DOI:
10.1039/D1TA06530K
(Paper)
J. Mater. Chem. A, 2021,
9, 23631-23642
Sorption-enhanced mixed-gas transport in amine functionalized polymers of intrinsic microporosity (PIMs)†
Received
2nd August 2021
, Accepted 1st October 2021
First published on 4th October 2021
Abstract
Pure-gas transport performance rarely matches mixed-gas performance for industrially relevant membrane applications. While significant effort has focused on studying the adverse effects of plasticization, an additional phenomenon known as competitive sorption can be used to improve performance, resulting in mixed-gas permselectivities that far exceed pure-gas predictions. Such findings are rare, but recent synthetic discoveries of functional polymers of intrinsic microporosity (PIMs) now provide a platform to investigate if competitive sorption effects can improve separation performance in complex mixtures by offsetting the adverse effects of plasticization. In this work, we report high-pressure and mixed-gas transport properties for six PIMs with identical benzodioxane backbones and a diverse set of backbone functionalities. Low-pressure mixed-gas tests revealed a relationship between CO2 sorption affinity of the PIMs and improvements in CO2/CH4 and CO2/N2 mixed-gas permselectivity compared to pure-gas scenarios. The amine-functionalized PIM-1 (PIM-NH2) showed an unprecedented 140% and 250% increase in equimolar CO2/CH4 and CO2/N2 mixed-gas permselectivity, respectively, compared to that of pure-gas tests at 2 atm. Additionally, PIM-NH2 retained high CO2/CH4 mixed-gas permselectivity (>20) up to pressures of 26 atm in 50/50 CO2/CH4 mixtures, demonstrating a resistance to plasticization. Pure-gas sorption and mixed-gas permeation for the six PIMs were compared to elucidate structure–property relationships. Results demonstrate the remarkable potential of primary amine functionalization for developing highly sorption-selective and plasticization-resistant membranes for gas separations.
Introduction
Global CO2 emissions reached over 30 Gt in 2020, while U.S. natural gas consumption has increased by approximately 30% in the past decade.1 In the U.S. chemical industry alone, about 50% of the energy consumption results from heat-intensive processes such as distillation and absorption, which also produce indirect GHG emissions.2 As such, implementing energy-efficient separation alternatives and carbon capture solutions is critical to meet the U.S. Department of Energy's emission reduction target of 80% by 2050.3 Unlike traditional unit operations, polymer membranes have a small, modular footprint and facile operation, and do not require toxic solvents or regeneration.4 However, polymer performance is limited by a trade-off between permeability and selectivity, which is defined by a theory that does not consider mixture effects.5,62
Since 2004, microporous polymers such as polymers of intrinsic microporosity (PIMs),6 thermally rearranged (TR) polymers,7 ROMPs,8 and CANALs9 have shown noteworthy performance enhancements. Benzotriptycene-based PIMs, for instance, redefined pure-gas CO2/N2 and CO2/CH4 upper bound benchmarks in 2019.10 Additional upper bounds for H2-based separations,11 and gas mixtures12 have been reported, where microporous polymers continue to outperform traditional polymers. Microporous polymers are designed with rigid monomers of high configurational free volume (e.g., spirobisindane and triptycene), which result in inefficiently packed solid-state structures with high internal surface areas, intrachain rigidity, and excellent permeability–selectivity combinations.13–16 However, their intrachain rigidity alone has been insufficient to mitigate plasticization effects at high pressures.17,18
Another important aspect required for industrial deployment of PIM-based membranes is understanding performance for complex mixtures. To this end, several groups have been investigating mixed-gas sorption for CO2/CH4, CO2/C2H4, CO2/N2O, and CO2/C2H6 mixtures in various polymers, including TZ-PIM,19 PIM-1,19 PTMSP,19 AO-PIM,20 6FDA-mPDA,21 PIM-Trip-TB,22 6FDA-HAB and its TR450 analogue,23 cellulose triacetate (CTA),24 PDMS,25,26 6FDA-TADPO,27 PEO,28 and PMMA.29,30 Sorption of complex C2H6/CO2/CH4 ternary mixtures in PIM-1 has also been recently reported, highlighting the importance of competition in influencing transport performance under realistic conditions.31 Mixed-gas sorption studies demonstrate that gases with high polymer affinity (e.g., CO2) can reduce the sorption of co-penetrants in a gas mixture, increasing sorption selectivity and permselectivity in separations such as CO2/CH4 and CO2/N2. In pristine polymers at high pressures, however, plasticization effects can decrease diffusion selectivity and permselectivity beyond improvements achieved by competition effects.23 This trade-off is particularly acute for diffusion-selective polymers including polyimides and PIMs.23
Recently, Eddaoudi and Koros have demonstrated that chemical functionality can be used to simultaneously improve sorption selectivity and plasticization resistance in MOF-based mixed-matrix membranes.32,33 When considering condensable penetrants (i.e., H2S and CO2) in these MMMs, competitive sorption can improve H2S and CO2 permeation, while controlled plasticization at high pressures can retain good CO2/CH4 (>20) and H2S/CH4 (>30) permselectivities. Related findings have also been reported for transport of binary mixtures in polymer films. One notable example relates to –OH modified PIM-polyimides (PIM-PIs), where Swaidan et al. and Alghunaimi et al. reported the syntheses of hydroxyl bearing PIM-PIs, TPDA-APAF34 and TDA1-APAF,35 respectively, which showed 11% and 17% higher mixed-gas CO2/CH4 permselectivities compared to pure-gas tests, respectively. Plasticization resistance improved in these PIM-PIs due to interchain rigidity provided by hydrogen bonding.
A related approach to increase sorption selectivity involves post-synthetic functionalization of the polymer backbone.36,37 Several functionalized variants of PIM-1 have been reported, including the incorporation of tetrazole,38 carboxylic acid,39–42 amidoxime,38 thioamide,43 amine,44 and amide45 groups. In these examples, however, functionalization has consistently resulted in reduced free volume and correspondingly low pure-gas permeabilities. With respect to sorption, most functionalized PIMs show moderate changes in CO2 uptake, with the exception of amine-functionalized PIM-1 (PIM-NH2).44,46 Unfortunately, however, pure-gas performance for PIM-NH2 is significantly below the 2008 upper bound47 and mixed-gas transport has not been studied. Given the extensive palette of post-synthetic chemistries available to PIMs, understanding how functionality affects mixed-gas performance and competition is crucial for the design of high-performing microporous membranes for industrially relevant conditions.
Herein, we elucidate direct structure–property relationships between polymer chemistry, sorption affinity, and mixed-gas competition effects for six functionalized PIM-1 derivatives. Mixed-gas sorption was modelled from pure-gas isotherms to assess the role of competitive sorption on mixed-gas permeation for CO2-based separations under industrially relevant feed compositions. Pure- and mixed-gas high-pressure permeation tests were also considered to evaluate the role of polymer functionality on plasticization. Sorption selectivity and mixed-gas permeability enhancements of these PIMs were compared to reported literature for microporous polymers, revealing that PIM-NH2 had the most improved competitive sorption properties among known PIMs. Additionally, PIM-NH2 showed an exceptional resistance to plasticization compared to PIM-1. These results underscore the promise of primary amine functionalization for significantly enhancing mixed-gas sorption selectivity and maintaining excellent separation performance at high pressures.
Experimental
Materials and film preparation
PIM-1 analogues with chemical moieties including nitrile (–CN, PIM-1), carboxylic acid (–COOH, PIM-COOH), amine (–CH2NH2, PIM-NH2 and PIM-deBOC(acid)), tert-butoxycarbonyl (–CH2NHCOOC(CH3)3, PIM-tBOC), and partial urea (–NHCONH–, PIM-deBOC(thermal)), represented in Fig. 1, were synthesized or chemically functionalized as described in our previous work.40,48 Methanol (HPLC, ≥99.9%) was purchased from Sigma Aldrich and used as received. PIM-NH2 derivatives including PIM-deBOC(thermal), PIM-deBOC(acid), and PIM-tBOC were soaked in methanol for 24 h and dried under vacuum at 130 °C for 12 h prior to all characterization tests. PIM-COOH samples were not methanol treated due to structural fragility when submerged in methanol. To enable robust comparison between samples, PIM-1 films were prepared with and without methanol treatment. Regardless of these treatment steps, all films were vacuum-dried at 130 °C for 12 h. Films were aged at ambient conditions for 290–448 days.
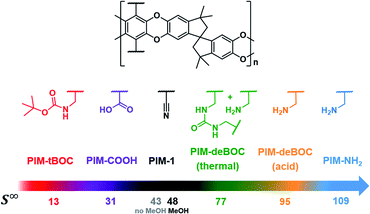 |
| Fig. 1 Generalized PIM-1 backbone and functionalities for the six PIM-1 analogues investigated in this study, along with their corresponding sorption coefficients at infinite dilution (S∞) in cmSTP3 cmpol−3 atm−1. | |
Pure- and mixed-gas permeation
Permeation tests were performed using automated constant-volume, variable-pressure permeation systems from Maxwell Robotics. A detailed experimental procedure for pure-gas testing is provided in the ESI.† For mixed-gas tests, a built-in air heating system was used to maintain the temperature at 35 °C and the gas mixture compositions in the feed and the permeate streams were measured using an Agilent 7890B gas chromatograph (GC). Aged film samples were loaded into a permeation cell in the mixed-gas system and degassed under vacuum for at least 8 h. The feed mixtures were generated at the desired composition using Bronkhorst mass flow controllers, which maintained high flowrates (300–800 sccm) to avoid concentration polarization. A stage cut of <0.1% was used for all tests, as recommended elsewhere.49,50 The upstream pressure was set using a proportional–integral-derivative (PID) controller. Steady state was verified by confirming linearity in downstream pressure rise as a function of time for the mixture and evaluation of the permeate composition over time for multiple time points. The duration of the equilibration step for each mixed-gas test was determined to be at least 10 times the pure-gas time lags of the slower permeating species (i.e., CH4 or N2) calculated at 1 atm and 35 °C. At steady state, the permeating mixture was collected into a degassed volume under static vacuum, after which, gas was injected into the GC for analysis. For a typical experiment, pure-gas CH4 and CO2 permeability were first analyzed in the mixed-gas system at 1 atm. Next, nine CO2/CH4 mixture compositions, defined throughout the manuscript by mole percentage, were sequentially tested for CO2/CH4 compositions of 10/90, 20/80, 30/70, 40/60, 50/50, 60/40, 70/30, 80/20, and 90/10. High performing samples were also tested using pure H2 and N2, and 50/50 mixtures of H2/CH4, H2/N2, and CO2/N2 at a total pressure of 2 atm. Following these low-pressure tests, the CO2/CH4 feed composition was fixed at 50/50 and the total mixed-gas pressure was increased from 2 to 26 atm.
For CO2 pressures above 1 atm, the fugacity was calculated to account for non-ideal gas behaviour using the virial equation of state.51 The mole fraction of gas in the downstream was calculated using GC calibration curves. The following relationships were used to calculate pure-gas permeability and the mixed-gas permeability for component i of a binary mixture, respectively:
| 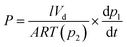 | (1) |
| 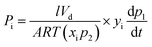 | (2) |
where
P is the permeability in barrer (10
−10 cm
STP3 cm cm
−2 cm
Hg−1 s
−1),
l is the film thickness,
Vd is the volume in the downstream,
p2 is the average upstream pressure,
A is the active cross-sectional area,
R is the ideal gas constant,
T is the absolute temperature, d
p1/d
t is the pressure rise in the downstream minus the leak rate. For mixed-gas calculations,
y and
x are the mole fractions of gas i in the downstream and the upstream, respectively, and
p1≪
p2, where
p1 < 10 Torr. Film thicknesses and treatment conditions are summarized in Table S1.
†
High-pressure pure-gas sorption
CO2, CH4, N2, and O2 sorption isotherms for methanol treated PIM-1, PIM-NH2, PIM-deBOC(acid), and PIM-deBOC(thermal) were collected at 35 °C using an automated pressure decay system from Maxwell Robotics. Detailed protocols for this test are provided in our previous work.52
Sorption in glassy polymers is commonly described through the dual-mode sorption (DMS) model:53,54
| 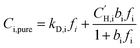 | (3) |
where
Ci is the concentration of gas i in the polymer (cm
STP3 cm
pol−3),
fi is the fugacity (atm) at equilibrium,

is the Langmuir sorption capacity (cm
STP3 cm
pol−3),
kD,i is the Henry's law constant (cm
STP3 cm
pol−3 atm
−1), and
bi is the Langmuir affinity constant (atm
−1).
55 Isotherms were fit to the DMS model
via nonlinear least squares fitting by constraining ln(
kD)
versus critical temperature (
Tc) to have the same slope as ln(
S)
versus Tc at 10 atm, where
S is the sorption coefficient.
56,57
Pure-gas DMS model parameters can be used to predict mixed-gas sorption as proposed by Koros.58,59 In this model, sorption into the equilibrium mode is assumed to be unaffected by competition, while co-penetrants compete to sorb into the Langmuir mode of the polymer.23 Competition for the Langmuir mode results in a depression of mixed-gas sorption for each penetrant in the mixture, which can be estimated using pure-gas DMS model parameters for penetrant i (kD,i, bi, and
) and the Langmuir affinity constant for the co-penetrant j in the binary mixture (bj). The concentration of penetrant i sorbed in the polymer in the presence of a co-penetrant j is:
| 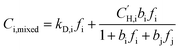 | (4) |
where
f is the partial fugacity of each penetrant. Mixed-gas sorption isotherms were modeled using constrained DMS parameters. Mixed-gas fugacities were calculated by invoking the Lewis–Randall approximation.
60
Pure- or mixed-gas sorption coefficients can be calculated by dividing the gas concentration by the corresponding fugacity as shown in eqn (5) and (6), respectively:
| 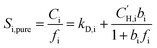 | (5) |
| 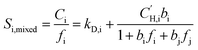 | (6) |
Sorption–diffusion model
Gas transport through a polymer is often described using the sorption–diffusion model, where the permeability is defined as the product of the diffusion coefficient (cm2 s−1) and the sorption coefficient (cmSTP3 cmpol−3 atm−1):61
Pure- and mixed-gas permselectivity (α) is defined as the ratio of the permeabilities for pure- and mixed-gas conditions:
| 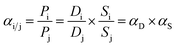 | (8) |
where
αD is the diffusion selectivity and
αS is the sorption selectivity. Pure-gas sorption selectivities were estimated using best-fit curves of experimental data, while mixed-gas sorption selectivities were calculated from sorption isotherms modeled using constrained DMS parameters, as described earlier. Diffusion selectivities were then calculated as the ratio between pure- or mixed-gas permselectivity and sorption selectivity.
Results and discussion
In this work, mixed-gas and high-pressure transport properties of six functionalized PIMs were evaluated. As shown in Fig. 1, the PIMs investigated have identical backbones with distinct functionalities of varying CO2 affinity including the nitrile (–CN), carboxylic acid (–COOH), amine (–CH2NH2), tert-butoxycarbonyl (–CH2NHCOOC(CH3)3, –tBOC), and urea (–NHCONH–). As previously reported, PIM-deBOC(acid) and PIM-deBOC(thermal) are chemical analogues of PIM-NH2, but with distinct free volume architecture and for PIM-deBOC(thermal), a light degree of urea crosslinks.48 In our prior work, the influence of physical packing on the transport properties of these polymers were investigated under pure-gas conditions and through time-lag analysis. In this work, the influence of side-group chemistry on mixed-gas transport is probed through mixed-gas permeation and direct sorption.
In addition to mixture considerations and high-pressure, in many separation applications, PIMs will lose permeability and increase selectivity over time through a process known as physical aging, but few studies report transport performance for structural analogues that have been systematically aged.63,64 Aging effects are also accelerated for thin films, and Jue et al. have demonstrated that industrially relevant PIM-1 hollow fibers have separation performance reminiscent of PIM-1 thick films that have aged for several years.65,66 To consider performance under more realistic scenarios, all films were aged for over 290 days. After aging, all films demonstrated the expected loss in permeability and increase in permselectivity typically observed for glassy polymers,63 with the exception of PIM-tBOC, which had little change in permselectivity and permeability due to its low fractional free volume (Fig. S1†).48
Variable composition mixed-gas permeation
CO2/CH4 mixtures.
Aged polymer films were first evaluated with CO2/CH4 binary mixed-gas feeds at a low total pressure of 2 atm (Fig. 2a and Table 1). When comparing pure- and mixed-gas tests performed at similar CO2 partial pressure of 1.2 atm, all polymers, with the exception of PIM-tBOC, display an increase in CO2/CH4 permselectivity and minimal changes in CO2 permeability. PIM-NH2 (290 d) showed an outstanding 140% increase in permselectivity from 12 in pure-gas tests to 29 in mixed-gas tests. Moreover, pure-gas permselectivities for PIM-NH2 (290 d) differed between fresh (3.6) and aged (12) films, showing the effect of aging on permselectivity of these films. In mixed-gas conditions, all samples containing amine functionality (PIM-NH2, PIM-deBOC(acid), and PIM-deBOC(thermal)) exhibited nearly identical permselectivities between 25 and 29, suggesting that mixed-gas transport is primarily controlled by competition as opposed to diffusion. However, when comparing the change in selectivity between pure-gas calculations and mixed-gas experiments, PIM-deBOC(acid) and PIM-deBOC(thermal) exhibited a smaller increase in permselectivity of 85% and 34%, respectively. Interestingly, there are significant deviations between time-lag and equilibrium sorption analysis, particularly for PIM-NH2.48 These differences are associated with non-linearities in the concentration gradient through the polymer film.44,46,67 Thus, the smaller increase in mixed-gas permselectivity for PIM-deBOC(thermal) versus PIM-NH2 is attributed to its lower Langmuir affinity for CO2, which is further discussed later. When considering other derivatives, small variations were observed between pure- and mixed-gas CO2/CH4 permselectivities for aged, untreated PIM-1 (+18%) and PIM-COOH (+26%), and aged, methanol treated PIM-1 (+16%) and PIM-tBOC (−6%). In other studies, small but significant differences in CO2/CH4 mixed- and pure-gas permselectivities have been documented for glassy polymers including the 6FDA-HAB polyimide (+25%), TDA1-APAF (+7%), and thermally rearranged PIM-6FDA-OH (+15%), where permselectivity increases for the mixed-gas case were also attributed to competition.35,50,68
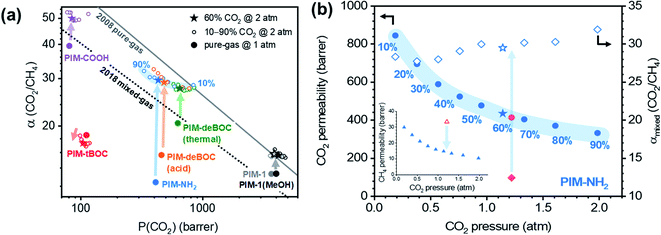 |
| Fig. 2 (a) Robeson plot for aged PIMs containing 2008 pure-gas and 2018 mixed-gas upper-bound relationships. Pure-gas tests were performed at 35 °C and 1.2 atm (filled circles) and mixed-gas tests at 35 °C and approximately 2 atm at nine compositions (open circles). Stars denote mixed-gas tests performed with a 60/40 CO2/CH4 composition and a CO2 partial pressure similar to that of pure-gas. (b) CO2 mixed-gas permeabilities (blue filled circles) and CO2/CH4 permselectivities (open diamonds) for incremental CO2 compositions at a total pressure of approximately 2 atm for PIM-NH2. Inset corresponds to mixed-gas CH4 permeabilities (filled triangles). Pink points indicate pure-gas CO2 (circle) and CH4 (open triangle) permeabilities, and pure-gas CO2/CH4 permselectivity (diamond). Stars denote permselectivity (open) and CO2 permeability (filled) tested at a 60/40 CO2/CH4 composition. For PIM-NH2(290 d) tested at 10–80% CO2 compositions, the total mixed-gas pressure was 1.9 atm. At a 90% CO2 composition, the total mixed-gas pressure was set to 2.2 atm to maintain a high flux and a low stage cut. | |
Table 1 Pure- and mixed-gas permeabilities and permselectivities tested at 1.2 atm partial pressure of CO2 and 35 °C. All uncertainties were calculated from error propagation and each test was performed on independently cast polymer films
Polymer sample designation |
Aging time (days) |
Treatment after castingb |
Permeability (barrer) |
Selectivity (α) |

|

|

|

|

|

|
Mixed-gas tests were performed at a 1.6 atm partial pressure of CO2.
Treatment designations: MeOH (MeOH soak for 24 h followed by a vacuum dry at 130 °C for 12 h), vacuum dry (dry at 130 °C for 12 h), and CO2 conditioning (pure-gas CO2 test up to 29 atm). Sample thicknesses and treatments are summarized in Table S1.
Differences in PIM-NH2 aging rate reflects the typical variation in permeation properties for two microporous films casted independently.
|
PIM-1 (fresh) |
1 |
MeOH |
9000 ± 350 |
8250 ± 350 |
660 ± 25 |
810 ± 50 |
13.5 ± 0.8 |
10.2 ± 0.8 |
PIM-1 (381 d) |
381 |
MeOH |
4000 ± 200 |
4200 ± 200 |
300 ± 20 |
270 ± 10 |
13.4 ± 0.9 |
16 ± 1 |
PIM-NH2 (fresh)a |
1 |
MeOH |
1070 ± 60 |
845 ± 50 |
300 ± 15 |
33.5 ± 5.0 |
3.6 ± 0.3 |
25.2 ± 3.9 |
PIM-NH2 (290 d)c |
290 |
MeOH |
410 ± 20 |
430 ± 20 |
33 ± 2 |
14.7 ± 0.8 |
12 ± 1 |
29 ± 2 |
PIM-NH2 (448 d)c |
448 |
MeOH |
480 ± 20 |
509 ± 25 |
44 ± 2 |
18.3 ± 1.4 |
11.1 ± 0.7 |
27.8 ± 2.5 |
PIM-NH2 (cond.) |
433 |
MeOH and CO2 conditioning |
410 ± 20 |
445 ± 25 |
54 ± 3 |
16.9 ± 1.5 |
7.6 ± 0.6 |
26.3 ± 2.8 |
PIM-tBOC |
351 |
MeOH |
110 ± 20 |
100 ± 10 |
6.1 ± 0.8 |
6.1 ± 0.8 |
18 ± 3 |
17 ± 3 |
PIM-deBOC(acid) |
339 |
MeOH |
460 ± 20 |
480 ± 20 |
30 ± 1 |
16.7 ± 0.6 |
15.6 ± 0.9 |
29 ± 2 |
PIM-deBOC(thermal) |
343 |
MeOH |
630 ± 30 |
650 ± 30 |
31 ± 2 |
24 ± 1 |
20 ± 1 |
28 ± 2 |
PIM-1 (402 d) |
402 |
Vacuum dry |
3700 ± 100 |
3900 ± 100 |
280 ± 10 |
250 ± 10 |
13.3 ± 0.7 |
15.8 ± 0.9 |
PIM-COOH (330 d) |
330 |
Vacuum dry |
81 ± 4 |
90 ± 20 |
2.1 ± 0.1 |
1.7 ± 0.1 |
39 ± 3 |
50 ± 4 |
To assess the influence of changes in CO2 content on mixed-gas permeation, incremental CO2/CH4 mixture compositions were investigated at a total pressure of 2 atm (Fig. 2 and S2†). CO2 and CH4 permeabilities decreased with increasing CO2 composition from 10% to 90% (Fig. 2b). This trend is consistent with the decrease in sorption expected for glassy polymers with increasing pressure in the framework of the dual-mode sorption model when no significant plasticization-induced variations in diffusion are expected.35 As pressure increases and Langmuir modes saturate, the sorption coefficient, and thus permeability, decreases due to the higher energetic penalty required to sorb into the Henry's law mode.69,70 The CO2/CH4 mixed-gas permselectivity slightly increased with increasing content of CO2, demonstrating how competition (i.e., CH4 exclusion from the polymer matrix) is proportional to the molar ratio of CO2 over CH4 in the mixture. When comparing pure- and mixed-gas tests at the same CO2 pressure, CO2 permeabilities were roughly equivalent to each other. Conversely, mixed-gas CH4 permeability was significantly lower compared to pure-gas permeabilities (cf., inset in Fig. 2b and Table 1), due to competitive sorption and exclusion of CH4. As presented in Fig. S2,† these trends applied to every aged PIM considered except PIM-tBOC, which was the polymer with the lowest CO2 infinite dilution sorption coefficient among those tested (Fig. 1). Taken together, the decrease in CH4 permeability from pure- to mixed-gas tests at the same partial pressure of CH4 correlated directly with the CO2 affinity of the polymer, as shown in Fig. S3.†
The shape of the isobaric mixed-gas permeability trends also provides valuable insights. By increasing CO2 compositions from 10% to 90%, CO2 permeabilities decreased by 60% for PIM-NH2 (290 d) and 50% for PIM-deBOC(acid) and the magnitude of the decreases correlated well with their Langmuir affinity constants of 1.006 atm−1 and 0.895 atm−1, respectively (Table S3†). In contrast, PIM-tBOC showed a small change in permeability over the same range of CO2 compositions from 118 to 99 barrer, which was ascribed to a low b value of 0.427 atm−1. In each case, tests at incremental CO2 compositions illustrate the important role of sorption on transport at varying feed conditions. At CO2 compositions of 10%, for instance, PIM-NH2 has competitive performance above the 2008 upper bound, indicating its potential for low-pressure and low concentration CO2 removal applications, such as those found in biogas purification.71
Other mixtures.
Binary H2/N2, H2/CH4, and CO2/N2 mixtures were also investigated due to their relevance in commercial and emerging membrane-based applications including hydrogen purification and post-combustion carbon capture.72 For these separations, significant competitive sorption effects were also observed in PIM-NH2 (Fig. S4†) and aligned directly with critical temperatures of the gases, summarized in Table S2.† Notably, CO2/N2 mixed-gas permselectivity increased by 3.5 times (+250%) that of the pure-gas performance, higher than the enhancement measured for CO2/CH4. This result is consistent with the rationale that the driving force for competition is the relative difference in sorption of two gases for a binary mixture. Critical temperature is often used as a correlating variable for sorption,73 and indeed Tc(N2) < Tc(CH4) ≪ Tc(CO2). Thus, increases in CO2/N2 permselectivity are expected to exceed those for CO2/CH4. Enhancements in CO2/N2 permselectivity have also been reported for other PIMs, including TZ-PIM38 (+47%) and MTZ-PIM74 (+80%), but to a lesser extent than that for PIM-NH2. Similar considerations apply for H2-based separations, which showed a reduction in H2/CH4 and H2/N2 permselectivity for PIM-NH2, consistent with the stronger sorption preference of CH4 and N2 compared to H2.
Pure-gas sorption tests and mixed-gas sorption predictions
Pure-gas sorption isotherms for methanol treated PIM-1 and PIM-NH2 derivatives are presented in Fig. 3a and S5,† along with previously published sorption isotherms for untreated PIM-1 and PIM-COOH.40 As shown in Fig. S5a and b,† the relative order in CO2 uptake observed for each film at 1 atm (PIM-tBOC ≪ PIM-1 < PIM-deBOC(thermal) < PIM-deBOC(acid) ≈ PIM-NH2) correlated remarkably well with the relative increase in CO2/CH4 mixed-gas permselectivity for each PIM. In contrast, O2, CH4, and N2 isotherms show a different trend in relative uptake with PIM-tBOC ≪ PIM-deBOC(acid) ≈ PIM-NH2 < PIM-1 ≈ PIM-deBOC(thermal). To provide deeper physical insight into the distinct CO2 sorption behavior compared to that of other gases, the pure-gas sorption at infinite dilution (S∞) was calculated. This material property, S∞, describes sorption of the first gas molecule into the polymer matrix and is dependent on temperature.40,69 By taking the limit of eqn (5) as fugacity approaches zero, S∞ is defined as: | 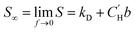 | (9) |
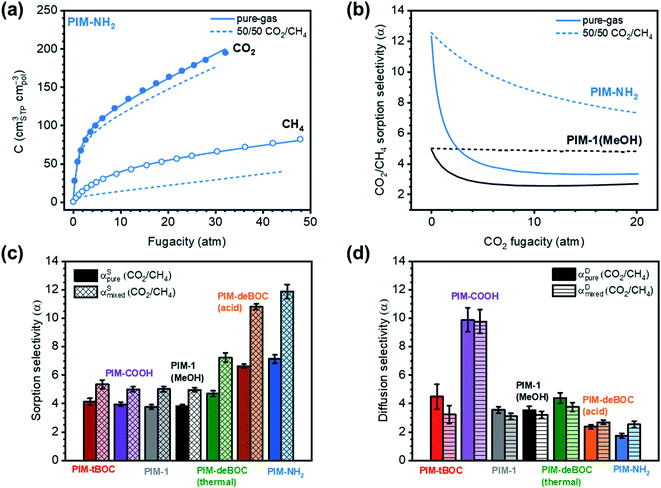 |
| Fig. 3 (a) Pure-gas CO2 and CH4 sorption isotherms (circles) fitted with the DMS model (solid lines), and CO2 and CH4 50/50 mixed-gas sorption isotherms predicted with the DMS model (dashed lines) for PIM-NH2. Open (CH4) and filled (CO2) symbols denote experimentally collected data. (b) Pure-gas CO2 and CH4 sorption selectivities (solid lines) and predicted 50/50 CO2/CH4 sorption selectivities (dashed lines) for PIM-NH2. (c) Pure- and predicted mixed-gas sorption selectivities at a CO2 partial fugacity of 1 atm. (d) Pure- and mixed-gas diffusion selectivities calculated using the sorption–diffusion model at a CO2 partial fugacity of 1 atm. | |
DMS parameters and S∞ values for all isotherms, including untreated PIM-COOH and PIM-1
40 are summarized in Table S3.† In line with the aforementioned sorption trends, the relative order of magnitude for S∞ and b for CO2 (Fig. 1 and Table S3†) correlates with the relative increase in permeability between pure-gas and mixed-gas measurements in Fig. 2a. At the same time, the relative order of S∞ for O2, N2, and CH4, aligns with the respective uptake trends of these gases, indicating a strong chemical interaction for PIM-NH2 and CO2 that is not observed for gases with lower critical temperatures.
The effect of competitive sorption was modeled from pure-gas isotherms using eqn (6), as presented in Fig. 3a and S6.† This modeling approach has demonstrated good agreement with experimental mixed-gas sorption for PIM-1, TZ-PIM, PTMSP, 6FDA-HAB and its TR450 analogue, 6FDA-mPDA, CTA, and PMMA.19,21,23,24,29,51,75 Thermodynamically rigorous models such as the nonequilibrium lattice fluid (NELF)76 model would provide more robust mixed-gas predictions,23,77 but lattice fluid parameters (i.e., ρ*, T*, p*) cannot be directly determined for the PIMs studied in this work because the polymers degrade below their glass transition temperature.78,79 As shown in Fig. S6a,† experimental pure-gas and predicted 50/50 CO2/CH4 mixed-gas PIM-1 sorption isotherms obtained in this work aligned very well with experimental results reported by Vopička et al.80 The modeling approach was thus extended to all six PIMs, as presented in Fig. S6 and S7.† Predicted mixed-gas sorption isotherms showed a small decrease in CO2 uptake ranging between −5% and −9% for all films, while CH4 isotherms displayed a much more pronounced decrease ranging from −29% to −47%. Because of its high solubility, CO2 sorption is weakly affected by the presence of CH4 in the mixture while CH4 experiences a significant reduction in sorption, resulting in a beneficial increase in CO2/CH4 sorption selectivity (Fig. S7b and c†). The effects of competition for increasing fugacities can be visualized by plotting the pure- and mixed-gas CO2/CH4 sorption selectivities (αSpure and αSmixed) (Fig. 3b and S7†). As shown in Fig. 3b, αSmixed for PIM-1 and PIM-NH2 is consistently higher than αSpure for all fugacities considered.
Diffusion selectivity was also evaluated. To this end, pure- and mixed-gas diffusion selectivities (αDpure and αDmixed) were estimated via the sorption–diffusion model (eqn (7)) using experimental pure-gas and modeled mixed-gas sorption coefficients for a CO2 partial fugacity of 1 atm. As shown in Fig. 3d and Table S4,† there is little change between the αDpure and αDmixed for each sample, reinforcing our assertion that the increase in mixed-gas permselectivity is driven by competition.
Pure-gas and mixed-gas plasticization
Penetrant-induced plasticization is a significant challenge for the deployment of membranes for natural gas purification.81 At industrially relevant pressures, penetrants like CO2 can swell the polymer and result in detrimental losses in selectivity. Effective methods to mitigate plasticization involve increasing interchain rigidity with addition of strong secondary interactions,8,82–84 thermal annealing,85–87 or chemical crosslinking.88,89 Here, we investigate the influence of amine hydrogen bonding and –tBOC functionality on CO2-induced plasticization of PIM-NH2 derivatives. As shown in Fig. 4a, PIM-1 showed a pure-gas plasticization pressure at a fugacity of 14 atm while PIM-NH2, PIM-deBOC(acid), and PIM-deBOC(thermal) showed no plasticization pressure up to a feed fugacity of 29 atm. The shape of the high-pressure curves for the PIM-NH2 derivatives; in particular, the significant drop in permeability at low fugacity, is associated with the strong contribution to Langmuir mode sorption, as can be gleaned from Fig. 3a and S6.† In contrast, PIM-tBOC exhibited a plasticization pressure at a fugacity of 10 atm, which was ascribed to weaker interchain interactions between –tBOC groups compared to dipolar –CN groups and hydrogen bonding –NH2 groups.
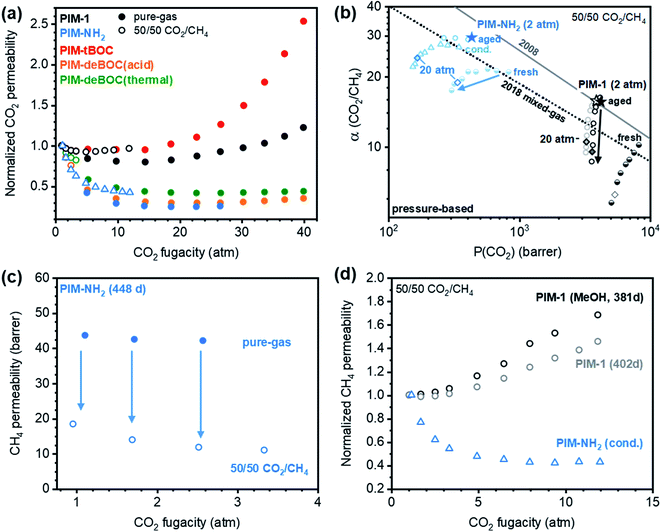 |
| Fig. 4 (a) Pure- (filled circles) and mixed-gas (open shapes) normalized CO2 permeabilities for PIM films. (b) Mixed-gas CO2/CH4 upper-bound and plasticization performance for conditioned PIM-NH2 (open blue triangles), fresh PIM-NH2 (half-filled blue circles), aged PIM-1 films which underwent methanol treatment (381 d, open black circles) or no methanol treatment (402 d, open gray circles), and a freshly methanol-treated PIM-1 film (half-filled black circles). Mixed-gas tests performed with a 60/40 CO2/CH4 mixture at 2 atm total pressure are denoted as stars. High-pressure mixed-gas points collected at a total pressure of 20 atm (e.g., 10 atm of CO2) are highlighted as diamonds for comparison with the pressure-based 2018 mixed-gas upper bound. Permeability data in this plot was specifically calculated using pressure for ease of comparison with literature. (c) Pure- and mixed-gas CH4 permeability for aged PIM-NH2 (448 d) up to a total pressure of 7 atm. (d) Normalized mixed-gas CH4 permeability versus CO2 partial pressure for methanol treated PIM-1 (open black circles), untreated PIM-1 (open gray circles), and conditioned PIM-NH2 (open blue triangles). Reference data for PIM-NH2(fresh) is provided in Fig. S10.† | |
PIM-NH2 was further evaluated for plasticization stability using a 50/50 CO2/CH4 mixture up to total feed fugacity of 24 atm (Fig. 4). Mixed-gas tests up to total fugacity of 5 atm were also provided for the other derivatives in Fig. S8 and S9.† For all PIM-NH2 films, the CO2/CH4 mixed-gas permselectivity increased slightly up to a total fugacity of 5 atm as shown in Fig. 4b and S9a.† This unusual finding relates to a favorable combination of CO2/CH4 competitive effects and high CO2–polymer affinity. Pure-gas tests up to 2.5 atm at similar partial fugacity were also compared to the mixed-gas plasticization curves in Fig. 4c, S10 and S11.† In each case, CO2 permeability is essentially the same in pure- and mixed-gas scenarios, while CH4 mixed-gas permeabilities decrease significantly compared to pure-gas values, a clear demonstration of competitive sorption in CO2-containing mixtures. CO2/CH4 mixed-gas plasticization was further evaluated by inspecting the co-permeability of CH4 with the mixture, an unambiguous indicator of plasticization.34 Since CH4 is not a typical plasticizing gas, its pure-gas permeability is only expected to decrease with increasing fugacity. However, when co-permeating with CO2, CH4 diffusion is expected to increase more readily by CO2-induced plasticization, resulting in an accentuated plasticization pressure curve for CH4.23 As presented in Fig. 4d, S10 and S11,† PIM-1 shows an immediate up-turn in CH4 permeability with increasing fugacity, while PIM-NH2 retains stable CH4 permeability that follows trends expected for non-plasticized glassy polymers up to a total feed fugacity of 24 atm. Moreover, by increasing CO2 partial pressure from 2 atm to 10 atm, there is a decrease in mixed-gas selectivity for both treated (−36%) and untreated (−32%) PIM-1 that is significantly more pronounced than that of PIM-NH2 (−10%), as shown on the Robeson plot in Fig. 4b.
Comparisons to other microporous polymers
Mixed-gas analysis at low pressures demonstrated how functionality influences competitive sorption for PIMs. To contextualize these findings, a literature survey of CO2/CH4 pure- and mixed-gas data for microporous polymers is summarized in Table S5† and Fig. 5. For the comparison shown in Fig. 5a, only literature studies reporting (1) pure-gas permeability measured at a pressure consistent with the CO2 partial pressure reported for the mixed-gas test, and (2) CO2 and CH4 high-pressure sorption isotherms were included. The ratio of CO2/CH4 mixed- to pure-gas permselectivity (αmixed/αpure) was plotted against αSpure, where αDpure and αDmixed were assumed invariant at low CO2 partial pressures, consistent with our findings presented in Fig. 3d. As a general trend, as αSpure increases, αmixed/αpure increases largely due to competitive sorption effects. Similarly, at a total pressure of 2 atm, the six PIM derivatives show an increasing trend, where amine-functionalized PIM-NH2 and its chemical analogue, PIM-deBOC(acid), show the largest increase in αmixed/αpure and the highest αSpure. The chemical structures of polymers showcased in Fig. 5a are shown in Fig. S12† in order of increasing αSpure values. Among the samples considered, backbones containing –OH34,83 and –COOH90,91 groups show generally higher CO2/CH4αSpure values than backbones containing groups such as –F, –H, or –CN.17,22,34,91–96 Moreover, all polymers considered from the literature have αSpure below 5.5 and a αmixed/αpure less than 1.5. In contrast, PIM-NH2 (2.4) and PIM-deBOC(acid) (1.9) showed the highest improvements to CO2/CH4αmixed (Fig. 5a). To the best of our knowledge, these improvements represent a record for microporous polymer membranes, highlighting the intriguing benefits of using amine-functionalized microporous polymers for CO2-based separations. Other amine-containing PIMs such as TZ-PIM and AO-PIM have shown less pronounced changes in sorption selectivity, as presented in the sorption upper bound in Fig. S13.† These trends correlate with lower CO2 Langmuir affinities for TZ-PIM-1 (b = 0.437 atm−1) and AO-PIM-1 (b = 0.324 atm−1) than PIM-NH2 (b = 1.006 atm−1).20 In these cases, we hypothesize that the lower CO2–polymer affinity may be associated with the nature of the acid–base interactions for each functional group as well as group accessibility. For instance, the amine in AO-PIM is accompanied by an –OH group that can hydrogen bond while TZ-PIM has low pKa values compared to primary amines38,97 and an aromatic ring that can create steric hindrance.
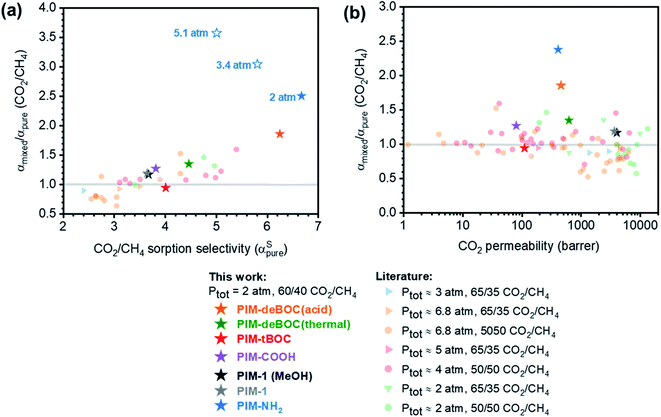 |
| Fig. 5 Ratio of mixed-gas and pure-gas CO2/CH4 permselectivity (αmixed/αpure) versus (a) pure-gas CO2/CH4 sorption selectivity (αpure) for aged PIMs and literature samples. All filled stars indicate tests at a mixed-gas total pressure of 2 atm. Open blue stars indicate mixed-gas tests performed on PIM-NH2 (448 d) at CO2 total pressures of 3.4 atm and 5.1 atm. (b) CO2 pure-gas permeability for this work (star symbols) and literature data (other symbols) for microporous polymers tested at approximate total pressures indicated in the legend. Detailed references, permeation data, and exact testing pressures are reported in Table S5.† | |
Compared to other PIM-1 analogues, the CO2/CH4 pure- and mixed-gas sorption selectivities for PIM-NH2 are remarkably high. When considering sorption at infinite dilution, PIM-NH2 falls on the infinite-dilution sorption upper bound (Fig. S13†), which was developed theoretically in 2014 by Lipscomb et al. for pure gases (i.e., excluding competitive sorption effects). As indicated by the authors, this upper bound is limited by the highest value of cohesive energy achievable in polymers, indicating that PIM-NH2 sorption values fall close to this thermodynamic limit, enabling the remarkable increase in CO2/CH4 and CO2/N2 mixed-gas permselectivity compared to pure-gas results. These results suggest a unique interaction between the Lewis basic –NH2 and CO2, consistent with the outstanding CO2 adsorption behavior reported for amine-functionalized MOFs and amine-functionalized polysulfone.37,98–101
Fig. 5b presents a larger collection of αmixed/αpure data reported for microporous polymers against pure-gas CO2 permeability, including studies that did not test sorption. For polymers reported in the literature, the αmixed/αpure permselectivity ratio centers around 1 ± 0.2 with the lowest and highest literature values of 0.52 and 1.59 for PIM-EA(Me2)-TB102 and 6FDA-DAM
:
DABA (1
:
2),90 respectively. Remarkably, the value of αmixed/αpure for PIM-NH2 (290 d and 448 d) is 2.4, surpassing all other microporous polymers considered in the literature. Interestingly, as total pressure rises, αmixed/αpure for PIM-NH2 further increases to over 3.5 at a pressure of 5.1 atm (Fig. 5a). These results demonstrate the promise of –NH2 chemistry for enhancing CO2-based mixed-gas transport through competition, while simultaneously providing plasticization resistance through hydrogen bonding.
Conclusion
Mixed-gas transport properties of six functionalized PIM-1 variants were systematically investigated after aging to study the role of CO2 affinity and polymer chemistry on competitive sorption. Low-pressure mixed-gas tests revealed a relationship between CO2 sorption affinity and changes in permselectivities of mixed-gas tests compared to pure-gas tests. Amine-functionalized PIM-1 showed a 140% increase in mixed-gas CO2/CH4 selectivity and a 250% increase in CO2/N2 mixed-gas selectivity compared to pure-gas results performed at the same partial pressure of CO2. Mixed-gas sorption predictions and sorption–diffusion model analysis indicated that CO2/CH4 permselectivity improvements resulted from sorption selectivity increases that are exclusive to mixed-gas conditions. Structure–property relationships were derived by comparing pure-gas sorption and mixed-gas permeation performance for samples in this study and for microporous polymers in the literature. PIM-NH2 showed the highest CO2/CH4 sorption selectivity for all polymers considered, and correspondingly, the greatest improvement for mixed-gas tests relative to pure-gas data. Microporous polymers containing hydroxyl and carboxylic acid functionalized backbones showed more limited improvements, and PIMs without hydrogen bonding moieties showed the most limited improvements. Notably, PIM-NH2 and its chemical analogue (PIM-deBOC(acid)) approached the theoretical sorption limit predicted in the 2014 pure-gas sorption upper bound. Finally, high-pressure 50/50 CO2/CH4 mixed-gas tests revealed exceptional plasticization resistance for PIM-NH2. This effect was attributed to enhanced interchain rigidity induced by hydrogen bonding in PIM-NH2. Our results demonstrate that increasing solubility–selectivity is of paramount importance to leverage competitive sorption and improve permselectivity for CO2-based gas pairs. Furthermore, plasticization resistance is necessary to retain this advantage at high pressures. Among the six PIMs considered, the primary amine functionalization (PIM-NH2) was identified as the best compromise of these two features, displaying attractive performance under testing conditions reminiscent to those found in industry.
Author contributions
K. Mizrahi Rodriguez: conceptualization, formal analysis, methodology, investigation, data curation, visualization, writing – original draft. F. M. Benedetti: conceptualization, formal analysis, methodology, investigation, writing – review & editing. N. Roy: investigation, data curation, writing – review & editing. A. X. Wu: writing – review & editing. Z. P. Smith: supervision, writing – review & editing.
Conflicts of interest
There are no conflicts to declare.
Acknowledgements
This work was supported by the U.S. Department of Energy, Office of Science, Office of Basic Energy Sciences, Separation Science program under Award Number DE-SC0019087. Additionally, support to investigate low-pressure competitive sorption was provided by the Office of Naval Research (ONR) under Award Number N00014-21-1-2666 and N00014-20-1-2418. F. M. Benedetti and K. Mizrahi Rodriguez acknowledge support from the Exxon Mobil Research and Engineering Company through the MIT Energy Initiative. K. Mizrahi Rodriguez and A. X. Wu were supported by NSF-GRFP fellowships (DGE-1745302 and DGE-1122374, respectively). The authors would like to thank Taigyu Joo and Wan-Ni Wu for providing the fresh PIM-NH2 and PIM-1 films used in this work, respectively. The authors also gratefully acknowledge Sharon Lin, Kayla R. Storme, Aristotle Grosz, and Duha Syar, for helping with the collection of pure- and mixed-gas literature data for microporous polymers, which is considered in the comparisons performed in this work.
References
-
U.S. EIA, U.S. Natural Gas Total Consumption (Million Cubic Feet), http://www.eia.gov/dnav/ng/hist/n9140us2a.htm, accessed 12 April 2021 Search PubMed.
- D. S. Sholl and R. P. Lively, Nature, 2016, 532, 435–437 Search PubMed.
-
DOE-EPSA, Energy CO2 Emissions Impacts of Clean Energy Technology Innovation and Policy, 2017, p. 43 Search PubMed.
- R. W. Baker, Ind. Eng. Chem. Res., 2002, 41, 1393–1411 Search PubMed.
- L. M. Robeson, J. Membr. Sci., 1991, 62, 165–185 Search PubMed.
- N. B. Mc Keown and P. M. Budd, Chem. Soc. Rev., 2006, 35, 675–683 Search PubMed.
- H. B. Park, C. H. Jung, Y. M. Lee, A. J. Hill, S. J. Pas, S. T. Mudie, E. Van Wagner, B. D. Freeman and D. J. Cookson, Science, 2007, 318, 254–258 Search PubMed.
- Y. He, F. M. Benedetti, S. Lin, C. Liu, Y. Zhao, H. Z. Ye, T. Van Voorhis, M. G. De Angelis, T. M. Swager and Z. P. Smith, Adv. Mater., 2019, 31, 1807871 Search PubMed.
- H. W. H. Lai, Y. C. Teo and Y. Xia, ACS Macro Lett., 2017, 6, 1357–1361 Search PubMed.
- B. Comesaña-Gándara, J. Chen, C. G. Bezzu, M. Carta, I. Rose, M. C. Ferrari, E. Esposito, A. Fuoco, J. C. Jansen and N. B. McKeown, Energy Environ. Sci., 2019, 12, 2733–2740 Search PubMed.
- R. Swaidan, B. Ghanem and I. Pinnau, ACS Macro Lett., 2015, 4, 947–951 Search PubMed.
- Y. Wang, X. Ma, B. S. Ghanem, F. Alghunaimi, I. Pinnau and Y. Han, Mater. Today Nano, 2018, 3, 69–95 Search PubMed.
- P. M. Budd, N. B. McKeown and D. Fritsch, J. Mater. Chem., 2005, 15, 1977–1986 Search PubMed.
- P. M. Budd, S. M. Makhseed, B. S. Ghanem, K. J. Msayib, C. E. Tattershall and N. B. McKeown, Mater. Today, 2004, 7, 40–46 Search PubMed.
- T. Corrado and R. Guo, Mol. Syst. Des. Eng., 2020, 5, 22–48 Search PubMed.
- S. Luo, Q. Zhang, L. Zhu, H. Lin, B. A. Kazanowska, C. M. Doherty, A. J. Hill, P. Gao and R. Guo, Chem. Mater., 2018, 30, 5322–5332 Search PubMed.
- R. Swaidan, B. Ghanem, M. Al-Saeedi, E. Litwiller and I. Pinnau, Macromolecules, 2014, 47, 7453–7462 Search PubMed.
- R. Swaidan, M. Al-Saeedi, B. Ghanem, E. Litwiller and I. Pinnau, Macromolecules, 2014, 47, 5104–5114 Search PubMed.
- E. Ricci, A. E. Gemeda, N. Du, N. Li, M. G. De Angelis, M. D. Guiver and G. C. Sarti, J. Membr. Sci., 2019, 585, 136–149 Search PubMed.
-
A. E. Gemeda, Solubility, diffusivity and permeability of gases in glassy polymers, in Ingegneria chimica dell'ambiente e della sicurezza, Alma Mater Studiorum-Università di Bologna, AMS Dottorato, 2015 Search PubMed.
- G. Genduso, B. S. Ghanem and I. Pinnau, Membranes, 2019, 9, 10 Search PubMed.
- G. Genduso, Y. Wang, B. S. Ghanem and I. Pinnau, J. Membr. Sci., 2019, 584, 100–109 Search PubMed.
- E. Ricci, F. M. Benedetti, M. E. Dose, M. G. De Angelis, B. D. Freeman and D. R. Paul, J. Membr. Sci., 2020, 612, 118374 Search PubMed.
- G. Genduso and I. Pinnau, J. Membr. Sci., 2020, 610, 118269 Search PubMed.
- R. D. Raharjo, B. D. Freeman, D. R. Paul, G. C. Sarti and E. S. Sanders, J. Membr. Sci., 2007, 306, 75–92 Search PubMed.
- G. Genduso, E. Litwiller, X. Ma, S. Zampini and I. Pinnau, J. Membr. Sci., 2019, 577, 195–204 Search PubMed.
- H. D. Kamaruddin and W. J. Koros, J. Membr. Sci., 1997, 135, 147–159 Search PubMed.
- C. P. Ribeiro and B. D. Freeman, Polymer, 2010, 51, 1156–1168 Search PubMed.
- E. S. Sanders, W. J. Koros, H. B. Hopfenberg and V. T. Stannett, J. Membr. Sci., 1984, 18, 53–74 Search PubMed.
- E. S. Sanders and W. J. Koros, J. Polym. Sci., Part B: Polym. Phys., 1986, 24, 175–188 Search PubMed.
- E. Ricci, F. M. Benedetti, A. Noto, T. C. Merkel, J. Jin and M. Grazia De Angelis, Chem. Eng. J., 2021, 130715 Search PubMed.
- Y. Liu, Z. Chen, W. Qiu, G. Liu, M. Eddaoudi and W. J. Koros, J. Membr. Sci., 2021, 627, 119201 Search PubMed.
- Y. Liu, G. Liu, C. Zhang, W. Qiu, S. Yi, V. Chernikova, Z. Chen, Y. Belmabkhout, O. Shekhah, M. Eddaoudi and W. Koros, Adv. Sci., 2018, 5, 1800982 Search PubMed.
- R. Swaidan, B. Ghanem, E. Litwiller and I. Pinnau, J. Membr. Sci., 2015, 475, 571–581 Search PubMed.
- F. Alghunaimi, B. Ghanem, N. Alaslai, M. Mukaddam and I. Pinnau, J. Membr. Sci., 2016, 520, 240–246 Search PubMed.
- M. L. Jue and R. P. Lively, React. Funct. Polym., 2015, 86, 88–110 Search PubMed.
- K. Ghosal, R. T. Chern, B. D. Freeman, W. H. Daly and I. I. Negulescu, Macromolecules, 1996, 29, 4360–4369 Search PubMed.
- N. Du, H. B. Park, G. P. Robertson, M. M. Dal-Cin, T. Visser, L. Scoles and M. D. Guiver, Nat. Mater., 2011, 10, 372–375 Search PubMed.
- X. Weng, J. E. Baez, M. Khiterer, M. Y. Hoe, Z. Bao and K. J. Shea, Angew. Chem., 2015, 54, 11214–11218 Search PubMed.
- K. Mizrahi Rodriguez, A. X. Wu, Q. Qian, G. Han, S. Lin, F. M. Benedetti, H. Lee, W. S. Chi, C. M. Doherty and Z. P. Smith, Macromolecules, 2020, 53, 6220–6234 Search PubMed.
- N. Du, G. P. Robertson, J. Song, I. Pinnau and M. D. Guiver, Macromolecules, 2009, 42, 6038–6043 Search PubMed.
- J. W. Jeon, D. G. Kim, E. H. Sohn, Y. Yoo, Y. S. Kim, B. G. Kim and J. C. Lee, Macromolecules, 2017, 50, 8019–8027 Search PubMed.
- C. R. Mason, L. Maynard-Atem, N. M. Al-Harbi, P. M. Budd, P. Bernardo, F. Bazzarelli, G. Clarizia and J. C. Jansen, Macromolecules, 2011, 44, 6471–6479 Search PubMed.
- C. R. Mason, L. Maynard-Atem, K. W. J. Heard, B. Satilmis, P. M. Budd, K. Friess, M. Lancì, P. Bernardo, G. Clarizia and J. C. Jansen, Macromolecules, 2014, 47, 1021–1029 Search PubMed.
- W.-H. Wu, P. Thomas, P. Hume and J. Jin, Membranes, 2018, 8, 20 Search PubMed.
- B. Satilmis, M. Lanč, A. Fuoco, C. Rizzuto, E. Tocci, P. Bernardo, G. Clarizia, E. Esposito, M. Monteleone, M. Dendisová, K. Friess, P. M. Budd and J. C. Jansen, J. Membr. Sci., 2018, 555, 483–496 Search PubMed.
- L. M. Robeson, J. Membr. Sci., 2008, 320, 390–400 Search PubMed.
- K. Mizrahi Rodriguez, S. Lin, A. X. Wu, G. Han, J. J. Teesdale, C. M. Doherty and Z. P. Smith, Angew. Chem., 2021, 133, 6667–6673 Search PubMed.
- R. Swaidan, B. Ghanem, E. Litwiller and I. Pinnau, Macromolecules, 2015, 48, 6553–6561 Search PubMed.
- K. L. Gleason, Z. P. Smith, Q. Liu, D. R. Paul and B. D. Freeman, J. Membr. Sci., 2015, 475, 204–214 Search PubMed.
- E. Ricci and M. G. De Angelis, Membranes, 2019, 9, 8 Search PubMed.
- S. Wang, N. L. Pomerantz, Z. Dai, W. Xie, E. E. Anderson, T. Miller, S. A. Khan and G. N. Parsons, Mater. Today Adv., 2020, 8, 100085 Search PubMed.
- G. H. Fredrickson and E. Helfand, Macromolecules, 1985, 18, 2201–2207 Search PubMed.
- D. R. Paul, J. Polym. Sci., Part A-2: Polym. Phys., 1969, 7, 1811–1818 Search PubMed.
-
S. Matteucci, Y. Yampolskii, B. D. Freeman and I. Pinnau, Transport of gases and vapors in glassy and rubbery polymers, in Materials Science of Membranes for Gas and Vapor Separation, ed. Y. Yampolskii, I. Pinnau, B. Freeman, 2006, pp. 1–47 Search PubMed.
- H. W. H. Lai, F. M. Benedetti, Z. Jin, Y. C. Teo, A. X. Wu, M. G. De Angelis, Z. P. Smith and Y. Xia, Macromolecules, 2019, 52, 6294–6302 Search PubMed.
- Z. P. Smith, D. F. Sanders, C. P. Ribeiro, R. Guo, B. D. Freeman, D. R. Paul, J. E. McGrath and S. Swinnea, J. Membr. Sci., 2012, 415–416, 558–567 Search PubMed.
- W. J. Koros, J. Polym. Sci., Part A-2: Polym. Phys., 1980, 18, 981–992 Search PubMed.
- W. J. Koros, R. T. Chern, V. Stannett and H. B. Hopfenberg, J. Polym. Sci., Part A-2: Polym. Phys., 1981, 19, 1513–1530 Search PubMed.
-
M. D. Koretsky, Engineering and Chemical Thermodynamics, John Wiley & Sons, 2012 Search PubMed.
- J. G. Wijmans and R. W. Baker, J. Membr. Sci., 1995, 107, 1–21 Search PubMed.
- B. D. Freeman, Macromolecules, 1999, 32, 375–380 Search PubMed.
- Z. X. Low, P. M. Budd, N. B. McKeown and D. A. Patterson, Chem. Rev., 2018, 118, 5871–5911 Search PubMed.
- R. R. Tiwari, J. Jin, B. D. Freeman and D. R. Paul, J. Membr. Sci., 2017, 537, 362–371 Search PubMed.
- M. L. Jue, V. Breedveld and R. P. Lively, J. Membr. Sci., 2017, 530, 33–41 Search PubMed.
- Y. Huang and D. R. Paul, J. Polym. Sci., Part B: Polym. Phys., 2007, 45, 1390–1398 Search PubMed.
- M. Lanč, K. Pilnáček, C. R. Mason, P. M. Budd, Y. Rogan, R. Malpass-Evans, M. Carta, B. C. Gándara, N. B. McKeown, J. C. Jansen, O. Vopička and K. Friess, J. Membr. Sci., 2019, 570–571, 522–536 Search PubMed.
- R. Swaidan, X. Ma, E. Litwiller and I. Pinnau, J. Membr. Sci., 2013, 447, 387–394 Search PubMed.
- A. X. Wu, J. A. Drayton, K. Mizrahi Rodriguez, F. M. Benedetti, Q. Qian, S. Lin and Z. P. Smith, Macromolecules, 2021, 54, 22–34 Search PubMed.
- W. J. Koros, D. R. Paul and G. S. Huvard, Polymer, 1979, 20, 956–960 Search PubMed.
- S. Yadvika, T. R. Sreekrishnan, S. Kohli and V. Rana, Bioresour. Technol., 2004, 95, 1–10 Search PubMed.
- M. Galizia, W. S. Chi, Z. P. Smith, T. C. Merkel, R. W. Baker and B. D. Freeman, Macromolecules, 2017, 50, 7809–7843 Search PubMed.
-
Y. Yampolskii, I. Pinnau and B. D. Freeman, Materials Science of Membranes for Gas and Vapor Separation, John Wiley & Sons, Ltd, Chichester, UK, 2006 Search PubMed.
- N. Du, G. P. Robertson, M. M. Dal-Cin, L. Scoles and M. D. Guiver, Polymer, 2012, 53, 4367–4372 Search PubMed.
- V. Loianno, S. Luo, Q. Zhang, R. Guo and M. Galizia, J. Membr. Sci., 2019, 574, 100–111 Search PubMed.
- F. Doghieri and G. C. Sarti, Macromolecules, 1996, 29, 7885–7896 Search PubMed.
- M. Minelli, S. Campagnoli, M. G. De Angelis, F. Doghieri and G. C. Sarti, Macromolecules, 2011, 44, 4852–4862 Search PubMed.
- H. Yin, Y. Z. Chua, B. Yang, C. Schick, W. J. Harrison, P. M. Budd, M. Böhning and A. Schönhals, J. Phys. Chem. Lett., 2018, 9, 2003–2008 Search PubMed.
- H. Yin, B. Yang, Y. Z. Chua, P. Szymoniak, M. Carta, R. Malpass-Evans, N. B. McKeown, W. J. Harrison, P. M. Budd, C. Schick, M. Böhning and A. Schönhals, ACS Macro Lett., 2019, 8, 1022–1028 Search PubMed.
- O. Vopička, M. G. De Angelis, N. Du, N. Li, M. D. Guiver and G. C. Sarti, J. Membr. Sci., 2014, 459, 264–276 Search PubMed.
- R. W. Baker and K. Lokhandwala, Ind. Eng. Chem. Res., 2008, 47, 2109–2121 Search PubMed.
- N. Alaslai, B. Ghanem, F. Alghunaimi, E. Litwiller and I. Pinnau, J. Membr. Sci., 2016, 505, 100–107 Search PubMed.
- X. Ma, R. Swaidan, Y. Belmabkhout, Y. Zhu, E. Litwiller, M. Jouiad, I. Pinnau and Y. Han, Macromolecules, 2012, 45, 3841–3849 Search PubMed.
- R. Swaidan, B. S. Ghanem, E. Litwiller and I. Pinnau, J. Membr. Sci., 2014, 457, 95–102 Search PubMed.
- Z. Tian, B. Cao and P. Li, J. Membr. Sci., 2018, 560, 87–96 Search PubMed.
- A. M. Kratochvil and W. J. Koros, Macromolecules, 2008, 41, 7920–7927 Search PubMed.
- W. Qiu, C. C. Chen, L. Xu, L. Cui, D. R. Paul and W. J. Koros, Macromolecules, 2011, 44, 6046–6056 Search PubMed.
- J. D. Wind, C. Staudt-Bickel, D. R. Paul and W. J. Koros, Ind. Eng. Chem. Res., 2002, 41, 6139–6148 Search PubMed.
- C. Zhang, L. Fu, Z. Tian, B. Cao and P. Li, J. Membr. Sci., 2018, 556, 277–284 Search PubMed.
- Z. Liu, Y. Liu, W. Qiu and W. J. Koros, Angew. Chem., 2020, 59, 14877–14883 Search PubMed.
- M. A. Abdulhamid, G. Genduso, Y. Wang, X. Ma and I. Pinnau, Ind. Eng. Chem. Res., 2020, 59, 5247–5256 Search PubMed.
- J. Wu, J. Liu and T. S. Chung, Adv. Sustainable Syst., 2018, 2, 1800044 Search PubMed.
- A. Fuoco, B. Satilmis, T. Uyar, M. Monteleone, E. Esposito, C. Muzzi, E. Tocci, M. Longo, M. P. De Santo, M. Lanč, K. Friess, O. Vopička, P. Izák and J. C. Jansen, J. Membr. Sci., 2020, 594, 117460 Search PubMed.
- Q. Song, S. Cao, R. H. Pritchard, B. Ghalei, S. A. Al-Muhtaseb, E. M. Terentjev, A. K. Cheetham and E. Sivaniah, Nat. Commun., 2014, 5, 4813 Search PubMed.
- F. Y. Li, Y. Xiao, Y. K. Ong and T. S. Chung, Adv. Energy Mater., 2012, 2, 1456–1466 Search PubMed.
- X. Wu, Y. Ren, G. Sui, G. Wang, G. Xu, L. Yang, Y. Wu, G. He, N. Nasir, H. Wu and Z. Jiang, AIChE J., 2020, 66, e16800 Search PubMed.
- K. R. Vijisha and K. Muraleedharan, Int. J. Greenhouse Gas Control, 2017, 58, 62–70 Search PubMed.
- G. Han, Q. Qian, K. Mizrahi Rodriguez and Z. P. Smith, Ind. Eng. Chem. Res., 2020, 59, 7888–7900 Search PubMed.
- G. Han, K. M. Rodriguez, Q. Qian and Z. P. Smith, Ind. Eng. Chem. Res., 2020, 59, 18139–18150 Search PubMed.
- S. Couck, J. F. M. Denayer, G. V. Baron, T. Rémy, J. Gascon and F. Kapteijn, J. Am. Chem. Soc., 2009, 131, 6326–6327 Search PubMed.
- R. Vaidhyanathan, S. S. Iremonger, G. K. H. Shimizu, P. G. Boyd, S. Alavi and T. K. Woo, Science, 2010, 330, 650–653 Search PubMed.
- C. H. Lau, K. Konstas, C. M. Doherty, S. J. D. Smith, R. Hou, H. Wang, M. Carta, H. Yoon, J. Park, B. D. Freeman, R. Malpass-Evans, E. Lasseuguette, M. C. Ferrari, N. B. McKeown and M. R. Hill, Nanoscale, 2020, 12, 17405–17410 Search PubMed.
Footnotes |
† Electronic supplementary information (ESI) available. See DOI: 10.1039/d1ta06530k |
‡ These authors contributed equally. |
|
This journal is © The Royal Society of Chemistry 2021 |