor to tune emission color. Although the size and phase of the nanocrystals did not remain constant (Fig. S3a–e, ESI†), the RGB% values were consistent (Fig. S3f and g, ESI†). Therefore, we determined that a reaction of 4 minutes and temperature of 230 °C was sufficient for rapid color tuning of the UCNP emission in our microfluidic reactor.
In Table 1 we provide a direct comparison between the synthesis method developed herein and other approaches from the literature. Overall, we find a Na-rich and F-deficient reaction formulation (versus the stoichiometry of NaYF4:Yb,Er) benefits the PL intensity, while color can be adjusted by tuning the doping degree of Yb3+. This is an important observation, and worthy of further study in future work. Moreover, results highlight the powerful capabilities of high-throughput microfluidic screening platforms in understanding complex synthetic systems. Indeed, in an experiment with N synthetic variables (multiple precursors ratios, residence time, temperature, etc.), each having M levels (defining the selected range of each factor), the overall number of required experimental iterations scales as MN. The time needed for these iterations is then given by MN × t, where t is the time for one iteration. In the case of NaYF4:Yb,Er UCNPs, a reaction time of at least 60 minutes is typical for one bulk synthesis. Thus, conducting comprehensive parameter space mapping using standard flask-based approaches would rapidly become unmanageable due to the excessive time required, whereas it remains easily accessible using our modified synthesis route and screening approach.
2.3 Synthesis and optimization of NaYF4:Yb,Er,Tm
As has been shown, tuning of R1–R4, residence time and temperature for NaYF4:Yb,Er enables reaction optimization with respect to PL intensities and color tunability between green and orange. However, to obtain white-light-emitting UCNPs, another lanthanide ion is required for blue emission, whilst Er3+ contributes to green and red emission. Tm3+ ions are the typical blue source in upconversion, and are commonly co-doped into NaYF4 with Er3+ ions.42 White light emission is achieved by optimization of RGB emission to a quite specific doping ratio.9 Accordingly, we introduced a new variable, R5 (Tm/(Tm + Er)) to extract the optimal Tm
:
Er ratio. Specifically, we used the previously optimized parameters for NaYF4:Yb,Er, except that R1 was varied to control the red-green balance. Fig. 5a shows spectra originating from NaYF4:Yb,Er,Tm (20% Yb) with different degrees of Tm doping (R5). The blue region (with peaks at 478 nm and 453 nm) arises from Tm3+, while green (543 and 524 nm) and red (652 nm) emissions arise from the Er3+ doping (Fig. 5d). To better understand the color evolution, spectra were converted into RGB tristimulus values based on the CIE colour matching functions.41 As R5 increases, the blue value exhibits a continuous increase (Fig. 5b), and the red value a continuous decrease, across the entire range. The value of green emission was not significantly affected before an R5 of ca. 80%, but experienced a sharp decrease with any further increase in Tm3+ ratio. The percentage of the red and green emission (Fig. 5c) decreased with increasing R5 (decreasing Er3+). The percentage of blue color increased from 0 to 95%, as R5 increased from 0 to 100%. As a result of the low percentage of red color across the whole range of R5, UCNP emission gradually changed from green to cold white and to blue with the different degrees of doping, as shown in Fig. 5e. The cold white light emitting NaYF4:Yb,Er,Tm nanocrystals were obtained for R5 values between 70 and 80%. Fig. 5d shows the energy level diagram of Yb3+–Er3+–Tm3+ tri-doped NaYF4 nanocrystals. The Yb3+ is initially excited at 980 nm from its ground state 2F7/2 level to the 2F5/2 level, followed by energy transfer to Er3+ and Tm3+. The Er3+ then undergoes 4S3/2 → 4I15/2 (543 nm, green), 2H11/2 → 4I15/2 (524 nm, green), and 4F9/2 → 4I15/2 (652 nm, red) transitions. The Tm3+ undergoes 1G4 → 3H6 (478 nm, blue) and 1D2 → 3F4 (453 nm, blue) transitions. In order to obtain white-light emission at a high excitation power, a high ratio of R5 is required to ensure sufficient blue emission intensity. Fig. S4 (ESI†) shows the CIE chromaticity diagram for NaYF4:Yb,Er,Tm UCNPs (20% Yb) with varying values of R5. The CIE chromaticity coordinates change from x = 0.3776, y = 0.6091 (green) to x = 0.2292, y = 0.3585 (cold white), ending at x = 0.1475, y = 0.1121 (blue), when increasing the doping ratio of Tm3+ from 0 to 100%. With 20% Yb3+, we achieve ‘cold’ white light rather than ‘true’ white due to a lack of red. However, by increasing the doping degree of Yb3+ to 60%, we can increase the red light content (Fig. S5, ESI†), thereby achieving true white light emission. The emission spectra of NaYF4:Yb,Er,Tm nanocrystals with 60% Yb and variable Tm doping (R5) are shown in Fig. S5a (ESI†). The red and green emission were similar across the entire range of R5 (Fig. S5c and d, ESI†), which benefits targeting of true white light emission. The corresponding CIE chromaticity diagram (Fig. S5b, ESI†) shows an R5 of 60–70%, yielding white light under 980 nm excitation (Movie S1, ESI†). Overall, the emission colors of the products display a wide variation from orange to blue, and pass through the white emission zone.
The above results powerfully demonstrate the merits of rapid reaction tuning for NaYF4:Yb,Er,Tm UCNPs in obtaining precise multicolor emission in the visible region when exited by a single wavelength light source. Fig. 6a reports that the emission color of NaYF4:Yb,Er,Tm can be adjusted in two dimensions, by varying the Yb percentage to tune the green–red ratio, and varying the Tm percentage to tune the green–blue ratio. Fig. 6b shows the XRD pattern of three samples, with R5 equal to 0, 70 and 100%, yielding NaYF4:Yb,Tm, NaYF4:Yb,Er,Tm, and NaYF4:Yb,Er, respectively. All three samples exhibit peaks from both the cubic (α-) and hexagonal (β-) phases. The diffraction peaks from the β-phase of the 0% sample (NaYF4:Yb,Er) were weaker than those for the 70% (NaYF4:Yb,Er,Tm) or 100% (NaYF4:Yb,Tm) samples. This implies that the β-phase ratio in the absence of Tm is less than in the Tm-doped UCNPs. TEM images (Fig. S6, ESI†) show that ‘popcorn-like’ NaYF4:Yb,Er nanocrystals were obtained. The sizes of the nanocrystals (Fig. 6c–e) did not significantly vary as a function of Tm doping. The R5 at 0, 70 and 100% samples exhibited average diameters of 53.4 ± 8.5, 49.5 ± 7.7, and 50.2 ± 8 nm, respectively. Therefore, the size of the NaYF4:Yb,Er,Tm UCNPs seems to primarily depend on the NaYF4:Yb matrix rather than the doping degree of the activators.
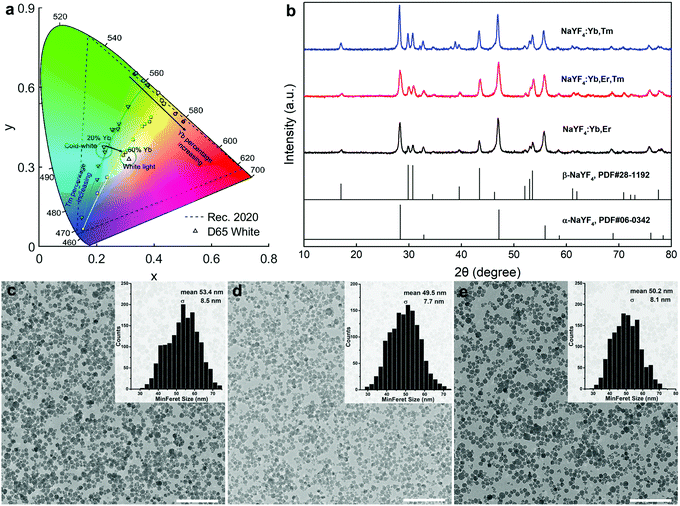 |
| Fig. 6 (a) Plot of CIE1931 color coordinates of the NaYF4:Yb,Er,Tm UCNPs with different value of R5 at Yb doping degree at 20% and 60%. (b) XRD patterns of NaYF4:Yb,Er,Tm UCNPs with R5 at 0% (black), 70% (red), and 100% (blue). (c–e) TEM images of the nanocrystals with R5 at 0%, 70%, and 100%. Scale bar is 500 nm. | |
2.4 Application of NaYF4:Yb,Er,Tm nanocrystals
To demonstrate the potential of using our color-tuned UCNPs in a thin film state, we fabricated a simple anti-counterfeit device. Here, a logo appears homogeneous under ambient light, but colored letters are revealed under infrared excitation. We recreated the ETH logo in a PDMS-based microdevice, which was fabricated via standard soft lithography. Subsequently, NaYF4:Yb,Er,Tm (20% Yb) UCNPs in ethanol (with R5 at 0, 70 and 100%, representing three different emission colour profiles, see Fig. S7, ESI†) were injected into the three character chambers separately, and dried by solvent evaporation. A smartphone camera was then used to capture images of the device through a simple lens–filter combination (Fig. 7a). Without the 980 nm excitation, all three characters appear identical (Fig. 7b). Once excited, the three different colors (green, cold white, and blue in Fig. 7c), can clearly be observed and imaged with the smartphone camera.
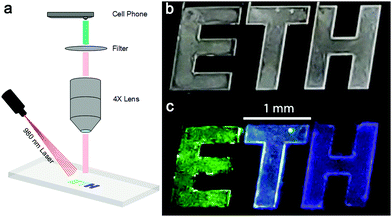 |
| Fig. 7 Color-tunable NaYF4:Yb,Er,Tm nanocrystals applied for anti-countering. (a) Concept for anti-counterfeiting with imaging by a smartphone camera. (b) ETH logo captured without excitation. (c) Three different colors emerging under the 980 nm excitation. | |
3 Conclusions
In summary, we have developed an entirely new synthesis strategy, in term of reagents, solvent and ligands, for the preparation and facile color tuning of NaYF4:Yb,Er,Tm UCNPs in a high-throughput manner. By taking advantage of the time and materials efficiency associated with droplet-based microfluidic reactors, compositional element ratios (R1 to R5), as well as the residence time and reaction temperature could be optimized via inline PL analysis. Using a guided optimization of reaction parameters, color-tunable UCNPs have been synthesized by changing the doping degree of the Yb sensitizer (allowing controlled green–orange tuning) and the ratio between activators Tm–Er (achieving controlled green–blue tuning). With this two dimensional tuning, single white-light emitting NaYF4:Yb,Er,Tm nanocrystals have been synthesized. As evidenced by the fabrication and testing of an anti-counterfeit device, the formed nanocrystals still exhibit excellent photoluminescence in the dried state. Finally, with this newly developed synthesis strategy, we have demonstrated great potential for fast screening of other host matrices, and coordinating ligands, as well as core–shell structures for various UCNP applications. Future work will focus on modifying the reactor to tolerate higher temperatures, testing solvents with higher boiling points, and exploring a wider range of precursors to measure their effects on particle properties.
Author contributions
Shangkun Li: conceptualization, investigation (all experiments), visualization, writing – original draft. Yingchao Meng: investigation (anticounterfeit device). Yujia Guo: investigation (COMSOL modelling). Tian Liu: investigation (XRD). Stavros Stavrakis: supervision, methodology, writing – review & editing. Philip D. Howes: conceptualization, supervision, writing – original draft, review & editing. Andrew J. deMello: funding acquisition, project administration, supervision, writing – review & editing.
Conflicts of interest
The authors have no conflicts of interest to report.
Acknowledgements
The authors acknowledge partial financial support from ETH Zürich. S. L. acknowledges support from the China Scholarship Council (CSC), Grant #201608140097. S. L. would like to thank Léonard Bezinge for assistance in CIE plotting. P. D. H. and S. S. would like to thank the Swiss National Science Foundation (SNSF) for support via a Spark Grant (Project No. CRSK-2_190750). The authors are grateful to the ScopeM center at ETH for technical assistance in TEM imaging.
References
- Z. Chen, C. L. Ho, L. Wang and W. Y. Wong, Single-Molecular White-Light Emitters and Their Potential WOLED Applications, Adv. Mater., 2020, 32, 1903269 CrossRef CAS.
- P. He, Y. Shi, T. Meng, T. Yuan, Y. Li, X. Li, Y. Zhang, L. Fan and S. Yang, Recent advances in white light-emitting diodes of carbon quantum dots, Nanoscale, 2020, 12, 4826–4832 RSC.
- H. Su, Y. Chen and K. Wong, Recent Progress in White Light-Emitting Electrochemical Cells, Adv. Funct. Mater., 2019, 1906898 Search PubMed.
- S. Sivakumar, F. C. J. M. Van Veggel and M. Raudsepp, Bright white light through up-conversion of a single NIR source from sol-gel-derived thin film made with Ln3+-doped LaF3 nanoparticles, J. Am. Chem. Soc., 2005, 127, 12464–12465 CrossRef CAS.
- E. Jang, S. Jun, H. Jang, J. Lim, B. Kim and Y. Kim, White-light-emitting diodes with quantum dot color converters for display backlights, Adv. Mater., 2010, 22, 3076–3080 CrossRef CAS.
- T. Higuchi, H. Nakanotani and C. Adachi, High-Efficiency White Organic Light-Emitting Diodes Based on a Blue Thermally Activated Delayed Fluorescent Emitter Combined with Green and Red Fluorescent Emitters, Adv. Mater., 2015, 27, 2019–2023 CrossRef CAS.
- K. T. Kamtekar, A. P. Monkman and M. R. Bryce, Recent advances in white organic light-emitting materials and devices (WOLEDS), Adv. Mater., 2010, 22, 572–582 CrossRef CAS.
- P. T. Furuta, L. Deng, S. Garon, M. E. Thompson and J. M. J. Fréchet, Platinum-functionalized random copolymers for use in solution-processible, efficient, near-white organic light-emitting diodes, J. Am. Chem. Soc., 2004, 126, 15388–15389 CrossRef CAS.
- C. Zhang, L. Yang, J. Zhao, B. Liu, M. Y. Han and Z. Zhang, White-Light Emission from an Integrated Upconversion Nanostructure: Toward Multicolor Displays Modulated by Laser Power, Angew. Chem., Int. Ed., 2015, 54, 11531–11535 CrossRef CAS.
- Z. He, W. Zhao, J. W. Y. Lam, Q. Peng, H. Ma, G. Liang, Z. Shuai and B. Z. Tang, White light emission from a single organic molecule with dual phosphorescence at room temperature, Nat. Commun., 2017, 8, 416 CrossRef.
- S. Wen, J. Zhou, K. Zheng, A. Bednarkiewicz, X. Liu and D. Jin, Advances in highly doped upconversion nanoparticles, Nat. Commun., 2018, 9, 2415 CrossRef.
- D. Sarkar, S. Ganguli, T. Samanta and V. Mahalingam, Design of Lanthanide-Doped Colloidal Nanocrystals: Applications as Phosphors, Sensors, and Photocatalysts, Langmuir, 2019, 35, 6211–6230 CrossRef CAS.
- G. Tessitore, G. A. Mandl, M. G. Brik, W. Park and J. A. Capobianco, Recent insights into upconverting nanoparticles: spectroscopy, modeling, and routes to improved luminescence, Nanoscale, 2019, 11, 12015–12029 RSC.
- K. Zheng, K. Y. Loh, Y. Wang, Q. Chen, J. Fan, T. Jung, S. H. Nam, Y. D. Suh and X. Liu, Recent advances in upconversion nanocrystals: Expanding the kaleidoscopic toolbox for emerging applications, Nano Today, 2019, 29, 100797 CrossRef CAS.
- R. D. Mehlenbacher, R. Kolbl, A. Lay and J. A. Dionne, Nanomaterials for in vivo imaging of mechanical forces and electrical fields, Nat. Rev. Mater., 2018, 3, 17080 CrossRef CAS.
- Y. Wang, S. Song, S. Zhang and H. Zhang, Stimuli-responsive nanotheranostics based on lanthanide-doped upconversion nanoparticles for cancer imaging and therapy: current advances and future challenges, Nano Today, 2019, 25, 38–67 CrossRef CAS.
- J. Zhou, J. L. Leaño, Z. Liu, D. Jin, K.-L. Wong, R.-S. Liu and J.-C. G. Bünzli, Impact of Lanthanide Nanomaterials on Photonic Devices and Smart Applications, Small, 2018, 14, 1801882 CrossRef.
- C. D. S. Brites, S. Balabhadra and L. D. Carlos, Lanthanide-Based Thermometers: At the Cutting-Edge of Luminescence Thermometry, Adv. Opt. Mater., 2019, 7, 1801239 CrossRef.
- Z. Li, H. Yuan, W. Yuan, Q. Su and F. Li, Upconversion nanoprobes for biodetections, Coord. Chem. Rev., 2018, 354, 155–168 CrossRef CAS.
- Y. Hu, Q. Shao, X. Deng, D. Song, S. Han, Y. Dong and J. Jiang, Thermally induced multicolor emissions of upconversion hybrids with large color shifts for anticounterfeiting applications, J. Mater. Chem. C, 2019, 7, 11770–11775 RSC.
- S. Wang, J. Chen, J. Lin, C. Yang, F. Huang and D. Chen, Nanocrystallization of lanthanide-doped KLu2F7-KYb2F7 solid-solutions in aluminosilicate glass for upconverted solid-state-lighting and photothermal anti-counterfeiting, J. Mater. Chem. C, 2019, 7, 14571–14580 RSC.
- A. J. Evangelista, M. Ivanchenko, A. F. Myers, L. N. McAnulty, G. K. M. Payne and H. Jing, Multi-shelled upconversion nanostructures with enhanced photoluminescence intensity via successive epitaxial layer-by-layer formation (SELF) strategy for high-level anticounterfeiting, J. Mater. Chem. C, 2020, 8, 5692–5703 RSC.
- J. Wang and P. A. Tanner, Upconversion for white light generation by a single compound, J. Am. Chem. Soc., 2010, 132, 947–949 CrossRef CAS.
- T. Wang, H. Yu, C. K. Siu, J. Qiu, X. Xu and S. F. Yu, White-Light Whispering-Gallery-Mode Lasing from Lanthanide-Doped Upconversion NaYF4 Hexagonal Microrods, ACS Photonics, 2017, 4, 1539–1543 CrossRef CAS.
- K. Lingeshwar Reddy, R. Balaji, A. Kumar and V. Krishnan, Lanthanide Doped Near Infrared Active Upconversion Nanophosphors: Fundamental Concepts, Synthesis Strategies, and Technological Applications, Small, 2018, 14, 1801304 CrossRef.
- Y. Sun, Y. Chen, L. Tian, Y. Yu, X. Kong, J. Zhao and H. Zhang, Controlled synthesis and morphology dependent upconversion luminescence of NaYF4:Yb, Er nanocrystals, Nanotechnology, 2007, 18, 275609 CrossRef.
- Z. Li and Y. Zhang, An efficient and user-friendly method for the synthesis of hexagonal-phase NaYF4:Yb, Er/Tm nanocrystals with controllable shape and upconversion fluorescence, Nanotechnology, 2008, 19, 345606 CrossRef.
- H. X. Mai, Y. W. Zhang, R. Si, Z. G. Yan, L. D. Sun, L. P. You and C. H. Yan, High-quality sodium rare-earth fluoride nanocrystals: Controlled synthesis and optical properties, J. Am. Chem. Soc., 2006, 128, 6426–6436 CrossRef CAS.
- B. K. H. Yen, N. E. Stott, K. F. Jensen and M. G. Bawendi, A Continuous-Flow Microcapillary Reactor for the Preparation of a Size Series of CdSe Nanocrystals, Adv. Mater., 2003, 15, 1858–1862 CrossRef CAS.
- H. Nakamura, Y. Yamaguchi, M. Miyazaki, H. Maeda, M. Uehara and P. Mulvaney, Preparation of CdSe nanocrystals in a micro-flow-reactor, Chem. Commun., 2002, 2844–2845 RSC.
- J. B. Edel, R. Fortt, J. C. DeMello and A. J. DeMello, Microfluidic routes to the controlled production of nanoparticles, Chem. Commun., 2002, 1136–1137 RSC.
-
H. Liu, O. Jakobsson, C. T. Xu, H. Xie, T. Laurell and S. Andersson-Engels, in Colloidal Quantum Dots/Nanocrystals for Biomedical Applications VI, ed. W. J. Parak, K. Yamamoto and M. Osinski, SPIE, 2011, vol. 7909, p. 790917 Search PubMed.
- D. Liu, Y. Jing, K. Wang, Y. Wang and G. Luo, Reaction study of α-phase NaYF4:Yb,Er generation via a tubular microreactor: Discovery of an efficient synthesis strategy, Nanoscale, 2019, 11, 8363–8371 RSC.
- J. Sui, J. Yan, K. Wang and G. Luo, Efficient synthesis of lithium rare-earth tetrafluoride nanocrystals via a continuous flow method, Nano Res., 2020, 13, 2837–2846 CrossRef CAS.
- I. Lignos, S. Stavrakis, G. Nedelcu, L. Protesescu, A. J. DeMello and M. V. Kovalenko, Synthesis of Cesium Lead Halide Perovskite Nanocrystals in a Droplet-Based Microfluidic Platform: Fast Parametric Space Mapping, Nano Lett., 2016, 16, 1869–1877 CrossRef CAS.
- J. Nette, P. D. Howes and A. J. DeMello, Microfluidic Synthesis of Luminescent and Plasmonic Nanoparticles: Fast, Efficient, and Data-Rich, Adv. Mater. Technol., 2020, 2000060 CrossRef CAS.
- S. Li, R. W. Baker, I. Lignos, Z. Yang, S. Stavrakis, P. D. Howes and A. J. DeMello, Automated microfluidic screening of ligand interactions during the synthesis of cesium lead bromide nanocrystals, Mol. Syst. Des. Eng., 2020, 5, 1118–1130 RSC.
- Y. Ding, P. D. Howes and A. J. Demello, Recent Advances in Droplet Microfluidics, Anal. Chem., 2020, 92, 132–149 CrossRef CAS.
- R. A. Janjua, C. Gao, R. Dai, Z. Sui, M. A. Ahmad Raja, Z. Wang, X. Zhen and Z. Zhang, Na+-Driven Nucleation of NaYF4:Yb,Er Nanocrystals and Effect of Temperature on Their Structural Transformations and Luminescent Properties, J. Phys. Chem. C, 2018, 122, 23242–23250 CrossRef CAS.
- F. Vetrone, J. C. Boyer, J. A. Capobianco, A. Speghini and M. Bettinelli, Significance of Yb3+ concentration on the upconversion mechanisms in codoped Y2O3:Er3+, Yb3+ nanocrystals, J. Appl. Phys., 2004, 96, 661–667 CrossRef CAS.
- H. S. Fairman, M. H. Brill and H. Hemmendinger, How the CIE 1931 color-matching functions were derived from Wright-Guild data, Color Res. Appl., 1997, 22, 11–23 CrossRef.
- G. S. Yi and G. M. Chow, Synthesis of hexagonal-phase NaYF4:Yb,Er and NaYF4:Yb,Tm nanocrystals with efficient up-conversion fluorescence, Adv. Funct. Mater., 2006, 16, 2324–2329 CrossRef CAS.
- Y. Wei, F. Lu, X. Zhang and D. Chen, Synthesis of oil-dispersible hexagonal-phase and hexagonal-shaped NaYF4:Yb,Er nanoplates, Chem. Mater., 2006, 18, 5733–5737 CrossRef CAS.
- H. Schäfer, P. Ptacek, H. Eickmeier and M. Haase, Synthesis of hexagonal Yb3+,Er3+-doped NaYF4 nanocrystals at low temperature, Adv. Funct. Mater., 2009, 19, 3091–3097 CrossRef.
Footnote |
† Electronic supplementary information (ESI) available. See DOI: 10.1039/d0tc04309e |
|
This journal is © The Royal Society of Chemistry 2021 |
Click here to see how this site uses Cookies. View our privacy policy here.