DOI:
10.1039/D1TA04130D
(Review Article)
J. Mater. Chem. A, 2021,
9, 19554-19588
Advances in SnO2-based perovskite solar cells: from preparation to photovoltaic applications
Received
16th May 2021
, Accepted 22nd July 2021
First published on 22nd July 2021
Abstract
Perovskite solar cells (PSCs) have recently demonstrated a rapid power conversion efficiency of above 25%. In terms of physical properties, SnO2 is similar to TiO2 but with stronger charge extraction at the interface. Furthermore, the SnO2 electron transporting layer (ETL) is prepared using new, simple, and efficient methods, resulting in high-performance PSCs. This review initially described recent progress in SnO2 nanostructures and preparation methods. The passivation options were then divided into elemental doping, bilayer alterations, and interfacial modifications. Finally, we discussed the challenges and limitations of SnO2 ETL-based PSCs and made recommendations for further research.
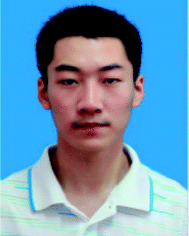 Pengfei Wu | Pengfei Wu is currently a PhD candidate in Applied Chemistry at Tianjin University under the supervision of Prof. Shirong Wang. He received his B. S. degree (2019) from China University of Petroleum (East China), China. His current research focuses on low-dimensional perovskites and efficient perovskite solar cells. |
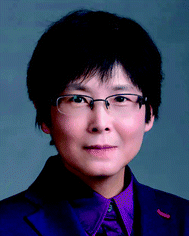 Shirong Wang | Shirong Wang is currently a Professor in the School of Chemical Engineering and Technology at Tianjin University. She received her B. S. degree (1991) and a PhD degree in applied chemistry at Tianjin University. She worked as a postdoctoral researcher in the State Key Laboratory of Fine Chemicals at the Dalian University of Technology. Her main research interests include perovskite solar cells, organic/quantum dot light-emitting diodes, and metal halide perovskite nanocrystals. |
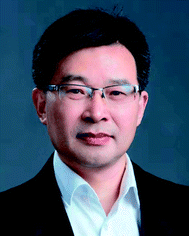 Xianggao Li | Xianggao Li is currently a Professor in the School of Chemical Engineering and Technology and the Department of Fine Chemical Engineering Director at Tianjin University. He received his B. S. degree in 1983 from Hunan University and a PhD. degree from the School of Chemical Engineering and Technology at Tianjin University, respectively. His main research interests include perovskite solar cells, organic/quantum dot light-emitting diodes, and metal halide perovskite nanocrystals. |
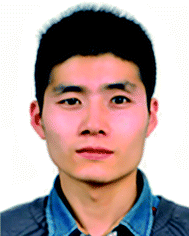 Fei Zhang | Fei Zhang is currently a Professor in the School of Chemical Engineering and Technology at Tianjin University. He received his B.Eng. (2011) and a PhD degree (2017) under the supervision of Prof. Shirong Wang at Tianjin University. He was a visiting PhD student in LPI at Ecole Polytechnique Fédérale de Lausanne (EPFL) under the supervision of Prof. Michael Grätzel and Dr Shaik Mohammed Zakeeruddin. Then, he worked as a Postdoctoral Researcher in the Chemistry and Nanoscience Center at the National Renewable Energy Laboratory under Dr.Kai Zhu's supervision. His interests concentrate on synthesizing low-dimensional perovskites, lead-free perovskites, and device engineering for perovskite optoelectrical devices. |
1. Introduction
Nowadays, perovskite solar cells (PSCs) have attracted substantial attention due to low expense, facile production, and high power conversion efficiency (PCE).1–5 Besides, the rapid development of theoretical and practical research has boosted its PCE from 3.8%
6 to 25.5%
3,7–12 in just ten years, which is close to that of polycrystalline silicon solar energy cells (Fig. 1a).
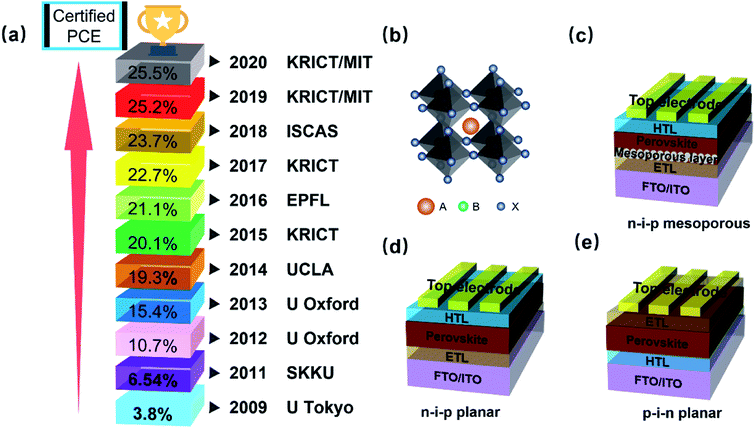 |
| Fig. 1 (a) Brief development history of PSCs (2009,6 2011,28 2012,29 2013,30 2014,31 2015,32 2016,33 2017,34 2018,35 2019,36 and 2020,7). (b) Crystal structure of cubic for metal halide perovskite. (c) PSCs with an n-i-p mesoporous structure. (d) PSCs with a regular n-i-p planar structure. (e) PSCs with a p-i-n planar structure. | |
The general chemical formula of perovskite is ABX3, in which A involves an organic cation group or inorganic metal cations such as Methylammonium (CH3NH3+), formamidinium (NH2CH
NH2+), as well as cesium (Cs+); B is generally a heavy metal cation such as lead(II) (Pb2+) and tin(II) (Sn2+); X is commonly a halide ion including chlorine (Cl−), bromide (Br−), or iodine (I−).13,14 (Fig. 1b) The Goldschmidt tolerance factor (t),15 is a simple way to check whether or not specific compositions can form a stable perovskite structure.
where
rA,
rB, and
rX represent the corresponding ionic radius of A, B and X, respectively. The 3D perovskite structure can be stable only when
t is located in the range of ∼0.8–1.0.
16
PSC structures are always classified into two types: n-i-p structures and p-i-n structures. The n-i-p structures are of two types: n-i-p mesoporous and n-i-p planar structures, whereas the p-i-n structures are only p-i-n planar structures (Fig. 1c–e).4,17,18 The n-i-p mesoporous structures are usually composed of a transparent conductive electrode such as fluorine-doped tin oxide (FTO) and indium tin oxide (ITO), an electron transporting layer (ETL), a mesoporous scaffold layer (which is always TiO2 or Al2O3), a perovskite absorption layer, a hole transporting layer (HTL) and a top electrode. However, the TiO2 mesoporous layer is always post-treated at high temperatures (typically above 450 °C) to increase the conductivity and remove the organic material.19–25 By contrast, the planar structure of n-i-p PSCs without a mesoporous scaffold also achieves a similar efficient power output and long-term stability to the device with the mesoporous configuration.26,27
TiO2 is a widely utilized ETL in PSCs for n-i-p planar structures due to its proper bandgap and good transmittance.37–40 However, when tested under continuous light illumination, TiO2 obtains electrons from I−, leading to perovskite cubic structural damage and generation of I2.41–43 Furthermore, TiO2 has a disadvantage of poor electron mobility (10−5 cm2 V−1 s−1) due to impaired intrinsic electron mobility (<1 cm2 V−1 s−1).44 Furthermore, organic residuals must be removed using high-temperature procedures, which will take a significant amount of time and energy. For high-performance PSCs, an appropriate and chemically stable ETL is required.
For efficient and stable PSCs, we must adhere to key ETL material principles: (a) high transmittance with minimum optical energy loss, (b) appropriate bandgap matching, (c) high conductivity, (d) low cost and (e) good reproducibility.45–48 Many metal oxides with unique optical and electrical properties have been reported as potential candidates for replacing TiO2 (Fig. 2), including metal oxides (ZnO,49–53 In2O3,54 Nb2O5,55,56 WO3,57,58 Fe2O3,59–62 and CeO2
63,64) ternary metal oxides (Zn2SnO4,65,66 BaSnO3
67–69 and SrTiO4
70,71), metal sulfides (MoS,72,73 CdS,74–76 In2S3,73 SnS2
77 and Bi2S3
78), GaN79 and CdSe;80,81 and InGaZnO4.82 However, some drawbacks still exist, like low PCE, poor interfacial contact, or high energy consumption.
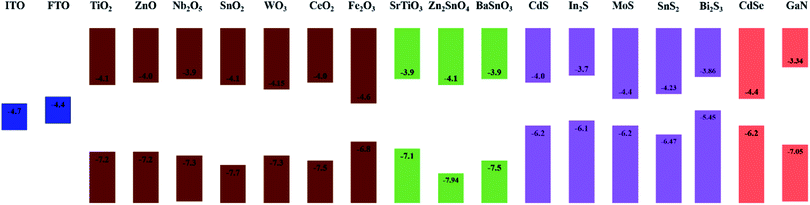 |
| Fig. 2 Conduction band (CB) and valence band (VB) of widely introduced inorganic metal materials as ETLs in PSCs. (blue: metal oxides: green: ternary metal; yellow: metal sulfide; pink: CdSe; and purple: GaN). | |
Since the first report of 6.5% by Dai et al. and the subsequent report of above 15% by Yan et al. in 2015,83,84 the performance of SnO2-based PSCs has exceeded 25%, making SnO2 an appealing ETL in PSCs and is considered a potential alternative instead of TiO2·SnO2 possesses the following remarkable properties: (1) depth CBM and optimal energy level alignment;85 (2) high bulk electron mobility (significantly greater than TiO2 ETL) and high conductivity;86,87 (3) large bandgap (3.6–4.5 eV) and high optical transparency;88,89 (4) high-temperature flexible deposition; and (5) outstanding stability under light, heat, and moisture, with minimal photoactivity.90,91 TiO2 in PSCs usually requires a mesoporous layer and especially high-temperature post-treatment (above 450 °C) to get dense with superior conductivity and crystallinity whether in a spin coating or spraying method, which costs more energy and expense. The SnO2 ETL is often achieved using spin coating and chemical bath deposition, requiring just post-treatment at low temperatures (≤200 °C), which is advantageous for a large-scale preparation.
In this review, we present an overview of the use of SnO2 in PSCs, including standard preparation procedures, SnO2 nanostructures, and performance optimization techniques such as elemental doping, surface modifications, and bilayer design. Then, we go into hysteresis and stability issues in further detail. Finally, we explore the problems and limits of SnO2 ETL-based PSCs, as well as possible future research directions.
2. Preparation methods of SnO2
The SnO2 film is typically prepared through solution processing, chemical bath deposition (CBD), and atomic layer deposition (ALD). Recently, emerging methods for deposition of SnO2 such as e-beam evaporation, magnetron sputtering, and electrochemical deposition are also reported to improve the conformability of SnO2 for flexible and large-scale PSCs. Fig. 3 shows the schematic illustration of various processing methods for SnO2 ETLs. We just picked some of the representative data of reported different preparation methods, even though other factors may have an impact on the performance. So, here, we have talked more about performance optimization strategies in detail in Section 4. Aside from these, the PCE is also influenced by the rigid or flexible substrate, preparation environment (N2 or air), and electrode types.
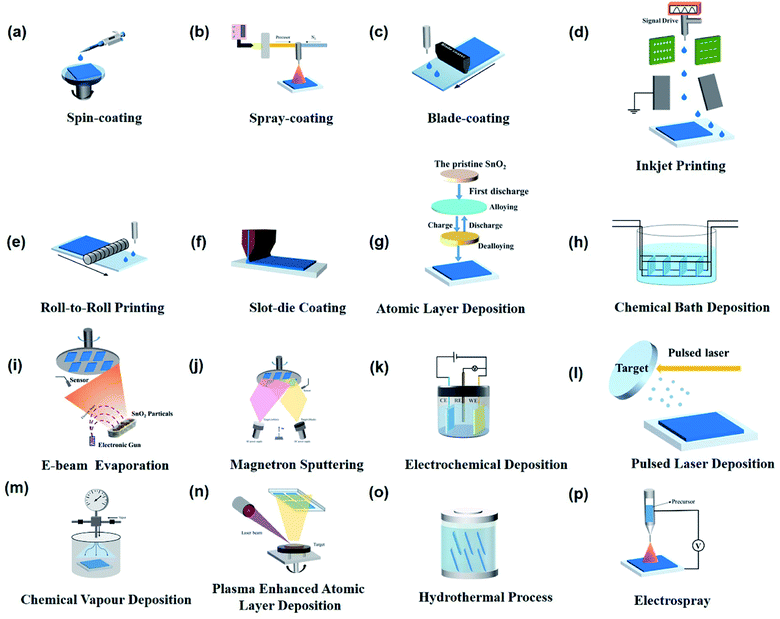 |
| Fig. 3 Schematic illustration of common SnO2 deposition processes in PSCs. (a) Spin-coating. (b) Spray-coating. (c) Blade-coating. (d) Inkjet-printing. (e) Roll-to-roll printing. (f) Slot-die coating. (g) Atomic layer deposition. (h) Chemical bath deposition. (i) E-beam evaporation electrospray. (j) Magnetron sputtering. (k) Electrochemical deposition. (l) Pulsed laser deposition. (m) Chemical vapor deposition. (n) Plasma enhanced atomic layer deposition. (o) Hydrothermal process. (p) Electrospray. | |
2.1 Solution-processable method
The solution process contains the thermal decomposition method, sol–gel method, deposition of synthesized SnO2 nanoparticles (NPs), and commercialized SnO2 colloidal precursors.92–94 In the meantime, spin-coating,95–98 spray-coating,99–101 slot-die coating,100,102,103 roll-to-roll microgravity printing,104 blade-coating105,106 and inkjet-printing107,108 are valuable technologies to fabricate quality SnO2 films.
2.1.1 Thermal decomposition method.
For thermal decompositions, Sn-based salts (SnCl2109,110 or SnCl4,111–113 or their hydrates SnCl2·2H2O114,115 or SnCl4·5H2O116,117) were first dissolved in polar solvents such as alcohol and deionized water, which were then deposited on an ITO or a FTO substrate by spin-coating and gradually converted into SnO2 through thermal annealing in ambient air. It should be noticed that the humidity and temperature of the environment significantly influence the annealing process and the quality of the obtained SnO2 films.
Zhang and co-workers prepared SnO2 thin films using SnCl4 as the tin source at low temperatures.112 Furthermore, they compared the passivation by SnCl4 at FTO/ETL with that at the ETL/perovskite interface to study the effect of the SnCl4 pre-treatment and post-treatment on SnO2 (Fig. 4a). With the assistance of SnCl4 pre-treatment, the devices based on Cl–SnO2 ETL obtained the best efficiency of 18.6% as compared to the post-treatment process (17.3%). The results confirmed that both modifications could boost the photovoltaic performance of the PSCs. However, SnCl4 pre-treatment increased SnO2 ETL in electron coupling with FTO, leading to higher electron mobility and better charge extraction efficiency.112
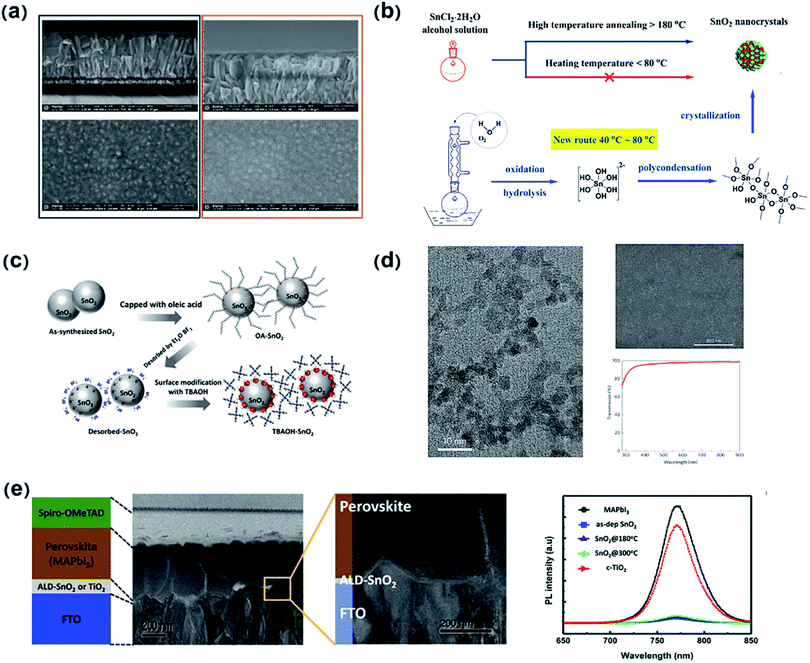 |
| Fig. 4 (a) Cross-sectional and top-view SEM images (left) of the FTO/SnO2 NC substrate and (right) FTO/Cl–SnO2. Reproduced with permission.112 Copyright 2020, Elsevier. (b) Schematic diagram of the wet chemical route to synthesize SnO2 nanocrystals. Reproduced with permission.114 Copyright 2017, Elsevier. (c) Schematic illustration of the ligand exchange procedure for TBAOH-capped SnO2 nanoparticles. Reproduced with permission.124 Copyright 2017, ACS Publishing Group. (d) TEM and SEM images of SnO2 nanoparticles and the transmission spectrum of SnO2 films. Reproduced with permission.44 Copyright 2017, Nature Publishing Group. (e) Device architecture and cross-sectional TEM images, and PL spectra of the device based on the ALD–SnO2 films. Reproduced with permission.125 Copyright 2019, Royal Society of Chemistry. | |
Annemarie Pucci and co-workers prepared SnO2 thin films using SnCl2·2H2O as the tin source. Their work elucidated the influence of two different solvents and various annealing temperatures on the layer morphology of SnO2 thin films. It was found that different morphologies were mainly observed during the spin-coating process and not the subsequent annealing process. Furthermore, high annealing temperatures (≈400 °C) do not entirely omit the differences in morphology, which were processed from the two kinds of solution systems. Although this device still showed severe hysteresis, the device with inkjet-printed SnO2 layers presented the highest output efficiency of about 19%.107
2.1.2 Sol–gel method.
Sol–gel is one of the most commonly used methods to produce thin dense films and powder catalysts at large homogeneous concentrations and under stoichiometry control because of the simplicity, low energy, reliability, reproducibility, and relatively mild fabrication conditions.118–121
Lee and co-workers introduced a simple sol–gel method to form a SnO2 bilayer (Bi–SnO2) as ETL with dopant-free and amorphous–crystalline heterophase propertie by a sequential spin-coating process. Bi–SnO2 demonstrated a smooth morphology, low density of energy level traps, and appropriate bandgap matching. As a consequence, Bi–SnO2 PSCs with active areas (≈3.55 cm2) reached up to about 15% with less hysteresis.122 Xu et al. optimized the aging time, and the PSCs based on the sol–gel SnO2 film yielded the best PCE of 19% with excellent light trapping ability and a textured SnO2 structure. But the sol–gel SnO2 film suffered from poor interfacial contact electrical properties with the perovskite absorber layer because of the annealing effects, resulting in the degradation of performance of PSCs.123
Wang et al. reported a whole sol–gel crystallized SnO2 fabrication process below 80 °C (Fig. 4b).114 Participation of environmental O2 and H2O via various methods of refluxing is essential as it could substantially lower the Sn2+ oxidation and lead to the hydrolysis of SnCl2·2H2O alcohol solution, alternatively establishing an energetically favorable pathway for SnO2 crystallization at low temperatures. The devices had a PCE and a steady-state PCE of 19.20% and 18.48%, respectively, which are significantly better than those of the devices based on high temperature annealed TiO2 ETLs (16.61% and 15.03%).
2.1.3 SnO2 nanoparticle synthesis method.
It is an efficient method to prepare a compact SnO2 film by depositing synthesized SnO2 nanoparticles (NPs) for high performance PSCs.126,127 To reduce the recombination centers in the SnO2 film, SnO2 NPs should be washed prior to the removal of residuals after synthesizing SnO2. The main issues for the utilization of SnO2 NPs are the dispersibility and the choice of solvent. A suitable solvent should be able to disperse NPs nicely and should not damage the perovskite layer.
Lee and co-workers selected tetrabutylammonium hydroxide (TBAOH) to successfully disperse SnO2 NPs (TBAOH–SnO2) well in ethanol via the ligand exchange method (Fig. 4c).124 Using this TBAOH–SnO2 NPs as ETL, the PSCs effectively reached up to 18.77% because of minor charge accumulation and good energy level alignment. Besides, the device with a TBAOH–SnO2 NPs layer only reduced approximately 10% in PCE, which showed improved thermal stability by TBAOH treatment.124 For SnO2 deposition, the crystallinity and morphology of the SnO2 films significantly relied on the annealing temperature.
Park and co-workers investigated the SnO2 formation at different annealing temperatures (RT, 80 °C, 120 °C, 160 °C, and 200 °C). It was found that when SnO2 was annealed at 120 °C, the champion PCE was obtained (19.0%). Using AFM and UPS, both the smooth surface and suitable band alignment of SnO2 film deposition at low temperatures have been observed, contributing to obtaining a high PCE and long stability of the device.73 However, traditional deposition of the SnO2 film imposes restrictions on the substrate choice and commercial applications because of a relatively high temperature and/or a long-duration sintering step. Many scientists came up with other creative post-annealing processes to deal with this problem, including microwave-assisted annealing,126 intense pulsed photonic annealing,128 and UV-sintering methods.129,130
2.1.4 SnO2 colloidal precursor.
You et al. formed a compact and uniform SnO2 film by using a commercialized SnO2 colloidal precursor (Fig. 4d).44 The process was that the SnO2 colloidal nanoparticle precursor was spin-coated at the medium speed rate on top of the ITO electrode. These substrates were annealed at 150 °C for 30 min to evaporate water. The device with SnO2 film deposition at low temperatures initially achieved 19.9% certificated efficiency. Later on, introducing a surface passivation layer of PbI2 and an organic halide salt phenethylammonium iodide (PEAI) boosted the certificated efficiency to 20.9%
131 and 23.32%.35 Tan and co-worker added KCl to the SnO2 colloidal precursor to passivate the ETL/perovskite interface and at the grain boundaries by K/Cl ions (SnO2–KCl).132 The strategy can enhance the Voc from 1.077 to 1.137 V, and a corresponding PCE increased from 20.2% to 22.2% for the devices using SnO2–KCl composite ETL.
2.2 Atomic layer deposition (ALD)
ALD is considered a promising deposition technique to fabricate ultra-thin and dense metal oxide layers with increased light transmittance based on a self-limiting surface reaction. Due to the precise control at the atomic level, the high-quality film of metal oxides has the merits of promoting charge transfer, suppressing the degradation caused by the external environment and internal ionic migration, and enhancing the photoelectric properties of the device.133,134
Lee and co-workers prepared planar SnO2-based PSCs using ALD by modulating the deposition and post-annealing temperatures. It was found that the post-annealing process can effectively passivate the perovskite and SnO2 interface, leading to reduced charge recombination. As a result, SnO2 based PSC with post-annealing achieved a PCE of 20% with high reproducibility and stability.135 Grätzel and co-workers prepared an amorphous SnO2 film by ALD, which can maintain its dense morphological characteristics even during the annealing process at 450 °C.87
Jeong and co-workers deposited thin SnO2 films by ALD with subsequent annealing at 180 °C as ETLs. In the cross-sectional image by transmission electron microscopy (TEM), the thicknesses of the SnO2 film were around 12 nm, similar to those of TiO2 (Fig. 4e). Compared with the c-TiO2/MAPbI3 sample, SnO2@180 °C/MAPbI3 showed a much decrease in PL intensity (≈94%), which exhibited an increase in photo-generated electron extraction from perovskite. Finally, PSCs based on SnO2 with annealing at 180 °C showed the highest PCE (>18%) with better reproducibility.125 Although ALD has these attractive advantages, it is necessary to deposit thin films under vacuum conditions and a matched operating space for scalable fabrication, resulting in high preparation costs.
2.3 Chemical bath deposition (CBD)
CBD is seen as a valuable technique to deposit a thin film of metal oxides as a buffer layer in photovoltaic cells, which has many advantages such as low fabrication cost, low-temperature process, suitability for different electrodes, and great reproducibility.136–138
Jun Hong Noh and co-workers prepared SnO2 by CBD as ETL and employed poly(3-hexylthiophene) (P3HT) with gallium(III) acetylacetonate (Ga(acac)3) additives. Consequently, they boosted the performance of free-doping PCE over 24% with a Voc of 1.15 V and a FF of 83.8%. It also showed superior water-resistant property for 2000 h under 85% RH without any encapsulation.139 Ko and co-workers fabricated a self-controlled SnO2 through a convenient CBD.140 It was found that the common-ion and precursor concentrations can effectively tune the growth of the SnO2 film on FTO, leading to a uniform and compact SnO2 layer. Finally, the device with a hydrolyzed SnO2 layer presented an excellent PCE of 20.21%.
Yoo and co-workers first prepared SnO2 as the ETL using CBD by controlling the formation of Sn intermediate species, which depended on the decomposition pathway of the Sn2+ precursor (SnCl2), including four stages. Their SEM images of the SnO2 layer showed that as the reaction time increases, the pH of the reaction solution increased, and the size of the SnO2 domain increased from around 50 nm to about 100 nm. Especially, a SnO2 layer formed a complete coverage on FTO at stage A-ii (pH 1.5), which can be observed from TEM images. The XRD confirmed the presence of various Sn intermediate species, which relied on the pH of the reaction solution (Fig. 5). Moreover, they added the MAPbBr3 + MACl additive in perovskite to stabilize the intermediate phase, enhance the perovskite orientation, and introduce alkylammonium bromide for the 2D perovskite passivation. Due to the holistic method, they obtained a certified PCE of 25.2% (Table 1).36 Despite the merits of simple operation and low cost, the quality of films in CBD is strongly determined by the processing parameters, including the temperature, concentration, and pH. In the meantime, the pollution of residual precursors after fabrication and the necessity for frequent bath replacement are also critical issues for the commercialization of PSCs.
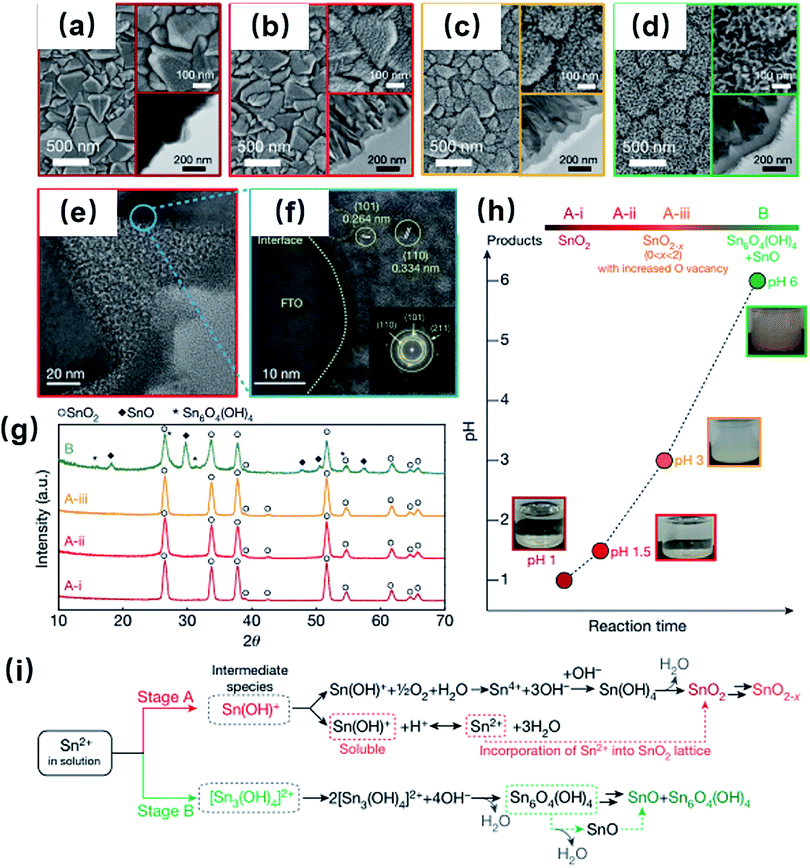 |
| Fig. 5 (a–d) SEM images of SnO2 at different stages: (a) A-i; (b) A-ii; (c) A-iii; and (d) B. The insets shown are SEM (top-right) and the corresponding cross-sectional TEMs (bottom-right). (e) and (f) High-resolution TEM images of SnO2 at stage A-ii (the inset: a fast Fourier transform pattern of the TEM image). (g) XRD patterns of the SnO2 films at different reaction stages. (h) Schematic illustration of the reaction progress with a photograph of the reaction solution at each stage. (i) Schematic illustration of the overall reaction mechanism for forming the SnO2-based films. Reproduced with permission.36 Copyright 2021, Nature Publishing Group. | |
Table 1 Representative PSCs based on SnO2 ETL fabricated by different preparation methodsa
Device structure |
Perovskite |
Deposition method |
Tin source |
J
sc (mA cm−2) |
V
oc (V) |
FF (%) |
PCE (%) |
Ref. |
HTL(a): spiro-OMeTAD; HTL(b): poly(3-hexylthiophene) (P3HT); C8H24N4Sn: tetrakis(dimethylamido)tin(IV); GQDs: graphene quantum dots; CBD: chemical bath deposition; PLD: pulse laser deposition; ALD: atomic layer deposition; CVD: chemical vapor deposition; PEALD: plasma-enhanced atomic-layer deposition.
|
ITO/SnO2/PCBM:Bphen/perovskite/HTL(a)/Au |
Cs0.04FA0.92MA0.04PbI3 |
Spin-coating |
SnO2 colloidal precursor |
25.15 |
1.167![[thin space (1/6-em)]](https://www.rsc.org/images/entities/char_2009.gif) |
78.64 |
20.39 |
122
|
ITO/SnO2/perovskite/HTL(a)/Au |
MAPbI3 |
Spin-coating |
SnCl4 |
23.2 |
1.12 |
71.4 |
18.6 |
112
|
FTO/SnO2/perovskite/HTL(a)/Au |
MAPbI3 |
Spin-coating |
SnCl2·2H2O |
22.88 |
1.06 |
82.54 |
18.89 |
123
|
ITO/SnO2/perovskite/HTL(a)/Au |
Cs0.05FA0.79MA0.16PbI2.45Br0.55 |
Spray-coating |
SnO2 colloid dispersion |
22.2 |
1.17 |
76.1 |
19.8 |
100
|
FTO/SnO2:GQDs/perovskite/HTL(a)/Au |
(FAPbI3)0.85(MAPbBr3)0.15 |
Spray-coating |
SnCl2·2H2O |
22.5 |
1.12 |
65.8 |
17.08 |
99
|
FTO/SnO2/perovskite/HTL(a)/Au |
MAPbI3 |
Spray-coating |
SnO2 colloid dispersion |
21.63 |
1.002 |
78 |
16.91 |
101
|
ITO/SnO2/perovskite/HTL(a)/Au |
Cs0.05(FA0.85MA0.15)0.95Pb(I0.85Br0.15)3 |
Slot-die-coating |
SnO2 colloid dispersion |
22.60 |
1.148 |
79 |
20.50 |
103
|
PEN/ITO/SnO2/perovskite/HTL(a)/Ag |
FAxMAyCs1−x−yPb(IzBr1−z)3 |
Roll-to-roll |
SnO2 colloid dispersion |
19.96 |
1.07 |
70.09 |
16.60 |
104
|
ITO/SnO2/perovskite/HTL(a)/Ag |
Cs/FAxMA1−xPbIyBr3−y |
Blade-coating |
SnO2 colloid dispersion |
23.1 |
1.08 |
72 |
18 |
106
|
FTO/SnO2/perovskite/HTL(a)/Au |
CsPbI3 |
Blade-coating |
SnO2 colloid dispersion |
20.67 |
1.12 |
81.98 |
19 |
105
|
ITO/SnO2/perovskite/HTL(a)/Au |
Cs0.10FA0.75MA0.15Pb(Br0.15I0.85)3 |
Inkjet-printing |
SnCl2·2H2O |
23.6 |
1.11 |
72 |
18.8 |
107
|
FTO/SnO2/perovskite/HTL(a)/Au |
MAPbI3 |
ALD |
C8H24N4Sn |
22.6 |
1.07 |
75.6 |
18.3 |
125
|
FTO/SnO2/TiO2/perovskite/HTL(a)/Au |
(FAPbI3)0.85(MAPbBr3)0.15 |
ALD |
C8H24N4Sn |
22.67 |
1.13 |
78 |
20.03 |
135
|
FTO/SnO2/perovskite/HTL(a)/Au |
FAPbI3 |
CBD |
SnCl2·2H2O |
25.09 |
1.194 |
84.7 |
25.4 |
36
|
FTO/SnO2/perovskite/HTL(b)/Au |
(FAPbI3)0.95(MAPbBr3)0.05 |
CBD |
SnCl2·2H2O |
25.5 |
1.15 |
83.8 |
24.6 |
139
|
FTO/SnO2/perovskite/HTL(a)/Au |
Cs0.05(MA0.17FA0.83)95Pb(I0.83Br0.17)3 |
E-beam evaporation |
SnO2 powders |
22.47 |
1.08 |
71 |
17.38 |
142
|
FTO/Zn–SnO2/perovskite/HTL(a)/Au |
FAxMAyCs1−x−yPb(Iz,Br1−z)3 |
E-beam evaporation |
SnO2 powders |
22.72 |
1.11 |
75 |
18.95 |
143
|
FTO/SnO2/perovskite/HTL(a)/Au |
Cs0.06MA0.27FA0.67PbI2.7Br0.3 |
Magnetron sputtering |
SnO2 target |
23.7 |
1.08 |
79 |
20.2 |
148
|
FTO/SnO2/perovskite/HTL(a)/Au |
FA0.85MA0.15Pb(I0.85Br0.15)3 |
Magnetron sputtering |
SnO2 target |
22.58 |
1.065 |
75.6 |
18.2 |
149
|
FTO/SnO2/PCBM/perovskite/HTL(a)/Au |
MAPbI3 |
PLD |
SnO2 target |
21.51 |
1.11 |
73 |
17.29 |
164
|
FTO/SnO2/C60-SAM/perovskite/HTL(a)/Au |
MA0.7FA0.3PbI3 |
PEALD |
SnO2 target |
22.71 |
1.113 |
80.75 |
20.41 |
156
|
ITO/SnO2/PCBM/perovskite/HTL(a)/Ag |
MAPbI3 |
Electrodeposition |
SnCl2 |
19.75 |
1.08 |
65 |
13.88 |
150
|
FTO/SnO2/perovskite/HTL(a)/Au |
Cs0.05(MA0.17FA0.83)0.95Pb(I0.83Br0.17)3 |
Combustion |
SnCl2·2H2O |
23.85 |
1.122 |
78.2 |
20.92 |
165
|
FTO/SnO2/PCBM/perovskite/HTL(a)/Ag |
MAPbI3 |
CVD |
SnCl2 |
14.7 |
1.03 |
67.51 |
10.2 |
159
|
FTO/SnO2/C60/perovskite/HTL(a)/Au |
MAPbI3 |
Electrospray |
SnCl2·2H2O |
23.7 |
1.103 |
77.3 |
20.2 |
163
|
FTO/SnO2/perovskite/HTL(a)/Au |
Cs0.05(MA0.17FA0.83)0.95Pb(I0.83Br0.17)3 |
Hydrothermal |
SnCl4·5H2O |
22.69 |
1.129 |
72.70 |
18.62 |
117
|
FTO/SnO2/perovskite/HTL(a)/Au |
MAPbI3 |
Thermal evaporation |
SnO2 powder |
23.36 |
1.04 |
69.2 |
16.79 |
161
|
P-FTO/perovskite/HTL(a)/Au |
MAPbI3 |
In situ plasma etching of FTO |
Commercial FTO substrate |
23.85 |
1.11 |
77.12 |
20.39 |
160
|
FTO/G-SnO2/C–SnO2/perovskite/HTL(a)/Au |
Cs0.05(MA0.17FA0.83)0.95Pb(I0.83Br0.17)3 |
Ball-milling |
SnO2 powder |
21.16 |
1.22 |
80.09 |
21.09 |
158
|
2.4 E-beam evaporation
E-beam evaporation is one of the high vacuum techniques, which generates more compact films and minimal waste of resources in contrast to the spin-coating process and ALD, and is beneficial to the large-scale application and commercialization of PSCs.141
Ma and co-worker fabricated SnO2 as ETL by e-beam evaporation for possible commercialization of PSCs with large-scale manufacture. As the SnO2 target source evaporated, the e-beam evaporation process could simulate thousands of SnO2 film substrates at one time by changing the position of the substrate holder. The advantages of e-beam evaporation were that the thickness and the crystallinity of the SnO2 film could be controlled precisely. Consequently, the PSCs with a uniform SnO2 film by e-beam evaporation demonstrated excellent performance of 18.2% and remarkable waterproof, which retained 97% of its original efficiency value with a relative humidity of 85% for over 34 days.142 Despite the relatively high deposition rates, e-beam evaporation has the disadvantage of controlling the film composition precisely.
Later, to reduce the resistivity of SnO2, Song and co-workers prepared SnO2 ETL by e-beam evaporation combined with Zn doping (Zn–SnO2). Following Zn doping, the Zn–SnO2 layer improved the charge mobility, inhibited the charge accumulation at the interface, and optimized the SnO2 energy level structure, resulting in a PCE increase from 18.95% to 20.16% with long-term stability. More importantly, after 100 bending tests, the flexible device maintained a PCE of over 15% (Fig. 6a and b).143 Furthermore, Li and co-workers used an oxygen plasma-triggered e-beam evaporation approach to create SnO2 films at ambient temperature without annealing. The oxygen plasma can precisely tune the stoichiometry of SnO2 films in the evaporation process due to its intense oxidation activity, thereby endowing SnO2 with uniformity, high transmittance, high Hall mobility, and good hydrophilicity.144 Aside from the requirement for a high vacuum, this approach is limited by X-ray damage on substrates and slow conduction speeds.
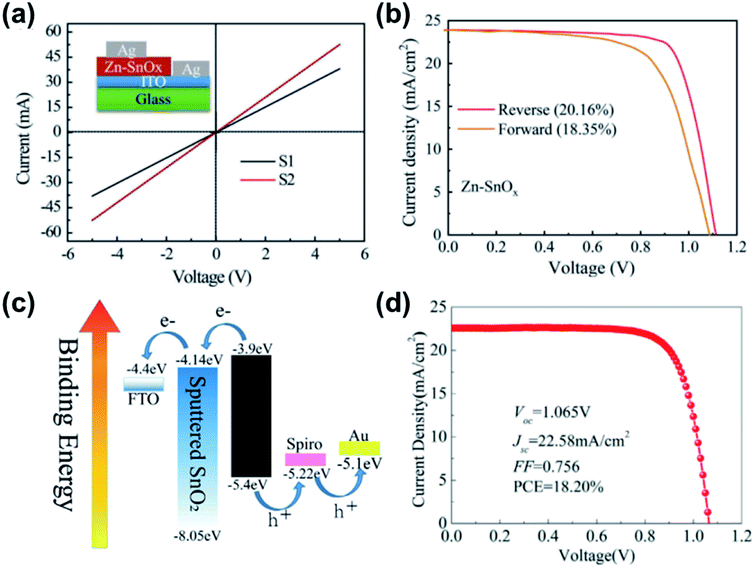 |
| Fig. 6 (a) Conductivity of bare SnOx (S1) and Zn–SnOx (S2); (b) the reverse scan J–V curves of the device based on Zn–SiOx. Reproduced with permission.143 Copyright 2020, Wiley-VCH. (c) Energy level scheme for various layers in a sputtered SnO2 based device. (d) J–V curves under the reverse voltage scan of the best device with sputtered SnO2. Reproduced with permission.149 Copyright 2019, Elsevier. | |
2.5 Magnetron sputtering
Magnetron sputtering is a mature and reliable deposition method with the use of low-cost metal-oxide targets to prepare metal-oxide thin films in the lab- and industrial-scale.145,146 SnO2 particles are sputtered by high energy argon ions, react with the reaction gas (like high purity oxygen), and then deposited on the top of the FTO, which is a continuous process. Magnetron sputtering has excellent merits, including the precise control of film density and thickness, low waste of raw materials, a mild deposition process, and low production cost. Besides, the deposition process is usually operated in a high vacuum chamber, leading to high repeatability with minor damage to the natural environment.147
Qiu and co-workers systematically investigated the chemical and physical characteristics of sputtered SnO2. It played a significant role in the formation of high quality to control the oxidizing process. Through improvement in the device architecture, the device with sputtered SnO2 ETL exhibited the champion PCE of 20.2% and a useful life of 625 h under T80 measurement, confirming the enhanced conductivity of SnO2.148 Meanwhile, Bai and co-workers discovered that while changing the working gas ratio of Ar/O2 can result in identical shape and crystallinity of sputtered SnO2, it can also result in different trap states and carrier transit dynamics in PSC devices. The devices based on sputtered SnO2 ETL demonstrated a champion PCE (up to 18.20%) (Fig. 6c and d).149 Additionally, Otoufi and co-workers introduced sputtered SnO2 on the TiO2 layer to form TiO2/SnO2 bilayers to improve electron extraction further and achieve a better PCE of 12.3% in comparison with only TiO2 as ETL (8.18%).146 The gas flows played a crucial role in controlling the oxygen vacancies. On the other hand, tail states within the bandgap are caused by the amorphous or nanocrystallinity in the films, which are effectively suppressed by interface passivation.
2.6 Other deposition methods
Besides the solution-processable and vacuum evaporation methods, other creative methods are emerging for high-quality SnO2 films due to demands for different applications. For example, Chen et al. prepared PSCs with SnO2-ETLs by electrochemical deposition. The PSCs using MAPbI3 as the light-absorbing layer obtained a PCE of 13.88% with negligible hysteresis.150 The electrochemical deposition was beneficial for SnO2 preparation at low temperatures (50 °C) without introducing a seed layer or a post-treatment process.151 The dual-fuel combustion method was usually an excellent choice to control the SnO2 growth.152,153 Compared to the conventional solution-process method, as combustion synthesis was ignited, the method not only requires significant external energy input but also is exothermic, leading to reduced production cost.
Additionally, other physical and chemical preparation technologies were also used to deposit SnO2 ETLs, including plasma-enhanced atomic layer deposition (PEALD),154–157 high energy ball-milling,158 chemical vapor deposition (CVD),159in situ plasma etching of FTO,160 thermal evaporation,161 hydrothermal processes,117,162 and electrospray method.163 In these methods, the SnO2 film crystallized well during the deposition process on the substrates without further post-heating treatment. Thus, these methods demonstrate significant advantages for the mass production of flexible PSCs, even though the process tends to take much time.
3. Nanostructures of SnO2
3D SnO2 materials such as SnO2 nanoparticles or nanocrystals are commonly exploited as ETL.83 However, Zhao et al. found that 0D–2D SnO2 ETLs can reduce light scattering from rough FTO and enlarge the perovskite grain size, leading to improved performance.166 Nanostructured SnO2 is a critical factor for high-efficiency PSCs to scale up planar PSCs for industrial applications. On the one hand, it can optimize the surface wettability for smooth perovskite coverage on the substrate. On the other hand, nanostructured SnO2 (Fig. 7) enhances the interfacial contact to eliminate the notorious electronic trap states, which removes the energy level traps at the interface. So, the dimensional control of SnO2 is a promising strategy to prepare SnO2 ETL with a high photoelectrical property.
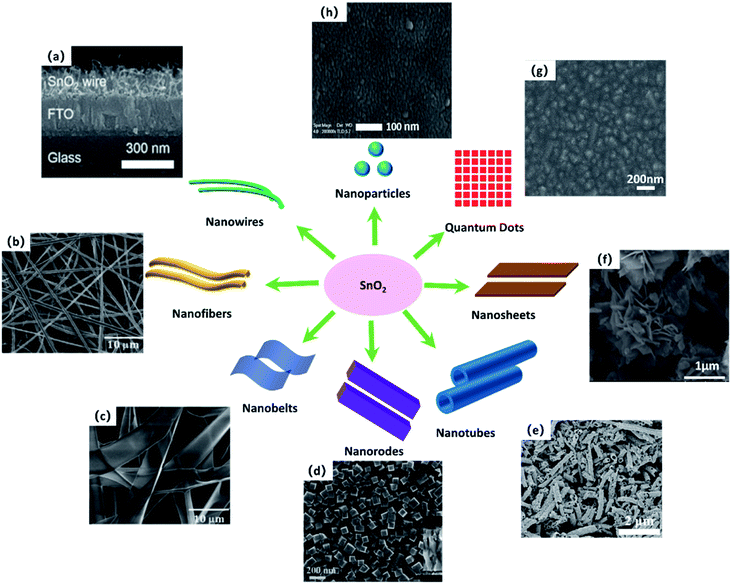 |
| Fig. 7 Schematic diagram of various nanostructures of SnO2 in accordance with SEM images of various SnO2 nanostructures: (a) nanowires reproduced with permission.167 Copyright 2015, Royal Society of Chemistry. (b) Nanofibers and (c) nanobelts. Reproduced with permission.168 Copyright 2018, Royal Society of Chemistry. (d) Nanorods. Reproduced with permission.169 Copyright 2018, Wiley-VCH. (e) Nanotubes. Reproduced with permission.170 Copyright 2020, Elsevier. (f) Nanosheets. Reproduced with permission.171 Copyright 2016, Elsevier. (g) Quantum dots. Reproduced with permission.93 Copyright 2018, Wiley-VCH; (h) nanoparticles. Reproduced with permission,83 Copyright 2015, ACS Publishing Group. | |
3.1 SnO2 nanowires
SnO2 nanowires (NWs), a one-dimensional (1D) semiconductor material, are a good ETL in the field of PSCs due to their efficient extraction and collection capacity. Besides, 1D nanomaterials have fewer defects like grain boundaries which significantly decrease in dead-ends.172–176
Han and co-workers synthesized 1D SnO2 as the ETL by a vapor–liquid–solid (VLS) reaction for realizing high conductivity for SnO2 (Fig. 7a). The SnO2 NWs showed a high transmittance of about 80%, similar to that of the bare FTO substrate, as observed from the UV-vis transmittance spectrum. SnO2 NWs were modified with TiO2 nanoshells as TiO2/SnO2 NW ETL via plasma-enhanced atomic layer deposition (PEALD). Consequently, the TiO2/SnO2 NW ETL device displayed over 95% absorbed photon-to-current conversion efficiency (APCE) at 750 nm with a PCE of 14.2% and decreased the electron transport time by one order of magnitude in comparison with that of mp-ETL-based devices.167
3.2 SnO2 nanofibers and nanobelts
1D SnO2 nanofibers (NFs) and nanobelts (NBs) demonstrate fast electron transport rate and light scattering ability used in the dye-sensitized solar cells (DSSCs).177–179 Mali and co-workers prepared SnO2 NFs and NBs with a smooth and uniform morphology as ETL via the electrospinning technique.168 The pure tetragonal rutile phase in SnO2 NFs and NBs was presented by structural analysis. SEM micrographs exhibited that both the lengths of SnO2 NFs and NBs reached up to 500 μm with a diameter of about 450–500 nm and a thickness of 180–200 nm, respectively (Fig. 7b and c). Their optimized devices yielded a PCE of >16% based on SnO2 NBs with good shelf-life stability.168
3.3 SnO2 nanorods
Due to a particular open porous structure, SnO2 nanorods (NRs) provide more expansive space to effectively fill the pores with the perovskite crystals180–183 and enhance the light-induced photo utilization of the perovskite layer with good light scattering ability.184
Xu and co-workers reported good crystalline SnO2 NRs as the ETL with a high aspect ratio through the sophisticated solvothermal approach to promote PSCs' stability and photovoltaic performance. They showed that oleic acid (OA) ligands could precisely control the length and the diameter of SnO2 NRs without complex treatments. Besides, the insulating OA ligands had a weaker impact on the electron mobility of SnO2 NRs than on TiO2 NRs. The OA-capped SnO2 NRs ETL-based PSCs with a planar structure achieved the best efficiency of over 18%, which was much higher than that of PSCs with an OA-capped TiO2 NR ETL (14.27%).117 Zhang et al. prepared the in situ SnO2 NRs as ETL via a convenient hydrothermal method in an acidic solution.162 The average diameters of SnO2 NRs increased from 15 to 25 nm by controlling the precursor concentration, and the corresponding area density drops down to several hundreds of μm−2. Later on, to simplify the SnO2 NR fabrication process and remove the OA ligand, Lv and co-workers reported a facile hydrothermal method to synthesize highly crystalline SnO2 SRs with good light-harvesting ability (Fig. 7d). In the hydrothermal process, the reaction time and temperature are vital process parameters that determine the morphology of SR, such as the length and the diameter. In addition, a TiO2 interlayer was inserted between the perovskite absorber layer and the SnO2 ETL, forming a graded heterojunction configuration, leading to the power output of up to 18.7% with better ambient stability and repeatability.169
3.4 SnO2 nanotubes
SnO2 nanotubes (NTs) as ETL have higher conductivity and a required conduction band edge (3.6 eV) that can induce superior electrochemical properties than TiO2 (3.2 eV) (Fig. 7e).185–188 Gao and co-workers created SnO2 NTs as ETL via an in situ template self-etching strategy. The ZnO2 nanorods as sacrificial templates were covered by smooth nanoporous SnO2 shells by spin coating. The corresponding EQE spectrum suggested that SnO2 NTs based PSCs exhibited a high photocurrent of 15.9 mA cm−2 with a stable efficiency of 12.1% in more than 1000 s under simulated light illumination.189
3.5 SnO2 nanosheets
The SnO2 nanosheets (NSs) demonstrate lots of advantages such as improving photo-induced carrier collection, promoting interfacial charge transfer, and processing good weathering performance of PSCs.163,171,190–192
Hydrothermal growth is a standard method to synthesize SnO2 nanosheets. Zhou and co-workers prepared SnO2 NSs as ETL via the hydrothermal method in place of the traditional mesoporous TiO2 layer in PSCs for the first time (Fig. 7f). The device achieved a maximum efficiency of over 7% by optimizing the perovskite's crystallization time and treating it with TiCl4 aqueous solution.171 Liu and co-workers deposited a mesoporous layer of SnO2 NSs on a thin, compact SnO2 layer by using the low-temperature hydrothermal method to improve the PSC stability. The device with the highest efficiency of 16.17% was obtained through such a facile method and retained 90% of its initial PCE value in ambient after 130 d of storage without encapsulation.190 To optimize the interfacial contact with the perovskite, doping with yttrium and introduction of a C60 interlayer are done in SNs ETL-based devices. Yang and co-workers synthesized a yttrium-doped SnO2 (Y–SnO2) as ETL via an in situ hydrothermal approach at 95 °C. As a result, the Y–SnO2 based PSC achieved a champion PCE of 17.29% with free hysteresis.
Furthermore, it clearly showed that SnO2 nanosheet films demonstrated a slightly wider bandgap and a more homogeneous distribution of SnO2 nanosheet arrays after Y-doping.192 The introduction of a C60 interlayer (Y:SnO2) between SNs ETL and perovskite was reported by Wu.191 They found that the C60 interlayer can tune the energy level matching, reduce the charge accumulation and thus prolong the electron lifetime and enhance the Voc. The champion PCE obtained was 18.31%, and the device with C60-NSs preserved over 90% of its champion PCE after 500 h of storage at RT in open air.
3.6 SnO2 quantum dots
Due to the low crystallinity, inferior electron mobility at lower annealing temperatures as well as instability of the SnO2 film deposited via colloidal SnO2 solution, many researchers synthesized high-crystallinity SnO2 quantum dots (QDs) as ETL by a facile mild solution method combined with spin coating on FTO to improve the light scattering and electron transport and reduce the charge recombination, leading to a dramatic increase in PCE.193–197
Yang and co-workers reported colloidal SnO2 QDs as ETLs by a facile and repeatable two-step solution-processable method (Fig. 7g). First, they obtained the low concentration of SnO2 QDs solution by controlling the amount of thiourea in the SnCl2·2H2O water solution, and then spin-coated SnO2 QDs and changed the annealing temperature to eliminate a mass amount of thiol- and amino-groups, leading to an appropriate energy level matching and changeable carrier dynamics. Finally, the planar PSCs with SnO2 QDs ETLs achieved a maximum power output of over 20% for the planar PSCs.93 Vijayaraghavan and co-workers chose SnO2 QDs as ETL to replace the commonly used mesoporous TiO2 owing to their excellent electron extraction and hole blocking ability. For low-cost production and superior stability in air for PSCs, they designed the structure of glass/ITO/SnO2 QDs/perovskite/carbon as HTL-free PSCs and deposited carbon electrodes via low-temperature curing to substitute the widely used vacuum-deposition. As a result, the highest power output of 13.64% was obtained.198 Wang and co-workers used SnO2 QDs as ETL, which was combined with potassium hexafluorophosphate (KPF6) treatment. KPF6 had the dual-passivation in which organic cation groups were reoriented or redistributed via the strong hydrogen bonds between the PF6− group and organic cations. Moreover, KPF6 passivated the interfacial traps to suppress the energy level traps to improve the conductivity of SnO2 through the solid ionic bonds between the PF6− group and Sn4+/Sn2+.199
4. Passivation strategies of SnO2 ETLs
Although SnO2 films have several advantages over other metal oxide films, they also have comparable interface difficulties when used as the ETLs of PSCs. There are many flaws at the interfaces due to the bungle between the metal oxides and perovskite films, which degrade the presentation of PSCs. Therefore, passivations on the SnO2 film are often used to improve the performance of SnO2 based perovskite solar cells that include elemental doping, bilayer design, and interface modification. The following are the consequences on passivation: (1) tuning of the energy level that promotes electron transfer at the ETL/perovskite interface; (2) passivation of the interfacial defects and suppress nonradiative recombination; (3) control of the crystallization process and improvement of the crystallinity of the perovskite film; and (4) endowing devices with improved humidity tolerance and long-term stability.
4.1 Elemental doping
Owing to intrinsic defects such as Sn interstitials or O vacancies, there is serious charge recombination and contact resistance at the interface between SnO2 ETL and perovskite, resulting in a low charge transportation rate.200–203 To suppress the traps on the SnO2 film as well as for better energy-level alignment, the researchers demonstrated several halides and metal ions as dopants to improve the conductivity properties of ETL including the halogen ions (Cl−
204–206 and F−
207–211), metal ions (Li+,212–215 Mg2+,196,216 Al3+,217,218 Zn2+,143,219 Sb3+,215,220,221 Mo2+,222 Ru2+,209,223 Ga3+,224,225 Zr2+,226,227 Nb5+
228–230 and Ta5+
231) and rare earth ions (Y3+,192,232 La3+
233) and Nd3+
165 (Table 2).
Table 2 Representative PSCs based on elemental doped SnO2 ETL
Doped element |
Doping raw material |
Device architecture |
Perovskite |
PCE (%) |
Ref. |
Cl− |
Chloroform-D/2-methoxy ethanol solvent |
FTO/Cl–SnO2/perovskite/spiro-OMeTAD/Au |
(FAPbI3)0.85(MAPbBr3)0.15 |
18.1 |
205
|
F− |
NH4F |
FTO/F–SnO2/perovskite/spiro-OMeTAD/Au |
(FAPbI3)0.85(MAPbBr3)0.15 |
20.2 |
207
|
Li+ |
Li-TFSI |
FTO/SnO2/Al2O3/perovskite/spiro-OMeTAD/carbon |
MAPbI3 |
10.01 |
214
|
Mg2+ |
MgAc·4H2O |
FTO/Mg–SnO2/perovskite/spiro-OMeTAD/Au |
MAPbI3 |
19.21 |
196
|
Al3+ |
Al(NO3)3 |
FTO/Al–SnO2/perovskite/spiro-OMeTAD/Au |
Cs0.05(MA0.17FA0.83)0.95Pb(I0.83Br0.17)3 |
17.66 |
218
|
Zn2+ |
ZnCl2 |
FTO/ZnSnO2/perovskite/CuPc/carbon |
Cs0.05FA0.79MA0.16PbI2.5Br0.5 |
17.78 |
143
|
Sb3+ |
SbCl3 |
ITO/Sb–SnO2/perovskite/spiro-OMeTAD/Au |
Cs0.05(FA0.85MA0.15)0.95Pb(I0.85Br0.15)3 |
20.73 |
221
|
Mo5+ |
MoCl5 |
FTO/Mo–SnO2/perovskite/spiro-OMeTAD/Au |
MAPbI3 |
10.52 |
222
|
Ru2+ |
RuCl3·5H2O |
FTO/Ru–SnO2/perovskite/spiro-OMeTAD/Au |
Cs0.05(MA0.10FA0.90)0.95Pb(I0.90Br0.10)3 |
22.0 |
223
|
Ga3+ |
Ga(NO3)3·6H2O |
ITO/Ga-SnO2/perovskite/spiro-OMeTAD/Ag |
(FAPbI3)0.97(MAPbBr3)0.03 |
18.18 |
224
|
Zr2+ |
ZrCl2O·8H2O |
ITO/Zr–SnO2/perovskite/spiro-OMeTAD/Au |
(FAPbI3)0.85(MAPbBr3)0.15 |
19.54 |
226
|
Nb5+ |
NbCl5 |
FTO/Nb–SnO2/perovskite/spiro-OMeTAD/Au |
FA0.75MA0.2Cs0.05Pb(I0.14Br0.86)3 |
20.5 |
228
|
Ta5+ |
TaCl5 |
ITO/Ta–SnO2/perovskite/spiro-OMeTAD/Au |
Cs0.05(MA0.10FA0.90)0.95Pb(I0.90Br0.10)3 |
20.8 |
231
|
Y3+ |
Y(OC4H9)3 |
FTO/Y–SnO2/perovskite/spiro-OMeTAD/Au |
Cs0.05(MA0.15FA0.85)0.95Pb(I0.85Br0.15)3 |
20.71 |
232
|
La3+ |
LaCl3·5H2O |
FTO/La–SnO2/perovskite/spiro-OMeTAD/Au |
MAPbI3 |
17.08 |
233
|
Nd3+ |
Nd(NO3)3·6H2O |
FTO/Nd-SnO2/perovskite/spiro-OMeTAD/Au |
Cs0.05(MA0.17FA0.83)0.95Pb(I0.83Br0.17)3 |
20.92 |
165
|
Gong and co-workers introduced Cl in SnO2 nanoparticles (SnO2–Cl) with a chloroform-D/2-methoxy ethanol solvent (Fig. 8a).205 The SnO2–Cl film had a hydrophobic surface as the effect of Cl, which increased the grain size of perovskite crystals (Fig. 9). Furthermore, SnO2–Cl as an ETL dramatically reduced the electron trap density and inhibited the charge recombination. In contrast to untreated SnO2 based PSCs, SnO2–Cl based PSCs achieved a PCE of 18.1%, with significant improvements in Jsc and reduced hysteresis. Later, Wang et al. reported F-doped SnO2 (F:SnO2) nanocrystals as the ETL by spin-coating SnCl2·2H2O and NH4F solution onto fresh FTO substrates in a facile solution-processable method at low temperatures (Fig. 8b).207 They found that the band offset between the ETL and the perovskite absorber is related to the F doping level in SnO2 nanocrystals; the band offset was effectively tailored by doping F into the SnO2 film, which led to an increasing built-in electric field for maximizing the Voc and charge collection simultaneously. Consequently, a champion PCE of 20.2% with a Voc of 1.13 V can be obtained for n-i-p planar PSCs using an F-doped SnO2 bilayer ETL.
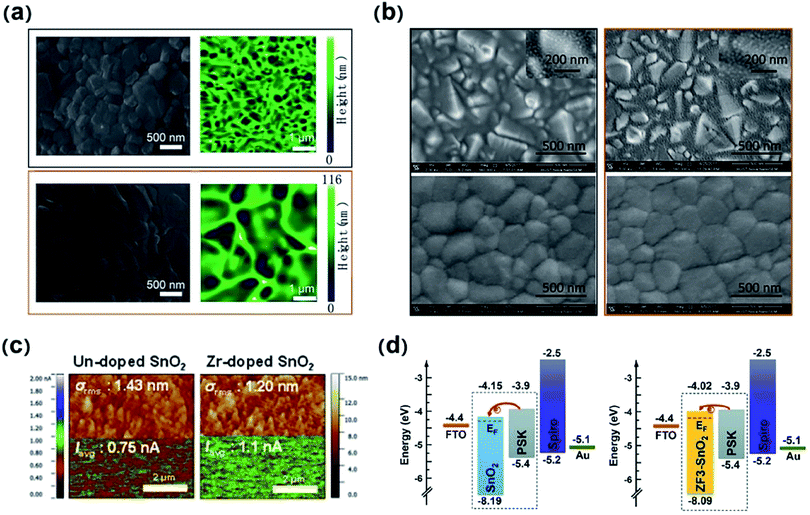 |
| Fig. 8 (a) Top view SEM images and AFM images of perovskite films on (up) FTO/SnO2 and (down) FTO/SnO2–Cl. Reproduced with permission.205 Copyright 2020, Royal Society of Chemistry. (b) SEM images of (left) SnO2 and its perovskite (right) F:SnO2 and its perovskite. Reproduced with permission.207 Copyright 2020, ACS Publishing Group. (c and a) AFM and c-AFM images (bottom) of SnO2 NPs thin films with and without Zr-doping. Reproduced with permission.226 Copyright 2019, Elsevier. (d) Schematic of the energy level arrangement for the pristine SnO2 device and the Zn/F doped-SnO2 device. Reproduced with permission.234 Copyright 2020, Wiley-VCH. | |
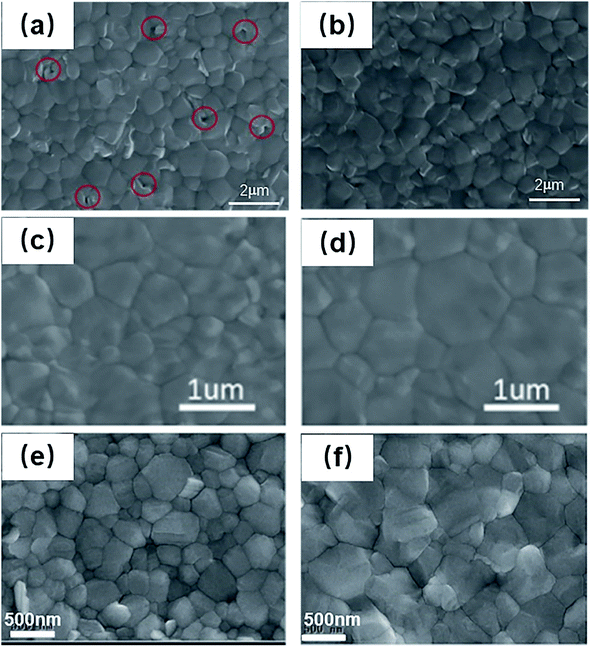 |
| Fig. 9 Top-view SEM images of perovskite films deposited on SnO2 and SnO2/metal oxide ETLs. (a) SnO2 and (b) In2O3/SnO2. Reproduced with permission.245 Copyright 2020, Wiley-VCH. (c) SnO2; (d) WOx/SnO2. Reproduced with permission.246 Copyright 2019, Elsevier B.V. (e) P–SnO2; (f) B–SnO2. Reproduced with permission.247 Copyright 2021, Wiley-VCH. | |
Jung and coworkers reported doped SnO2 NPs by Zr element, improving the multiple optoelectronic properties of SnO2 (Fig. 8c). Zr doping reduced the current leakage and suppressed the charge recombination, facilitating the interfacial transfer between the ETL and the perovskite layer. Consequently, they obtained the best PCE of 19.54% with reduced hysteresis. The research demonstrated that the controlled Zr-doping SnO2 film led to adjusted energy levels, increased electrical conductivities, and decreased surface defect densities for optimal interface properties of PSCs.226 Some other elements also showed similar positive results.209,214,223,231
Fang and co-workers designed a Zr/F co-doped SnO2 ETL due to its low conduction band position and limited intrinsic carriers; they found that the doping of Zr can increase the CB of SnO2 for higher Voc, which decreased the energy traps in electron extraction and restrained the interface recombination between the ETL and the perovskite (Fig. 8d). Moreover, as n-type doping, F doping endowed SnO2 with a mass of free electrons and facilitated the conductivity of the ETL for improvement in short-circuit current (Jsc). With the merits of Zr/F co-doping, the device boosted the PCE by over 19% with free hysteresis, which surpassed the undoped device. This result demonstrated the effect of Zr/F co-doping on the regulation of energy level match and conductivity of SnO2.234
4.2 Bilayer ETLs
A mass of pinholes and cracks appear in the SnO2 film during the fabrication process. The traps reduce the current leakage energy and retard the carrier transfer at the interface, resulting in current leakage in PSCs and a poor photovoltaic performance. Bilayer ETLs based on SnO2 films combined with a thin layer of metal oxide (i.e. TiO2,235,236 Al2O3,214,237 Ga2O3,238 ZnO,239–242 SnO2
122,221 and ZnTiO3
243) or organic transporting materials (PCBM and C60) are used to suppress the interface and bulk defects and charge recombination.
4.2.1 SnO2/metal oxide ETLs.
In n-i-p PSC devices, bilayers of metal oxide composite structures are massively used as ETLs. For example, considering the advantages of the high electron mobility of SnO2 (≈10−3 cm2 V−1 s−1) compared with that of TiO2 (≈10−5 cm2 V−1 s−1) and the high reproducibility of TiO2 ETLs, Mali and co-workers selected SnO2 coated c-TiO2 bilayer ETLs. Owing to its high conductivity, SnO2 occupied the defect sites, which can suppress the surface traps of the TiO2 film, leading to efficient electron extraction from the perovskite absorber. Meanwhile, the high mobility of the SnO2 interfacial layer played a role in maintaining the charge balance of the PSC.244
Wang and co-workers reported a convenient low-temperature process to prepare an In2O3/SnO2 bilayer as the ETL (Fig. 9a and b). The introduction of In2O3 was beneficial to the formation of smooth, dense, and low-defect-density perovskite films. Besides, the CB of In2O3 was lower than that of Sn-doped In2O3 (ITO), facilitating the charge transfer at the interface between perovskite and the ETL, thus reducing the Voc loss. The device with a PCE of over 23% was achieved, and a high Voc of 1.17 V was obtained with the potential loss (0.36 V). Additionally, the PSC retained 97.5% initial PCE after 80 days in a N2 atmosphere without encapsulation and maintained 91% of its original PCE after 180 h under 1 AM illumination.245
Wang and co-workers proposed an amorphous WOx/SnO2 hybrid ETL to effectively block holes through the defects of the SnO2 film to ITO, thereby promoting the charge extraction and reducing the electron–hole recombination at the interface (Fig. 9c and d). Moreover, due to the appropriate energy-level alignment and high conductivity, they also obtained better electron transfer channels. In contrast to flexible PSCs with a single SnO2 ETL, the PSCs with an amorphous WOx/SnO2 hybrid ETL showed a better PCE of 20.52%. Moreover, the WOx/SnO2 preparation process was at a temperature below 150 °C.246
Ye and co-workers proposed a bilayer ETL consisting of two different SnO2 films at a low temperature (70 °C), combined with various amounts of NH4Cl as additives (Fig. 9e and f). The novel SnO2 bilayer tuned the bandgap alignment at the SnO2/perovskite interface and reduced strain in the perovskite film growth, which resulted in negligible carrier recombination, high conductivity, and low voltage loss. Finally, the best device with a doped SnO2 bilayer ETL achieved a photovoltaic performance of 21.75% and strongly increased the Voc up to 1.21 V with negligible hysteresis.247
4.2.2 SnO2/fullerene derivative ETLs.
Fullerene and its derivatives with efficient charge extraction are commonly used as the ETL in p-i-n planar PSCs, including C60 and phenyl-C61-butyric acid methyl ester (PC61BM).157,248–257 Owing to the drawbacks of SnO2 in inefficient charge extraction and interfacial charge recombination, many researchers have tried to deposit the SnO2 film combined with fullerenes or its derivatives as a bilayer to eliminate the issues in achieving efficient and stable PSCs.
Tian and co-workers used 2,5-diphenyl-C60-fulleropyrrolidine (DPC60) to passivate the defects on the SnO2 film surface, forming a SnO2/DPC60 bilayer structure as the ETL in PSCs (Fig. 10a, b and c). DPC60 can reduce charge recombination in the interface of SnO2/perovskite and offer an appropriate band edge alignment, which led to electron transfer from the perovskite absorber to the FTO electrode. As a result, this reveals that electron extraction can be improved via modification on the perovskite/SnO2 interface with (DPC60). The PCE of 20.4% was obtained for PSCs based on the SnO2/DPC60 bilayer, which was higher than that of 18.8% PSCs based on a single SnO2 film.258
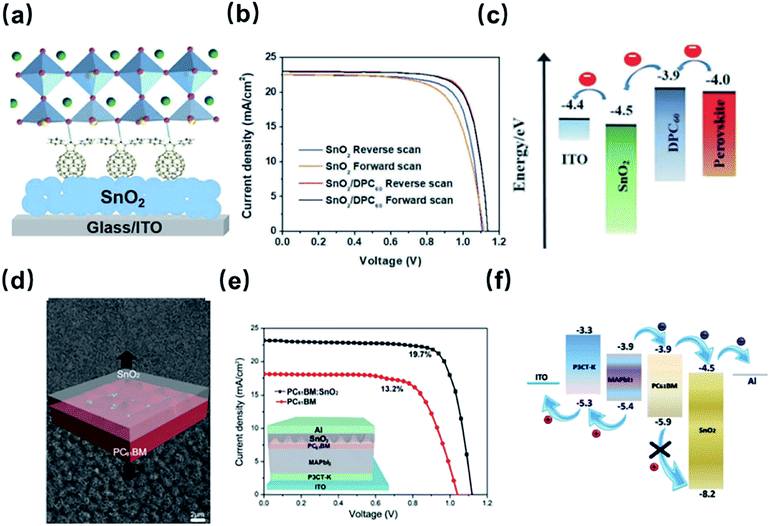 |
| Fig. 10 (a and d) Illustrations of the interfacial treatment. (b and e) Forward and reverse scan J–V curves of devices with and without interfacial modification. (c and f) Energy band diagram of PSCs; (a–c) Reproduced with permission.258 Copyright 2020, Wiley-VCH. (d–f) Reproduced with permission.265 Copyright 2018, ACS Publishing Group. | |
Later on, Raiford and co-workers reported an ultrathin (2 nm) layer of polyethyleneimine ethoxylate (PEIE) to functionalize the surface of C60 for the subsequent preparation of SnO2 by ALD as an electron contact bilayer for p-i-n PSCs. They first used Auger electron spectroscopy (AES) mapping to shed light on the effect of PEIE on SnO2 nucleation in C60. Through a series of testing conditions in the air atmosphere with simultaneous stressors of heat and illumination, improved nucleation contributed to more stable PSCs. These devices got a high initial PCE of 18.5% with 40% improvement in stability over devices with C60/SnO2 contacts without modification with PEIE following a continuous operation at 60 °C unencapsulated in the ambient environment for 250 h.259
In recent, Palmstrom and co-workers investigated the impacts of SnO2 on the perovskite film and explored the role of C60 as an organic electron extractor at the interface. Furthermore, they offered strategies to remove the C60 layer at the perovskite/SnO2 interface.260 Organic extraction layers reduced the mechanical strength and endowed the perovskite/C60 interface with mechanical defects in PSCs.261,262 Owing to the high fabrication cost and poor mechanical stability of C60, PCBM is desirable to reduce the need for the organic layer in future devices.
In addition to regular PSCs, SnO2 is used in inverted PSCs as bilayers with organic transport materials, which preserve efficient electron transport and improve ambient stability. Zhu et al. first used the hydrothermal method to create highly crystalline SnO2 nanocrystals (NCs) that could be used as an efficient ETL in conjunction with C60. The best device, owing to its high crystallinity and hydrophobicity, achieves a high PCE of 18.8% and retains more than 90% of its initial PCE after 30 days of storage in an ambient environment with >70% relative humidity.127 Similarly, Liu used HClO4 to control crystallization during the SnO2 deposition process. Based on the SnO2/C60 bilayer, the unencapsulated inverted PSCs had a champion PCE of more than 16% and retained more than 80% of its initial PCE value after 90 days (>2100 h) of storage in an air atmosphere.263 Luo et al. reported carbon nanotube films coated with SnO2 (SnO2@CSCNT) as ETL in the application of inverted CH3NH3PbI3/NiO PSCs and achieved the best PCE of 14.3% with better stability under a high humidity, thermal stress, or continuous light soaking condition.264
Wang and colleagues used a PC61BM:SnO2 bilayer as the ETL in inverted PSCs and achieved a high PCE of 19.7% (Fig. 10d, e and f). The device with the PC61BM:SnO2 bilayer outperformed the device based on a single PC61BM ETL by 49.0%. The SEM revealed smooth and compact PC61BM:SnO2 layers with minor pinholes and cracks, promoting electron transfer and reducing charge recombination. Furthermore, the device's stability was improved over the PC61BM-only device (Table 3).265
Table 3 Representative PSCs based on bilayer ETLs
Bilayer |
Depositon method |
J
SC (mA cm−2) |
V
oc (V) |
FF (%) |
PCE (%) |
Ref. |
SnO2/In2O3 |
Spin-coating |
24.45 |
1.17 |
87.09 |
23.24 |
245
|
SnO2/a-WOx |
Vacuum thermal evaporation |
23.01 |
1.11 |
80.34 |
20.52 |
246
|
L-SnO2/H–SnO2 |
Spin-coating |
23.6 |
1.21 |
76.2 |
21.75 |
247
|
SnO2/DPC60 |
Spin-coating |
23 |
1.14 |
77.7 |
20.4 |
258
|
SnO2/C60 with PEIE |
Vacuum thermal evaporation |
19.66 |
1.154 |
81.8 |
18.5 |
259
|
SnO2/PC61BM |
Spin-coating |
23.15 |
1.12 |
76 |
19.7 |
265
|
4.3 Interface modification
Although SnO2 has excellent optoelectronic properties, defects or traps appear in SnO2 films because of improper deposition methods, which will become the center of carrier recombination, resulting in decreased PCE. UV treatment is a common way to remove surface impurities or residuals, suppress the oxygen vacancies, and facilitate surface wettability for perovskite film growth.102,266–269 To improve the film quality, reduce the surface defects, and facilitate interfacial contact, many researchers introduced a series of materials to passivate interfacial defects and promote the conductivity of the SnO2 film, which are all very significant to achieve an efficient and stable PSC device, including ammonium salts, quantum dots, self-assembled monolayers, 2D materials, and carbon materials.
4.3.1 Ammonium salts.
Despite its high electron mobility, SnO2 ETL deposited at a low temperature suffers from poor film crystallinity and large defect density, which usually reduce the PSC efficiency. Ammonium salts are introduced for SnO2 surface modification to reduce the interfacial defects.97,247,270,271
Chen and co-workers introduced Girard's Reagent T (GRT) into the SnO2 NP colloidal solution and obtained a high efficiency of 21.63% with free hysteresis. The AFM and contact angle test results showed better roughness and wettability for the SnO2 film with GRT modification due to the chemical interaction between GRT and SnO2 NPs (Fig. 11a and b). As a result, the quality of perovskite films with GRT modification was also improved. Many holes could be effectively eliminated in the perovskite film compared with the control ones. The vertically oriented large grains were across the whole cross-section, which confirmed that GRT modification is beneficial to the SnO2 NP dispersion and the interfacial contact between the ETL and the perovskite layer.97
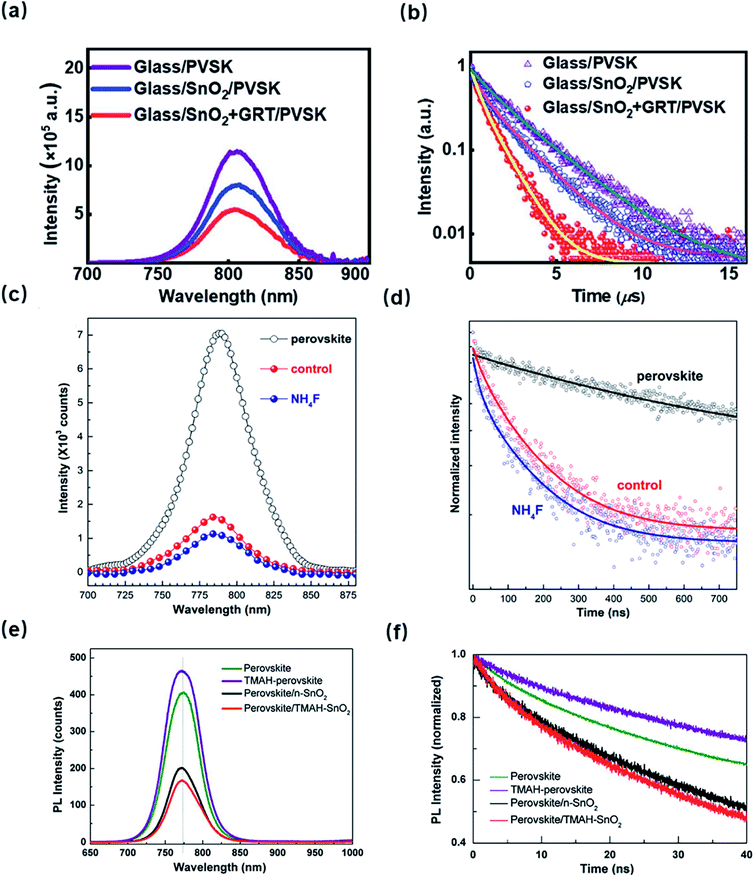 |
| Fig. 11 Steady-state PL spectra of the perovskite film deposited on: (a) SnO2 and GRT-SnO2, (c) SnO2 and NH4F–SnO2, (e) SnO2 and TMAH-SnO2. Normalized time-resolved PL: (b) SnO2 and GRT-SnO2 ETLs, (d) SnO2 and NH4F–SnO2 ETLs, and (f) SnO2 and TMAH-SnO2. (a and b) Reproduced with permission.97 Copyright 2021, Royal Society of Chemistry. (c and d) Reproduced with permission.272 Copyright 2020, ACS Publishing Group. (e and f) Reproduced with permission.273 Copyright 2018, Royal Society of Chemistry. | |
Jung and co-workers used ammonium fluoride (NH4F) to modify the SnO2 surface to eliminate trap sites and change the Fermi level of SnO2 thin films (Fig. 11c and d). They suggested that an ammonium cation in NH4F generated ammonia gas and water vapor due to the reaction with hydroxyls on the SnO2 surface. Besides, fluoride anions would get inserted into the trap sites, resulting in an adjustment of the energy level. PSCs with treated SnO2 achieved a champion performance of 23.2%.272
Huang et al. showed that the addition of the IL tetramethylammonium hydroxide N(CH3)4OH (TMAH) to the SnO2 nanoparticle suspension to form a stable and homogeneous suspension could result in a better SnO2 ETL with lower defect density and higher conductivity for a better FF (Fig. 11e and f).273 As a result, TMAH-SnO2-based PSCs showed a higher PCE (20.28%) than that of non-treated SnO2-based PSCs (18.14%). Ethylenediaminetetraacetic acid (EDTA) is introduced onto SnO2 to increase the electron mobility of the ETLs, resulting in a PCE of 21.60% and a certified PCE reaching 21.52%.274
4.3.2 Quantum dots (QDs).
The quantum dots (QDs) had a tunable bandgap together with the quantum confinement and edge effects, which were novel promising zero-dimensional materials to decorate the SnO2 surface to promote the electron conduction of SnO2.103,275
Chen and co-workers developed a SnO2/graphitic carbon nitride quantum dot (g-C3N4 QD) nanocomposite (G-SnO2) as the functional ETL to accurately control the interfacial charge dynamics of efficient n-i-p planar PSCs (Fig. 12a, b and c). The g-C3N4 QDs could suppress the oxygen-vacancy-induced traps and facilitate the interfacial charge transfer by redistributing the electronic density around the neighboring SnO2 crystal unit, resulting in enhanced electrical properties, appropriate bandgap matching and high electron conduction. Employing the SnO2/g-C3N4 QDs-based ETL, a champion PCE of 22.13% can be obtained with negligible hysteresis and long-term stability.276
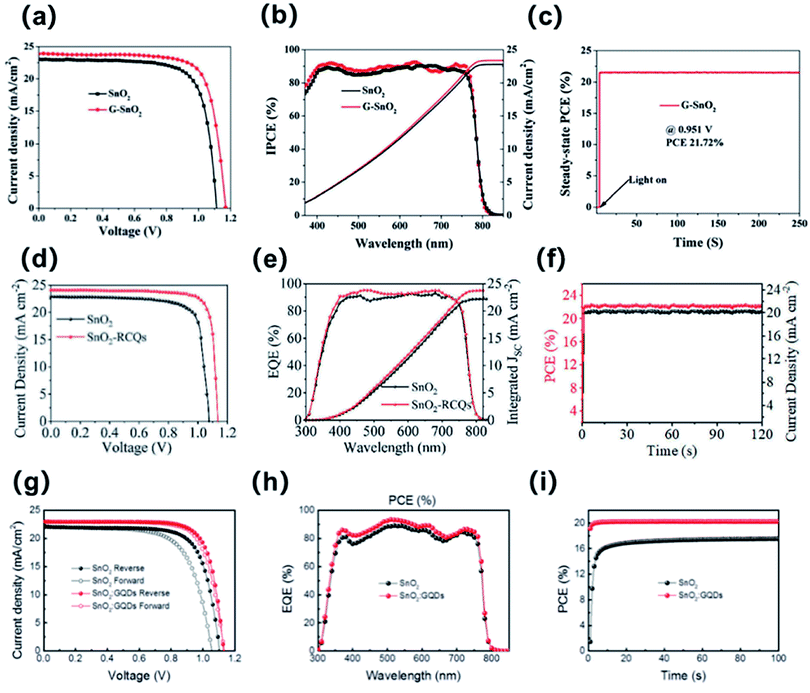 |
| Fig. 12
J–V curves of perovskite solar cells based on (a) pristine and g-C3N4 QD-treated SnO2 ETLs; (d) pristine and RCQ-treated SnO2 ETLs; (g) pristine and G QD-treated SnO2 ETLs. The corresponding IPCE spectra of (b) pristine and g-C3N4 QD-treated SnO2 ETLs; (e) pristine and RCQ-treated SnO2 ETLs; and (h) pristine and GQD-treated SnO2 ETLs. Steady efficiency at the maximum power point of (c) pristine and g-C3N4 QD-treated SnO2 ETLs; (f) pristine and RCQ-treated SnO2 ETLs; (i) pristine and GQD-treated SnO2 ETLs. (a–c) Reproduced with permission.276 Copyright 2020, Royal Society of Chemistry. (d–f) Reproduced with permission.277 Copyright 2020, Wiley-VCH. (g–i) Reproduced with permission.269 Copyright 2017, ACS Publishing Group. | |
Hui and co-workers recently reported carboxylic-acid- and hydroxyl-rich red-carbon quantum dot (RCQ) modified-SnO2 with a suitable band alignment (Fig. 12d, e and f). The calculated electron mobility of SnO2-RCQs by using the space charge-limited current (SCLC) model was increased by 2 orders of magnitudes, resulting in a significant reduction in the conductivity of SnO2-RCQs. Simultaneously, the RCQ modified SnO2 layer not only passivated the ETL/perovskite interface but also facilitated the crystallinity of the perovskite film, with dense and smooth morphology over a large area. This device with modified SnO2 obtained an outstanding efficiency of 22.77% with negligible hysteresis and showed long-term stability against moisture, maintaining over 95% of the original PCE value after 1000 h storage in an ambient environment.277
Xie and co-workers added graphene quantum dots (GQDs) to the SnO2 precursor to form a SnO2:GQDs layer as the ETL by a simple spin-coating method (Fig. 12g, h and i). It was found that GQDs effectively promoted electron transport and passivated the electron traps at the interface, which led to improved electron extraction rate and reduced charge accumulation at the ETL/perovskite interface. As a consequence, the best device based on SnO2:GQDs ETLs exhibited a PCE of 20.23% and a free hysteresis effect.269
4.3.3 Self-assembled monolayers (SAMs).
Like commonly used metal oxides such as TiO2, SnO2, ZnO, MoOx, or organic charge transport moleculars such as PC61BM and poly(3,4-ethylenedioxy-thiophene)polystyrene (PEDOT:PSS), SnO2 has hydroxyl groups at the surface of the film, and they cause defects states near the valence band, which can induce a nonradiative recombination at the SnO2/perovskite interface.278 Recently, owing to chemical bonding, self-assembled monolayer (SAM) ordered arrays of organic molecules formed by the spontaneous absorption onto a surface of molecular constituents from a vapor or liquid phase, are introduced to modify the SnO2 surface, which is beneficial to tuning the energy level, regulating the WF at the surface and promoting the charge collection.49,279–283 Besides, SAMs can reduce the vacancies and crystal traps at the perovskite, resulting in high-quality films and improved performance and stability of PSCs.
Yan and co-workers reported a choline chloride SAM on the surface of SnO2 (chol-SnO2) by chemically reacting with the perovskite film to eliminate oxygen vacancies at the interface and lengthen the carrier lifetime (Fig. 13a and d). Finally, the device based on SnO2 modified by choline chloride achieved a champion photovoltaic performance of 18.90% with free-hysteresis and a high Voc (1.145 V).284
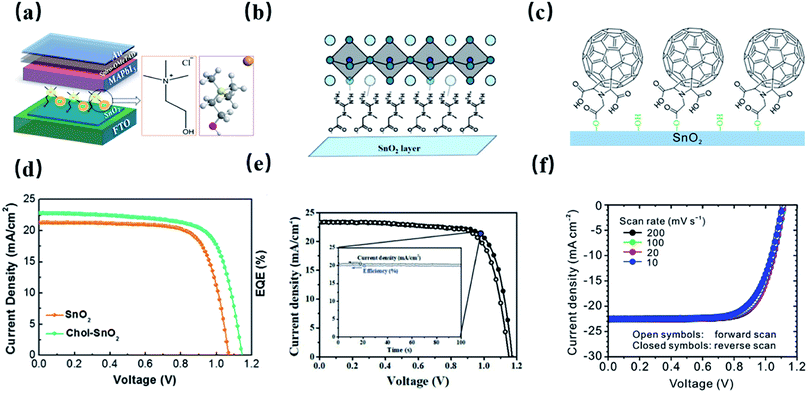 |
| Fig. 13 Schematic illustration of the formation of various self-assembled monolayers adhering to SnO2 layers and J–V curve of devices with different SAM treatments. (a and d) Choline chloride treatment. Reproduced with permission.284 Copyright 2020, ACS Publishing Group. (b and e) 2-[Carbamimidoyl(methyl)amino] acetic acid treatment. Reproduced with permission.285 Copyright 2015, Royal Society of Chemistry. (c and f) C60-pyrrolidine tris-acid treatment. Reproduced with permission.287 Copyright 2020, Nature Publishing Group. | |
Kim and co-workers employed 2-[carbamimidoyl(methyl)amino]acetic acid (creatine), one of the standard amino acids in the human body, on a SnO2 layer (Fig. 13b and e). Given the chemical structure, creatine can anchor on the surface of SnO2 by a carboxylic acid functional group and had high polarity, leading to an increase in the charge extraction efficiency. The formamidinium-based perovskite PSC with modified SnO2 achieved the optimal efficiency of 22.1% and maintained 90% initial performance even after 50 days.285
Anizelli and co-workers performed a systematic study of the application of two special SAMs, ethylphosphonic acid (EPA) and 4-bromobenzoic acid (BBA), onto SnO2 and the NiO2 ETL for stability. A series of stability tests of films and devices with and without SAM layers, at a working-condition temperature of 75 °C and continuous illumination, were designed. They demonstrated a distinct evolution of perovskite thin films with irradiation time by adopting SAMs, and SAMs also generated several changes in the photoelectric parameters of the devices in view of their chemical propertie and their combination with the SnO2 and NiO2 layers. At last, SAMs exhibited a significant effect on the lifetime of PSCs, extending up to five times that of the device's T50% (time for the efficiency to reach half of its initial value) in some cases.286
Hysteresis and degradation occurring in traditional PSCs structures with SnO2 as the ETL has not been well understood. Tumen-Ulzii and co-workers modified the SnO2 surface by a C60 pyrrolidine tris-acid (CPTA) SAM because of the ability to form a strong chemical interaction with the SnO2 surface (Fig. 13c and f). Furthermore, the SAM can effectively deactivate these hydroxyl groups, which localized the positive ions, leading to the hysteresis and degradation in PSCs. After surface treatment, they obtained free-hysteresis and long-term stability PSCs with no degradation after 1000 h of continuous light incidence.287 Although PCBM or the C60 buffering layer has the merits of suppressing hysteresis behavior and reducing the interfacial charge recombination loss, we do not ignore the drawbacks of difficulties in depositing them. For buffering layers, thermal deposition can precisely control its thickness, but it takes more cost and energy consumption to deposit them in a vacuum environment. On the other hand, solvent orthogonality is required to be solved because the polar solvents used in perovskite precursors like dimethylformamide (DMF) and dimethyl sulphoxide (DMSO) destroy the perovskite crystal structures.
4.3.4 Two-dimensional materials.
Recently, two-dimensional (2D) materials such as graphene, MXenes, and metal sulfides have been commonly introduced into SnO2 based on PSCs owing to their unique photoelectric properties.72,77,288–291 MXenes are promising 2D materials that process high electron mobility and less light absorption in the visible range. Their general chemical formula is Mn+1XnTx, in which M displays a type of early transition metal, X usually is a carbon and/or a nitrogen atom, and T is the surface termination group (usually oxygen- and/or fluorine-containing groups).292–294 Interestingly, the application of MXenes has been reported in PSCs as additives in the perovskite layer and ETLs.291,295,296
Wang and co-workers used Ti3C2Tx MXene to increase the conductivity and the charge collection ability of the SnO2 ETL. The MXene-modified SnO2 ETL also led to a preferable growth of perovskite films with free defects. Consequently, the PSCs obtained the best photovoltaic performance of 20.65% with minimal hysteresis.290
Yang and co-workers introduced Ti3C2 (the most widely studied MXene) nanosheets into SnO2 precursors to increase the ETL conductivity via a low-temperature method (Fig. 14a, b and c). Through optimizing the Ti3C2 contents, the device with the perovskite film of MAPbI3 obtained 18.34%, which was much higher than that of only SnO2-based PSCs. The enhancement of the PCE can be ascribed to good bandgap alignment, leading to faster charge extraction from the perovskite layer.291
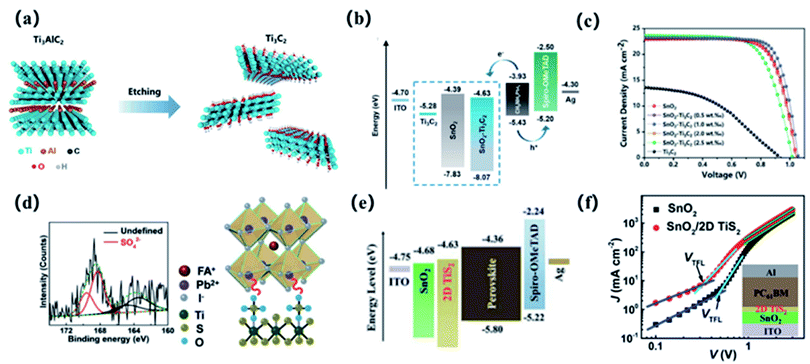 |
| Fig. 14 (a) Schematic diagram of Ti3C2 formation. (b and e) Energy level diagram of the components in the semiconductor hybrid perovskite. (c) Backward scan J–V curves of devices with a SnO2 ETL and with SnO2–Ti3C2 ETLs at various Ti3C2 concentrations. (d) High-resolution XPS spectra of S 2p on the SnO2–TiS2 film; working mechanism of 2D TiS2 interaction with the perovskite crystal; (f) J–V curves of electron-only devices with the inset illustrating the device structure. (a–c) Reproduced with permission.288 Copyright 2019, Royal Society of Chemistry. (d–f) Reproduced with permission.291 Copyright 2019, Royal Society of Chemistry. | |
Some metal sulfide materials are also good choices to modify the SnO2 for enhanced electron conductivity. Huang and co-workers spin-coated the 2D TiS2 layer on the top of SnO2 as a bilayer ETL (Fig. 14d, e and f). 2D TiS2 can suppress trap sites of SnO2 and change the energy level alignment, resulting in improved electron mobility. The highest PCE reached was 21.73% with free hysteresis.288
4.3.5 Carbon materials.
Carbon-based materials are suitable charge-transfer materials for commercializing large-scale PSCs due to low-expense, high electron conduction, especially low-temperature fabrication (100 °C),297,298 and similar work function as gold (5.0 and 5.1 eV, respectively).297 Besides, they possess a highly hydrophobic nature, which protect moisture infiltration into the perovskite layer, resulting in long-term stability without encapsulation.299 Nowadays, carbon-based materials including graphene, carbon nanotubes (CNTs), and carbon or graphene nanodots are widely used in PSCs as additives in perovskite precursors, interlayers between ETL or HTL with the perovskite layer and cathode.300–305
Tang and co-workers prepared SnO2 and carbon nanotubes (CNTs) hybrid ETL (SnO2–CNT) by spin-coating method.116 Besides, carbon nanotubes (CNTs) were modified by oxidative treatment and then dispersed well in SnCl4·5H2O solution (Fig. 15a and b). As a consequence, the best PCE of 20.33% was obtained with negligible hysteresis, which was attributed to the significant increase in the conductivity of SnO2 films and decrease in the trap-state density of SnO2 films. Wang and co-workers firstly introduced carbon nanodots (CNDs) into SnO2 ETLs by a facile solution method. CNDs could effectively reduce the defect density and promote electron mobility in SnO2. Finally, the device with ITO/SnO2: CNDs/perovskite/spiro-OMeTAD/Au structure obtained a high power conversion efficiency (PCE) of over 20% with free hysteresis. Additionally, the SnO2: CNDs device dropped only 10% of the initial PCE after storage in ambient for 1200 h and showed better UV stability after continuous UV illumination.306
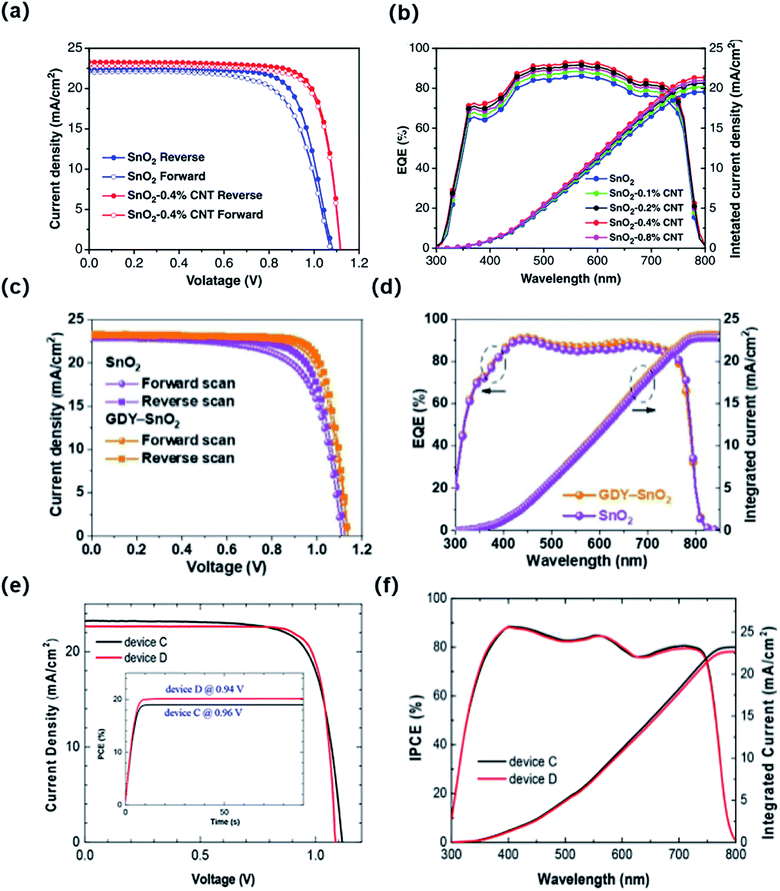 |
| Fig. 15
J–V curves of (a) SnO2 and CNT-SnO2 ETLs; (c) SnO2 and GDY-SnO2 ETLs; (e) SnO2 and graphene-SnO2 ETLs. EQE spectra of (b) SnO2 and CNT-SnO2 ETLs. (d) SnO2 and GDY-SnO2 ETLs. (f) SnO2 and graphene–SnO2 ETLs. (a and b) Reproduced with permission.116 Copyright 2020, Wiley-VCH. (c and d) Reproduced with permission.307 Copyright 2020, Wiley-VCH. (e and f) Reproduced with permission.308 Copyright 2018, ACS Publishing Group. | |
Zhang and co-workers firstly introduced graphdiyne (GDY) as a novel carbon material to optimize the charge collocation process of SnO2 ETL, tune the growth of perovskite and eliminate interfacial defects towards both perovskite crystallization process and subsequent photovoltaic service duration. Through the GDY modification, the PCE of over 21% was achieved with 10% improvement together with free hysteresis. It is demonstrated that GDY treated SnO2 layer improved electron mobility and more facilitated energy level alignment (Fig. 15c and d). Moreover, the improved interfacial hydrophobicity effectively impeded excess heterogeneous perovskite nucleation, leading to the higher crystal grain size of perovskite, fewer grain boundaries, and decreased trap density.307
Zhao and co-workers treated SnO2 nanocrystals by chemically modified graphene as ETL for highly efficient and stable PSC (Fig. 15e and f). Besides, they also reported a creative strategy to disperse graphene in an aqueous solution that can preserve any residue on the SnO2 film. Finally, the best device showed a PCE of over 20% with a high fill factor of up to 82%, which resulted from the enhanced conductivity (Table 4).308
Table 4 Representative PSCs with interface modification
|
Passivation materials |
J
sc (mA cm−2) |
V
oc (V) |
FF (%) |
PCE (%) |
Ref. |
Ammonium salts |
Girard's reagent T |
22.92 |
1.146 |
82.3 |
21.63 |
97
|
Ammonium salts |
NH4F |
24.60 |
1.16 |
81.4 |
23.2 |
272
|
Ammonium salts |
N(CH3)4OH |
22.51 |
1.14 |
79 |
20.28 |
273
|
Ammonium salts |
EDTA |
24.57 |
1.11 |
79.2 |
21.6 |
274
|
QDs |
g-C3N4 |
23.03 |
1.176 |
78.3 |
22.13 |
276
|
QDs |
RCQ |
24.10 |
1.14 |
83 |
22.77 |
277
|
QDs |
GQD |
23.05 |
1.134 |
77.8 |
20.31 |
269
|
SAMs |
Choline chloride |
22.80 |
1.145 |
72.41 |
18.90 |
284
|
SAMs |
Creatine |
23.4 |
1.19 |
75.9 |
20.8 |
285
|
2D materials |
Ti3C2Tx |
24.34 |
1.11 |
76.4 |
20.65 |
290
|
2D materials |
Ti3C2 |
23.14 |
1.06 |
75 |
18.34 |
291
|
2D materials |
TiS2 |
24.57 |
1.11 |
79.4 |
21.73 |
288
|
Carbon materials |
CNDs |
23.26 |
1.12 |
78.23 |
20.33 |
116
|
Carbon materials |
CNDs |
23.14 |
1.10 |
79 |
20.03 |
306
|
Carbon materials |
Graphdiyne |
23.32 |
1.137 |
79.62 |
21.11 |
307
|
Carbon materials |
Graphene |
22.66 |
1.084 |
82.1 |
20.16 |
308
|
5. Hysteresis and stability
The severe hysteresis and inferiority in the stability of PSCs under operation conditions are urgent challenges that quietly inhibit the PSCs from being used in the commercialization of laboratory products.
5.1 Hysteresis suppression
The presence and behavior of hysteresis, the difference in the I–V curve from the reverse scan (open-circuit to short-circuit) and the forward scan (short-circuit to open-circuit), is a fundamental problem in the perovskite solar cell field. This results in obstacles to the progress of the actual performance of the devices in operation. Compared with devices with an n-i-p structure with a scaffold and an inverted p-i-n structure with a fullerene ETL, PSCs with the regular planar structure show much more evidence of the hysteresis effect.
Although the reasons for the appearance of hysteresis in PSCs are not evident, energy defects at the interfaces, the grain boundary in the perovskite film, and capacitive and ferroelectric polarization possibly affect the hysteresis behavior.309–312 Recently, it seems that hysteresis is induced by ion migration, which caused charge accumulation, unbalanced charge mobility, defects or traps in the instinct of perovskites and perovskite/ETL interfaces.313–317 As mentioned above, various types of device structures exhibited different hysteresis behaviors. The contact between the perovskite and charge transport layers is the main factor within these other structures, suggesting that the contact could also influence the hysteresis.318
Compared with many other metal oxides as ETLs, SnO2 has the merits of a more bottomless conduction band, higher conductivity, and electron mobility, which is beneficial to charge transfer from perovskite to ETL low charge accumulation at the interface. To date, devices based on the SnO2 ETL in planar PSCs show a little hysteresis effect than many common metal oxides as ETLs, especially the TiO2 ETL.319 For PSCs with n-i-p planar structures, SnO2 is an ideal electron transport material as the ETL to almost solve the hysteresis problem. As previously mentioned, SnO2 combined with doped elements,320 bilayers,247,321,322 and interfacial engineering323,324 can retard the formation of the defect states, which improve the carrier transport performance, leading to negligible hysteresis in the device.
Tumen-Ulzii and co-workers treated the SnO2 surface with a self-assembled monolayer and achieved a hysteresis-free PCE over 18% (Fig. 16). It was found that hydroxyl groups existing at the SnO2 surface induced positive ion localization, resulting in hysteresis and degradation. Interestingly, a self-assembled monolayer of a fullerene derivative (CPTA-SAM) can effectively deactivate these surface –OH groups. The temperature and scan speed can affect the hysteresis. Besides, with temperature increase or scan speed decrease, more significant hysteresis is apparent in J–V curves. PSCs with CPTA-SAM treatment significantly reduced the J–V hysteresis of PSCs at different scan speeds in contrast to devices without CPTA-SAM treatment. Furthermore, negligible hysteresis was observed in PSCs with the CPTA-SAM even at a high temperature of 60 °C.287
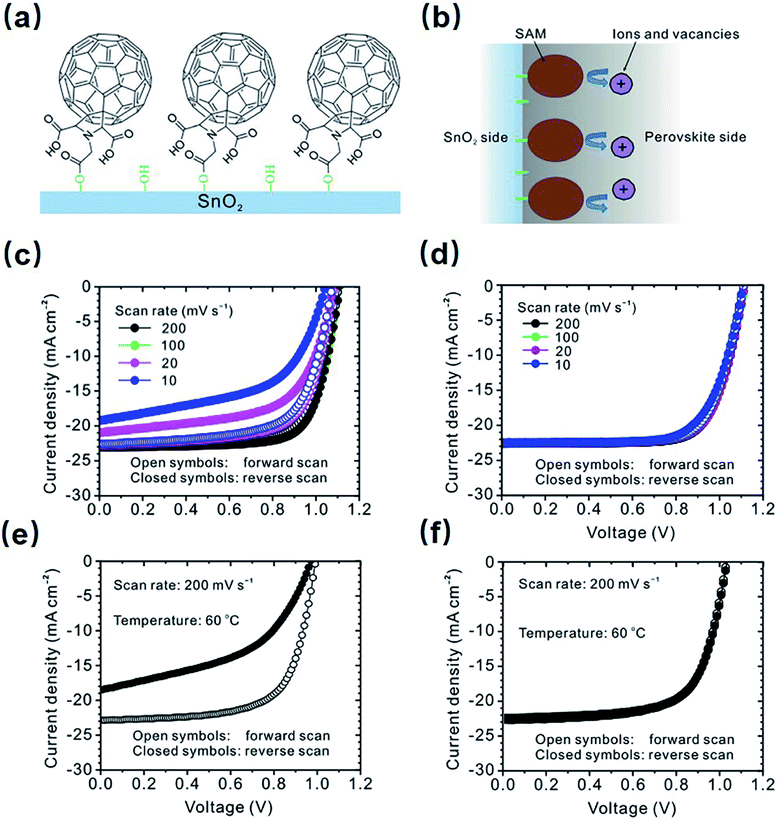 |
| Fig. 16 Positive effect of using a self-assembled monolayer (SAM) on the hysteresis and carrier recombination characteristics in perovskite solar cells. (a) Mixed self-assembled monolayers adhering to the SnO2 substrates and (b) schematic illustration of reducing localized ionic charges by eliminating –OH groups on the surface; backward and forward scan J–V curves at different scan speeds: (c) untreated devices and (d) devices with SAM treatment; backward and forward scan J-V curves measured at a fast scan rate of 200 mV s−1 at 60 °C: (e) untreated devices and (f) devices with SAM treatment. Reproduced with permission.287 Copyright 2020, Nature Publishing Group. | |
Ye and co-workers spin-coated KCl and NaCl on the SnO2 surface to suppress the hysteresis in flexible PSCs.325 The KCl and NaCl treatments effectively improve the power output of flexible PSCs. However, devices with NaCl modification showed a profound hysteresis effect in J–V curves. It suggested that the hysteresis relied strongly on alkali metals. With the increase of the ionic radius of alkali metal ions from Li+ to K+ and then to Cs+, the hysteresis effect first gradually decreased and disappeared, and then appeared again and increased, which was strongly related to the trapping density.326,327 In addition, it can reduce the device hysteresis to use a suitable SnO2 fabrication process such as ALD, CBD, etc.
5.2 Device stability
5.2.1 Ambient stability.
SnO2 is more stable in oxygen and moisture than TiO2, an ideal alternative to the TiO2 ETL for device stability.188,328,329 Zhang and co-workers found that using poly(vinylpyrrolidone)-doped SnO2 as the ETL, 88% of its highest PCE is retained even after 41 days of storage without encapsulation.330 Huang and co-workers also revealed that the SnO2 ETL modified with polymer ethoxylated polyethylenimine (PEIE) indicated significant storage device stability, and the device with SnO2:PEIE ETL preserved 82.1% of its original PCE (PCE¼, 16.89%) after 70 days of storage.331 Wang and co-workers designed TiO2/SnO2 bilayer as the ETL in CsPbI2Br all-inorganic perovskite, and the devices showed superior photovoltaic properties, particularly excellent phase and thermal stability. After one month of storage in an N2 environment without any encapsulation, the PCE can retain about 95% of the original efficiency (Fig. 17a and b).332
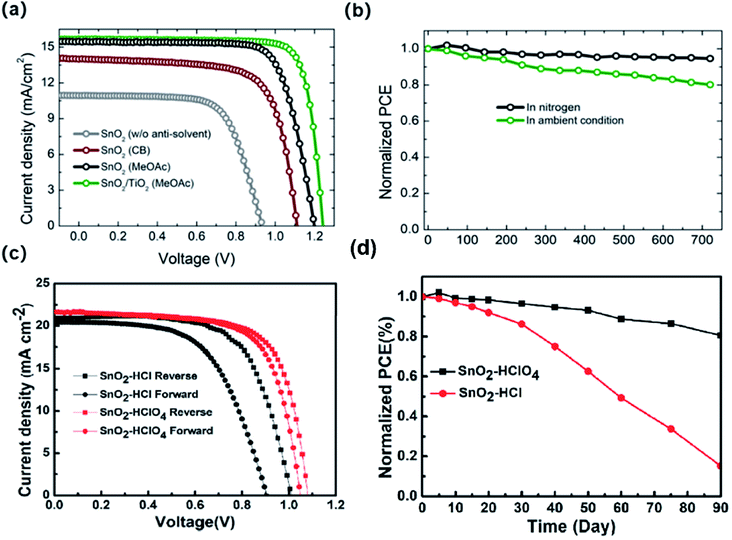 |
| Fig. 17
J–V curves of devices based on (a) SnO2 and TiO2/SnO2 ETLs and (c) HCl–SnO2 and HClO4–SnO2 ETLs. Stability test of devices on (b) SnO2 and TiO2/SnO2 ETLs in an N2 – filled glovebox and under ambient conditions (@R.T., 20–30% relative humidity) (d) HCl–SnO2 and HClO4–SnO2 ETLs under the humidity of 55% ± 10% at 85 °C in open air. (a and b) Reproduced with permission.332 Copyright 2020, ACS Publishing Group. (c and d)Reproduced with permission.263 Copyright 2020, Elsevier. | |
Apart from SnO2 as ETLs in regular structures, SnO2 can be introduced as a protecting layer on the top of the perovskite layer or fullerene layer.87,127,265,333,334 Wang and co-workers used SnO2 nanocrystals combined with HClO4 and HCl treatment as a buffer layer, inserting it into the C60/Ag electrode interface (Fig. 17c and d). The devices with the HClO4–SnO2 ETL without encapsulation increased the PCE up to 16.36% with almost negligible hysteresis and maintained over 80% of their highest PCE after storage in open air for 90 days (>2100 h) and reduced by only about 20% its initial efficiency after 41 h under heating at 85 °C.263
5.2.2 Light illumination stability.
TiO2 is a common metallic oxide used as the ETL, which induces decomposition of PSC under UV illumination owing to their photocatalytic activity. Due to the efficiency and stability degradation, there is a mass of oxygen vacancies in the TiO2 lattice, which can be generated by UV illumination.200 The oxygen vacancies cause deep trap states, interacting with molecular oxygen in ambient air, and then cause deep trap sites, which can interact with molecular oxygen in the environment by adsorption, leading to their passivation.41,335,336 When deep traps are excited by oxygen desorption, the charge recombination easily occurs at the interface, resulting in a PCE loss. Besides, TiO2 is able to obtain excess electrons from perovskite because of its high photochemical activity, which can cause photo-decomposition of the perovskite crystals, particularly in the humid environment.337–339
Liu and co-workers studied the reasons for the degradation process of the perovskite layer deposited on different ETLs (PCBM, TiO2, and SnO2) under strong ultraviolet irradiation. Although the SnO2 layer reduced light-induced chemical activity in contrast to the TiO2 layer, the serious decomposition of perovskite observed at the perovskite/SnO2 interface, together with the formation of hole structures, decreases the carrier transfer at the interface, owing to the separation of the perovskite absorber from ETLs and thus rapidly decreased the device performance (Fig. 18b).340 Wang and co-workers used carbon nanodots (CNDs) to dope SnO2 as the ETL and found that SnO2:CNDs based PSCs achieved an efficiency over 20% with nearly free hysteresis and maintained over 90% of the initial PCE in a N2 atmosphere for 1200 h and showed better UV stability under UV illumination for 200 h.306
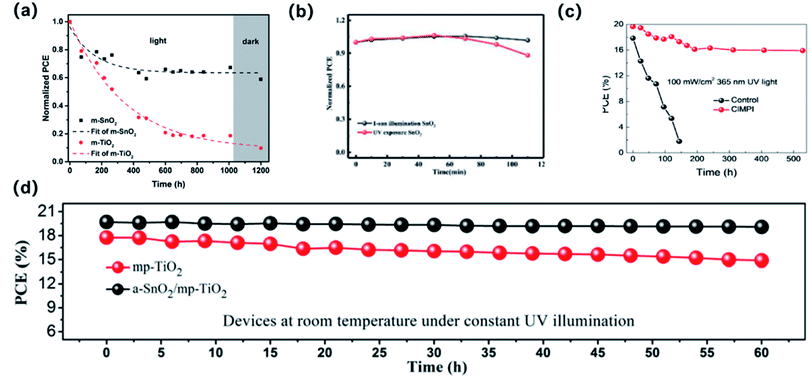 |
| Fig. 18 UV light aging test of devices based on (a) m-SnO2 and m-TiO2 under 100 mW cm−2 illumination in a N2 atmosphere; reproduced with permission.339 Copyright 2018, Royal Society of Chemistry. (b) mp-SnO2 under one sun illumination and UV exposure in a N2 atmosphere. Reproduced with permission.340 Copyright 2020, Royal Society of Chemistry. (c) ClMPI-SnO2 reproduced with permission.341 Copyright 2019, Elsevier B.V. (d) mp-TiO2 and SnO2/mp-TiO2. Reproduced with permission.342 Copyright 2018, ACS Publishing Group. | |
Similarly, Abate and co-workers reported that Ga doping could improve UV-resistant mesoporous SnO2. When SnO2 and TiO2 based PSCs were exposed to continuous light illumination for 1000 h, both devices exhibited a rapid decrease to 80% in the first 100 h (Fig. 18a). After this, SnO2 based PSCs retained around 70% of the original efficiency up to 1000 h of full-spectrum illumination. In contrast, the normalized PCE of TiO2 based PSCs dropped rapidly and stabilized only 20% of the initial PCE after illumination.339
Wang and co-workers introduced a chlorine-rich perovskite interlayer (ClMPI) to modify the SnO2 ETL/perovskite interface through halide exchange to improve the interfacial charge transfer (Fig. 18c). Encouragingly, the ClMPI-based PSC still maintained over 82% of the initial PCE under UV exposure with a high power of 100 mW cm−2 after 500 h.341
More encouragingly, Gratzel and co-workers designed a bilayer ETL of an amorphous SnO2 coated TiO2 scaffold (mp-TiO2) layer, and they displayed that the devices with a TiO2/SnO2 bilayer ETL rendered them more resistant to UV light than devices with only mp-TiO2 as a single ETL (Fig. 18d).342
5.2.3 Heat stability.
In addition to light and humidity, thermal treatment is also the cause of poor stability problems of perovskite solar cells. The temperature of the cells can increase under exposure to sunlight, resulting in accelerated degradation, especially for MA-based perovskite solar cells.343 Some strategies were reported, such as designing 2D/3D heterojunctions, using a green antisolvent, interface engineering, and additive engineering for SnO2-based perovskite solar cells.344–350
Chen and coworkers deposited 4-imidazoleacetic acid hydrochloride (ImAcHCl) on the top of the SnO2 film, leading to a chemical bridge between SnO2 and perovskite through an ester bond with SnO2 (Fig. 19a). As a result, ImAcHCl could improve the perovskite crystallization, suppress the nonradiative recombination, and the promote carrier lifetime. Moreover, an unencapsulated device with ImAcHCl-modified SnO2 retained 90% of its initial after 40 h of aging at 80 °C, while PCE was degraded by 53% for the control device.344
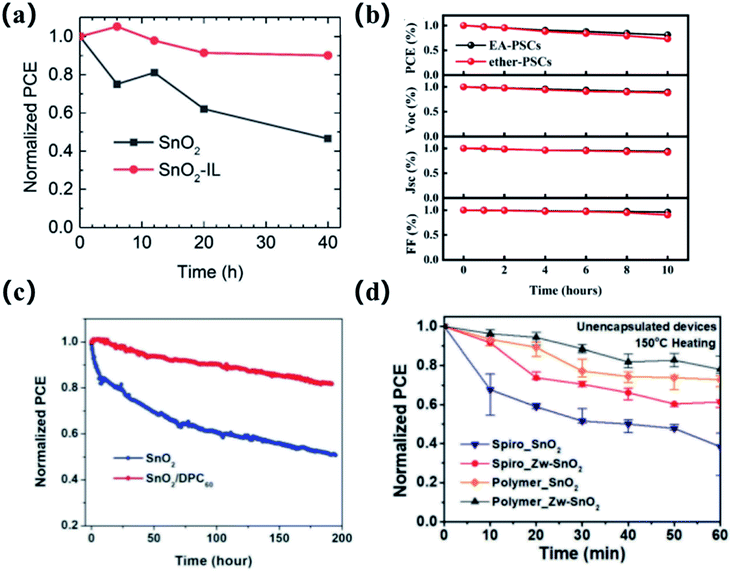 |
| Fig. 19 Device thermal stability of (a) unencapsulated devices at 85 °C in the dark in the glovebox. Reproduced with permission.344 Copyright 2019, Wiley-VCH. (b) Unencapsulated devices at 100 °C in air. Reproduced with permission.349 Copyright 2020, Elsevier. (c) MPP tracking of the unencapsulated devices at (55 ± 5 °C) in a N2 atmosphere. Reproduced with permission.348 Copyright 2020, Wiley-VCH. (d) Unencapsulated devices at 150 °C in air. Reproduced with permission.345 Copyright 2018, Royal Society of Chemistry. | |
Zhang and coworkers used ethyl acetate (EA) as a green antisolvent to control the perovskite crystallization process, resulting in smooth and dense perovskite films with free pinholes (Fig. 19b). In the ambient atmosphere, the unencapsulated device maintained about 85% of its initial PCE after more than 1900 h of storage. Meanwhile, the device also showed remarkable thermal stability, retaining 81.03% of the original PCE value at 100 °C for 10 h.349
Interface engineering between SnO2 and the perovskite layer is a common way to improve thermal stability. Tian and coworkers synthesized 2,5-diphenyl C60 fulleropyrrolidine (DPC60) as an interfacial layer between perovskite and SnO2 in planar perovskite solar cells (PSCs) (Fig. 19c). As a result, PSCs modified with DPC60 obtained a PCE of 20.4% with high reproducibility. Furthermore, the device retained 82% of its initial efficiency after 200 h of 1 sun continuous irradiation and thermal aging (55 ± 5 °C) due to the suppression of heterogeneous nucleation and improvement in the crystallinity of the perovskite film.348 Similarly, Choi and coworkers exhibited a PCE of 21.43% by incorporation of SnO2 with a zwitterionic compound (3-(1-pyridinio)-1-propane sulfonate), which led to improved PCE and thermal stability (Fig. 19d).345
6. Commercialization and applications
6.1 Flexible perovskite solar cells (FPSCs)
Because of their potential in portable electronics, flexible perovskite solar cells (FPSCs) have sparked widespread research interest. Because of their low-temperature processability, SnO2 ETLs play a critical role in realizing highly efficient flexible PSCs.351–353
Zhou et al. reported graphene quantum dots (GQDs)/SnO2 composites (G@SnO2) as effective ETLs. GQDs can improve SnO2 electron mobility and coverage. Furthermore, G@SnO2 has a higher energy level than that of pristine SnO2, resulting in increased charge transfer and decreased charge recombination. As a result, the flexible PSCs based on G@SnO2 ETLs achieved a champion PCE of 17.7% with exceptional mechanical durability, retaining more than 91% of the initial PCE value after 500 bending cycles with a bending radius of 7 mm.354
Wang et al. reported plasma-enhanced atomic layer deposition (PEALD) for SnO2 ETLs processed at low temperatures. The PEALD-synthesized SnO2 is postannealed at 100 °C in the presence of water vapor. Finally, the best flexible PSC demonstrated the highest reported efficiency of 18.36%.155
Dong et al. used ultraviolet ozone (UVO) pretreatment to introduce controlled trace amounts of surface absorbed water on the FTO or ITO for a full-coverage SnO2 ETL with desirable morphology and crystallinity. It demonstrated optimal hydrolysis–condensation reactions for SnO2 regrowth. Compared to the control SnO2 ETL without UVO pretreatment, the rigid and flexible PSC devices with UVO pretreatment achieved high PCEs of up to 20.5% and 17.5%, respectively.355
However, the reported flexible PSCs are almost based on small surface areas to date. It is well known that low-temperature processable SnO2 provides fundamental support for mass production via some scalable fabrication and coating techniques, with enormous potential for practical applications in light, wearable, and portable electronic devices.
6.2 Large-area perovskite solar cells
Large-area fabrication is a critical component in achieving industrial production and commercialization. In general, when the area of the devices is increased to a large scale, the PCE decreases due to the inevitable loss of homogeneity in the films. As a result, various scalable deposition methods and related morphology control strategies for large-area uniform SnO2 films are developed, determining the performance of large-area flexible PSCs. A solution-based scalable deposition method appears to be promising for preparing large-area SnO2 films. In the lab, spin-coating is the most commonly used method for producing PSCs; however, it is hard to use it in the large-area fabrication.356,357 Other new methods for scalable production include slot-die,103 spray-coating,358 CBD,359,360 sputtering,148,149 and bar coating.361
Bu et al. slot-die-coated SnO2 on 5 × 6 cm2-sized substrates with a potassium interfacial passivation strategy and achieved a 14.89% efficiency (Fig. 20a and b). The results demonstrated that potassium passivation could stabilize SnO2 colloids, reducing hysteresis in SnO2-based devices.103 Taheri et al. pioneered the concept of combining laser scribing optimization with automated spray-coating of SnO2 layers for FPSCs (Fig. 20c and d). The method produced uniform and dense SnO2 ETLs with PCEs of 12% and 10.7% with active areas of 16.84 and 21.2 cm2, respectively.358 Qiu et al. looked into the effect of an oxidizing environment on the formation of SnO2 films. As an example, the champion device with an aperture area of 22.8 cm2 demonstrated a PCE of 12.03%. Currently, the largest SnO2-based PSC obtained by CBD deposition was 53.64 cm2 (active area) on a 100 cm2 substrate, with a certified PCE of 17.4%.360
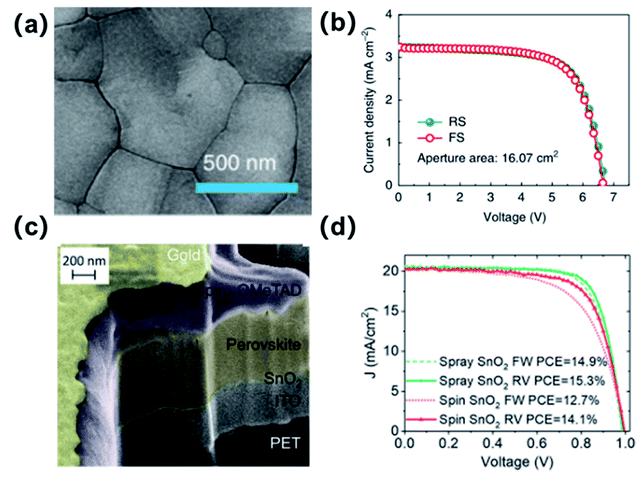 |
| Fig. 20 Top view SEM images of (a) a perovskite film based on SnO2 fabricated by slot-die coating; (c) the cross-sectional SEM images of a perovskite solar cell based on SnO2 fabricated by spray-coating; forward/reverse scan J–V curve of (b) the best device based on SnO2 fabricated by slot-die coating; (d) the best device based on SnO2 fabricated by spray-coating. (a and b) Reproduced with permission.103 Copyright 2020, Nature Publishing Group; (c and d) Reproduced with permission.358 Copyright 2021, ACS Publishing Group. | |
The manufacturing cost of SnO2 films is an important parameter for scalable SnO2 fabrication. Appropriate deposition methods and raw materials can significantly reduce costs. Instability, on the other hand, can seriously shorten the device's lifespan. As a result, the optimized deposition methods, passivation strategies, and encapsulation have a high potential for scaling up PSCs.
7. Conclusions and outlook
SnO2 has many merits of direct bandgap, high conductivity, low trap density, and being solution-processable under low-temperature conditions, which has been regarded as an ideal electron transport material in the field of PSCs. So far, the champion efficiency of SnO2 ETL-based planar PSCs combined with passivation strategy has been increased to 25.2%, which approached the device with the mesoporous structure.
SnO2-based PSCs have distinctive device structures, including planar, mesoporous, distressed, and flexible substrate-based structures. Different testimony techniques for SnO2 to suit various structures have been reported. Although the annealing temperature is much lower than that of TiO2 preparation, it is still excessively high to flexible PSCs or inversed structure PSCs. Subsequently, loads of work should be promoted to bring down the process temperature and simplify the process.
Like other metal oxidation, pristine SnO2 films contain many bulk and surface defects. As previously mentioned SnO2 combined with doped elements, bilayers, and interfacial engineering can retard the formation of the defect states, which improves the carrier transport performance, leading to negligible hysteresis in the device. To guide the design of an efficient process, we need to fully characterize the types and density of defects that form in SnO2 and the followed perovskite to gain a fundamental understanding. In addition, standard characterization protocols should be established to properly evaluate the effect of interfacial modifiers on the performance and stability of PSCs. In principle, a molecular library could be found by investigating the effect of functional groups, the conjugated system, and the substituent of the additives or interfacial modifiers on the passivation capacity. With the research information amassed and distributed in the entire exploration field, we can utilize AI to find the basic standards for choosing the materials and effective processes.
Conflicts of interest
The authors declare no conflict of interest.
Acknowledgements
This work was financially supported by the National Nature Science Foundation of China (21676188).
References
- Q. A. Akkerman and L. Manna, ACS Energy Lett., 2020, 5, 604–610 CrossRef CAS PubMed.
- C. Case, N. Beaumont and D. Kirk, ACS Energy Lett., 2019, 4, 2760–2762 CrossRef CAS.
- M. Jeong, I. W. Choi, E. M. Go, Y. Cho, M. Kim, B. Lee, S. Jeong, Y. Jo, H. W. Choi and J. Lee, Science, 2020, 369, 1615–1620 CrossRef CAS PubMed.
- X. Lin, D. Cui, X. Luo, C. Zhang, Q. Han, Y. Wang and L. Han, Energy Environ. Sci., 2020, 13, 3823–3847 RSC.
- F. Zhang, H. Lu, J. Tong, J. J. Berry, M. C. Beard and K. Zhu, Energy Environ. Sci., 2020, 13, 1154–1186 RSC.
- A. Kojima, K. Teshima, Y. Shirai and T. Miyasaka, J. Am. Chem. Soc., 2009, 131, 6050–6051 CrossRef CAS PubMed.
- A. R. b. M. Yusoff and M. K. Nazeeruddin, Adv. Energy Mater., 2018, 8, 1702073 CrossRef.
- M. Kim, G.-H. Kim, T. K. Lee, I. W. Choi, H. W. Choi, Y. Jo, Y. J. Yoon, J. W. Kim, J. Lee, D. Huh, H. Lee, S. K. Kwak, J. Y. Kim and D. S. Kim, Joule, 2019, 3, 2179–2192 CrossRef CAS.
- H. Min, M. Kim, S.-U. Lee, H. Kim, G. Kim, K. Choi, J. H. Lee and S. I. Seok, Science, 2019, 366, 749–753 CrossRef CAS PubMed.
- N. J. Jeon, H. Na, E. H. Jung, T.-Y. Yang, Y. G. Lee, G. Kim, H.-W. Shin, S. I. Seok, J. Lee and J. Seo, Nat. Energy, 2018, 3, 682–689 CrossRef CAS.
- W. S. Yang, B.-W. Park, E. H. Jung, N. J. Jeon, Y. C. Kim, D. U. Lee, S. S. Shin, J. Seo, E. K. Kim and J. H. Noh, Science, 2017, 356, 1376–1379 CrossRef CAS PubMed.
- H. Zhu, Y. Ren, L. Pan, O. Ouellette, F. T. Eickemeyer, Y. Wu, X. Li, S. Wang, H. Liu and X. Dong, J. Am. Chem. Soc., 2021, 143, 3231–3237 CrossRef CAS PubMed.
- M. Saliba, J. P. Correa-Baena, M. Grätzel, A. Hagfeldt and A. Abate, Angew. Chem., Int. Ed., 2018, 57, 2554–2569 CrossRef CAS PubMed.
- S. Yun, Y. Qin, A. R. Uhl, N. Vlachopoulos, M. Yin, D. Li, X. Han and A. Hagfeldt, Energy Environ. Sci., 2018, 11, 476–526 RSC.
- V. M. Goldschmidt, Naturwissenschaften, 1926, 14, 477–485 CrossRef CAS.
- B. Saparov and D. B. Mitzi, Chem. Rev., 2016, 116, 4558–4596 CrossRef CAS PubMed.
- J. You, L. Meng, T.-B. Song, T.-F. Guo, Y. Yang, W.-H. Chang, Z. Hong, H. Chen, H. Zhou, Q. Chen, Y. Liu, N. De Marco and Y. Yang, Nat. Nanotechnol., 2016, 11, 75–81 CrossRef CAS PubMed.
- F. Zhang and K. Zhu, Adv. Energy Mater., 2020, 10, 1902579 CrossRef CAS.
- J. Jeong, M. Kim, J. Seo, H. Lu, P. Ahlawat, A. Mishra, Y. Yang, M. A. Hope, F. T. Eickemeyer and M. Kim, Nature, 2021, 592, 381–385 CrossRef CAS PubMed.
- W. Hu, W. Zhou, X. Lei, P. Zhou, M. Zhang, T. Chen, H. Zeng, J. Zhu, S. Dai and S. Yang, Adv. Mater., 2019, 31, 1806095 CrossRef PubMed.
- D. Yang, R. Yang, J. Zhang, Z. Yang, S. Liu and C. Li, Energy Environ. Sci., 2015, 8, 3208–3214 RSC.
- F. Zhang, D. Bi, N. Pellet, C. Xiao, Z. Li, J. J. Berry, S. M. Zakeeruddin, K. Zhu and M. Grätzel, Energy Environ. Sci., 2018, 11, 3480–3490 RSC.
- F. Zhang, W. Shi, J. Luo, N. Pellet, C. Yi, X. Li, X. Zhao, T. J. S. Dennis, X. Li, S. Wang, Y. Xiao, S. M. Zakeeruddin, D. Bi and M. Grätzel, Adv. Mater., 2017, 29, 1606806 CrossRef PubMed.
- F. Zhang, C. Xiao, X. Chen, B. W. Larson, S. P. Harvey, J. J. Berry and K. Zhu, Joule, 2019, 3, 1452–1463 CrossRef CAS.
- C. Xiao, F. Zhang, X. Chen, M. Yang, S. P. Harvey, M. C. Beard, J. J. Berry, C.-S. Jiang, M. M. Al-Jassim and K. Zhu, ACS Energy Lett., 2021, 6, 650–658 CrossRef CAS.
- S.-H. Lee, S. Jeong, S. Seo, H. Shin, C. Ma and N.-G. Park, ACS Energy Lett., 2021, 6, 1612–1621 CrossRef CAS.
- H. Lu, Y. Liu, P. Ahlawat, A. Mishra, W. R. Tress, F. T. Eickemeyer, Y. Yang, F. Fu, Z. Wang, C. E. Avalos, B. I. Carlsen, A. Agarwalla, X. Zhang, X. Li, Y. Zhan, S. M. Zakeeruddin, L. Emsley, U. Rothlisberger, L. Zheng, A. Hagfeldt and M. Grätzel, Science, 2020, 370, eabb8985 CrossRef CAS PubMed.
- J.-H. Im, C.-R. Lee, J.-W. Lee, S.-W. Park and N.-G. Park, Nanoscale, 2011, 3, 4088–4093 RSC.
- M. M. Lee, J. Teuscher, T. Miyasaka, T. N. Murakami and H. J. Snaith, Science, 2012, 338, 643–647 CrossRef CAS PubMed.
- M. Liu, M. B. Johnston and H. J. Snaith, Nature, 2013, 501, 395–398 CrossRef CAS PubMed.
- H. Zhou, Q. Chen, G. Li, S. Luo, T.-b. Song, H.-S. Duan, Z. Hong, J. You, Y. Liu and Y. Yang, Science, 2014, 345, 542–546 CrossRef CAS PubMed.
- W. S. Yang, J. H. Noh, N. J. Jeon, Y. C. Kim, S. Ryu, J. Seo and S. I. Seok, Science, 2015, 348, 1234–1237 CrossRef CAS PubMed.
- M. Saliba, T. Matsui, J.-Y. Seo, K. Domanski, J.-P. Correa-Baena, M. K. Nazeeruddin, S. M. Zakeeruddin, W. Tress, A. Abate, A. Hagfeldt and M. Grätzel, Energy Environ. Sci., 2016, 9, 1989–1997 RSC.
- E. H. Jung, N. J. Jeon, E. Y. Park, C. S. Moon, T. J. Shin, T. Y. Yang, J. H. Noh and J. Seo, Nature, 2019, 567, 511–515 CrossRef CAS PubMed.
- Q. Jiang, Y. Zhao, X. Zhang, X. Yang, Y. Chen, Z. Chu, Q. Ye, X. Li, Z. Yin and J. You, Nat. Photonics, 2019, 13, 460–466 CrossRef CAS.
- J. J. Yoo, G. Seo, M. R. Chua, T. G. Park, Y. Lu, F. Rotermund, Y. K. Kim, C. S. Moon, N. J. Jeon, J. P. Correa-Baena, V. Bulovic, S. S. Shin, M. G. Bawendi and J. Seo, Nature, 2021, 590, 587–593 CrossRef CAS PubMed.
- A. Agresti, S. Pescetelli, L. Cinà, D. Konios, G. Kakavelakis, E. Kymakis and A. D. Carlo, Adv. Funct. Mater., 2016, 26, 2686–2694 CrossRef CAS.
- M. M. Byranvand, T. Kim, S. Song, G. Kang, S. U. Ryu and T. Park, Adv. Energy Mater., 2018, 8, 1870020 CrossRef.
- T. Duong, Y. Wu, H. Shen, J. Peng, X. Fu, D. Jacobs, E. C. Wang, T. C. Kho, K. C. Fong and M. Stocks, Adv. Energy Mater., 2017, 7, 1700228 CrossRef.
- S. Sidhik, A. Cerdan Pasaran, D. Esparza, T. Lopez Luke, R. Carriles and E. De la Rosa, ACS Appl. Mater. Interfaces, 2018, 10, 3571–3580 CrossRef CAS PubMed.
- T. Leijtens, G. E. Eperon, S. Pathak, A. Abate, M. M. Lee and H. J. Snaith, Nat. Commun., 2013, 4, 2885 CrossRef PubMed.
- J. Liu, Y. Wu, C. Qin, X. Yang, T. Yasuda, A. Islam, K. Zhang, W. Peng, W. Chen and L. Han, Energy Environ. Sci., 2014, 7, 2963–2967 RSC.
- B. Wang, Q. Wang, Y. M. Wei and Z. P. Li, Renewable Sustainable Energy Rev., 2018, 90, 187–194 CrossRef.
- Q. Jiang, L. Zhang, H. Wang, X. Yang, J. Meng, H. Liu, Z. Yin, J. Wu, X. Zhang and J. You, Nat. Energy, 2016, 2, 1–7 Search PubMed.
- C. C. Chueh, C. Z. Li and K. Y. Jen, Energy Environ. Sci., 2015, 8, 1160–1189 RSC.
- P. Chen, Y. Bai, S. Wang, M. Lyu, J.-H. Yun and L. Wang, Adv. Funct. Mater., 2018, 28, 1706923 CrossRef.
- S. S. Shin, S. J. Lee and S. I. Seok, Adv. Funct. Mater., 2019, 29, 1900455 CrossRef CAS.
- L. Lin, T. W. Jones, T. C. J. Yang, N. W. Duffy, J. Li, L. Zhao, B. Chi, X. Wang and G. J. Wilson, Adv. Funct. Mater., 2021, 31, 2008300 CrossRef CAS.
- J. Han, H. Kwon, E. Kim, D.-W. Kim, H. J. Son and D. H. Kim, J. Mater. Chem. A, 2020, 8, 2105–2113 RSC.
- H. Zhu, Y. Liu, F. T. Eickemeyer, L. Pan, D. Ren, M. A. Ruiz-Preciado, B. Carlsen, B. Yang, X. Dong, Z. Wang, H. Liu, S. Wang, S. M. Zakeeruddin, A. Hagfeldt, M. I. Dar, X. Li and M. Grätzel, Adv. Mater., 2020, 32, 1907757 CrossRef CAS PubMed.
- M. A. Mahmud, N. K. Elumalai, M. B. Upama, D. Wang, V. R. Gonçales, M. Wright, C. Xu, F. Haque and A. Uddin, J. Power Sources, 2018, 383, 59–71 CrossRef CAS.
- M. M. Tavakoli, R. Tavakoli, P. Yadav and J. Kong, J. Mater. Chem. A, 2019, 7, 679–686 RSC.
- P. Chen, X. Yin, M. Que, X. Liu and W. Que, J. Mater. Chem. A, 2017, 5, 9641–9648 RSC.
- X. Guo, Z. Lin, J. Ma, Z. Hu, J. Su, C. Zhang, J. Zhang, J. Chang and Y. Hao, J. Power Sources, 2019, 438, 226981 CrossRef CAS.
- X. Ye, H. Ling, R. Zhang, Z. Wen, S. Hu, T. Akasaka, J. Xia and X. Lu, J. Power Sources, 2020, 448, 227419 CrossRef CAS.
- F. Zhao, Y. Guo, X. Wang, J. Tao, J. Jiang, Z. Hu and J. Chu, Sol. Energy, 2019, 191, 263–271 CrossRef CAS.
- C. Chen, Y. Jiang, Y. Wu, J. Guo, X. Kong, X. Wu, Y. Li, D. Zheng, S. Wu and X. Gao, Sol. RRL, 2020, 4, 1900499 CrossRef CAS.
- K. Wang, Y. Shi, Q. Dong, Y. Li, S. Wang, X. Yu, M. Wu and T. Ma, J. Phys. Chem. Lett., 2015, 6, 755–759 CrossRef CAS PubMed.
- Y. Guo, T. Liu, N. Wang, Q. Luo, H. Lin, J. Li, Q. Jiang, L. Wu and Z. Guo, Nano Energy, 2017, 38, 193–200 CrossRef CAS.
- W. Hu, T. Liu, X. Yin, H. Liu, X. Zhao, S. Luo, Y. Guo, Z. Yao, J. Wang and N. Wang, J. Mater. Chem. A, 2016, 5, 1434–1441 RSC.
- Q. Luo, H. Chen, Y. Lin, H. Du, Q. Hou, F. Hao, N. Wang, Z. Guo and J. Huang, Adv. Funct. Mater., 2017, 27, 1702090 CrossRef.
- W. Zhu, Q. Zhang, C. Zhang, D. Chen, L. Zhou, Z. Lin, J. Chang, J. Zhang and Y. Hao, Dalton Trans., 2018, 47, 6404–6411 RSC.
- X. Wang, L.-L. Deng, L.-Y. Wang, S.-M. Dai, Z. Xing, X.-X. Zhan, X.-Z. Lu, S.-Y. Xie, R.-B. Huang and L.-S. Zheng, J. Mater. Chem. A, 2017, 5, 1706–1712 RSC.
- J. Yang, Q. Zhang, J. Xu, H. Liu, R. Qin, H. Zhai, S. Chen and M. Yuan, Nanomaterials, 2019, 9, 1666 CrossRef CAS PubMed.
- J. Dou, Y. Zhang, Q. Wang, A. Abate, Y. Li and M. Wei, Chem. Commun., 2019, 55, 14673–14676 RSC.
- F. Sadegh, S. Akin, M. Moghadam, V. Mirkhani, M. A. Ruiz-Preciado, Z. Wang, M. M. Tavakoli, M. Graetzel, A. Hagfeldt and W. Tress, Nano Energy, 2020, 75, 105038 CrossRef CAS.
- C. W. Myung, G. Lee and K. S. Kim, J. Mater. Chem. A, 2018, 6, 23071–23077 RSC.
- J. Chung, S. S. Shin, G. Kim, N. J. Jeon, T.-Y. Yang, J. H. Noh and J. Seo, Joule, 2019, 3, 1977–1985 CrossRef CAS.
- S. S. Shin, J. H. Suk, B. J. Kang, W. Yin, S. J. Lee, J. H. Noh, T. K. Ahn, F. Rotermund, I. S. Cho and S. I. Seok, Energy Environ. Sci., 2019, 12, 958–964 RSC.
- A. Bera, K. Wu, A. Sheikh, E. Alarousu, O. F. Mohammed and T. Wu, J. Phys. Chem. C, 2014, 118, 28494–28501 CrossRef CAS.
- N. Tsvetkov, B. C. Moon, J. Lee and J. K. Kang, ACS Appl. Energy Mater., 2019, 3, 344–351 CrossRef.
- K. Mahmood, A. Khalid, S. W. Ahmad, H. G. Qutab, M. Hameed and R. Sharif, Sol. Energy, 2020, 203, 32–36 CrossRef CAS.
- A. J. Yun, J. Kim, T. Hwang and B. Park, ACS Appl. Energy Mater., 2019, 2, 3554–3560 CrossRef CAS.
- C. D. Wessendorf, J. Hanisch, D. Müller and E. Ahlswede, Sol. RRL, 2018, 2, 1800056 CrossRef.
- W. A. Dunlap-Shohl, R. Younts, B. Gautam, K. Gundogdu and D. B. Mitzi, J. Phys. Chem. C, 2016, 120, 16437–16445 CrossRef CAS.
- G. Tong, Z. Song, C. Li, Y. Zhao, L. Yu, J. Xu, Y. Jiang, Y. Sheng, Y. Shi and K. Chen, RSC Adv., 2017, 7, 19457–19463 RSC.
- E. Zhao, L. Gao, S. Yang, L. Wang, J. Cao and T. Ma, Nano Res., 2018, 11, 5913–5923 CrossRef CAS.
- D.-B. Li, L. Hu, Y. Xie, G. Niu, T. Liu, Y. Zhou, L. Gao, B. Yang and J. Tang, ACS Photonics, 2016, 3, 2122–2128 CrossRef CAS.
- H. Wei, J. Wu, P. Qiu, S. Liu, Y. He, M. Peng, D. Li, Q. Meng, F. Zaera and X. Zheng, J. Mater. Chem. A, 2019, 7, 25347–25354 RSC.
- F. Tan, W. Xu, X. Hu, P. Yu and W. Zhang, Nanoscale Res. Lett., 2017, 12, 614 CrossRef PubMed.
- L. Wang, W. Fu, Z. Gu, C. Fan, X. Yang, H. Li and H. Chen, J. Mater. Chem. C, 2014, 2, 9087–9090 RSC.
- Z. Rao, B. Du, C. Huang, L. Shu, P. Lin, N. Fu and S. Ke, J. Alloys Compd., 2019, 789, 276–281 CrossRef CAS.
- W. Ke, G. Fang, Q. Liu, L. Xiong, P. Qin, H. Tao, J. Wang, H. Lei, B. Li and J. Wan, J. Am. Chem. Soc., 2015, 137, 6730–6733 CrossRef CAS PubMed.
- Y. Li, J. Zhu, Y. Huang, F. Liu, M. Lv, S. Chen, L. Hu, J. Tang, J. Yao and S. Dai, RSC Adv., 2015, 5, 28424–28429 RSC.
- Q. Jiang, X. Zhang and J. You, Small, 2018, 14, 1801154 CrossRef PubMed.
- W. Ke, D. Zhao, A. J. Cimaroli, C. R. Grice, P. Qin, Q. Liu, L. Xiong, Y. Yan and G. Fang, J. Mater. Chem. A, 2015, 3, 24163–24168 RSC.
- L. Kavan, L. Steier and M. Grätzel, J. Phys. Chem. C, 2017, 121, 342–350 CrossRef CAS.
- E. J. Yeom, S. S. Shin, W. S. Yang, S. J. Lee, W. Yin, D. Kim, J. H. Noh, T. K. Ahn and S. I. Seok, J. Mater. Chem. A, 2017, 5, 79–86 RSC.
- A. F. Khan, M. Mehmood, M. Aslam and M. Ashraf, Appl. Surf. Sci., 2010, 256, 2252–2258 CrossRef CAS.
- S. Lin, B. Yang, X. Qiu, J. Yan, J. Shi, Y. Yuan, W. Tan, X. Liu, H. Huang and Y. Gao, Org. Electron., 2018, 53, 235–241 CrossRef CAS.
- X. Qin, Z. Zhao, Y. Wang, J. Wu, Q. Jiang and J. You, J. Semicond., 2017, 38, 011002 CrossRef.
- R. M. Pasquarelli, D. S. Ginley and R. O'Hayre, Chem. Soc. Rev., 2011, 40, 5406–5441 RSC.
- G. Yang, C. Chen, F. Yao, Z. Chen, Q. Zhang, X. Zheng, J. Ma, H. Lei, P. Qin and L. Xiong, Adv. Mater., 2018, 30, 1706023 CrossRef PubMed.
- L. Zuo, H. Guo, D. W. deQuilettes, S. Jariwala, N. De Marco, S. Dong, R. DeBlock, D. S. Ginger, B. Dunn, M. Wang and Y. Yang, Sci. Adv., 2017, 3, e1700106 CrossRef PubMed.
- J. Jia, J. Dong, B. Shi, J. Wu, Y. Wu and B. Cao, ACS Appl. Mater. Interfaces, 2021, 13, 2472–2482 CrossRef CAS PubMed.
- A. E. Shalan, E. Akman, F. Sadegh and S. Akin, J. Phys. Chem. Lett., 2021, 12, 997–1004 CrossRef CAS PubMed.
- H. Bi, X. Zuo, B. Liu, D. He, L. Bai, W. Wang, X. Li, Z. Xiao, K. Sun, Q. Song, Z. Zang and J. Chen, J. Mater. Chem. A, 2021, 9, 3940–3951 RSC.
- P. Hang, J. Xie, C. Kan, B. Li, Y. Zhang, P. Gao, D. Yang and X. Yu, Adv. Mater., 2021, 33, 2006910 CrossRef CAS PubMed.
- X. Fan, Y. Rui, X. Han, J. Yang, Y. Wang and Q. Zhang, J. Power Sources, 2020, 448, 227405 CrossRef CAS.
- J. A. Smith, O. S. Game, J. E. Bishop, E. L. K. Spooner, R. C. Kilbride, C. Greenland, R. Jayaprakash, T. I. Alanazi, E. J. Cassella, A. Tejada, G. Chistiakova, M. Wong-Stringer, T. J. Routledge, A. J. Parnell, D. B. Hammond and D. G. Lidzey, ACS Appl. Energy Mater., 2020, 3, 5552–5562 CrossRef CAS PubMed.
- B. Taheri, E. Calabrò, F. Matteocci, D. Di Girolamo, G. Cardone, A. Liscio, A. Di Carlo and F. Brunetti, Energy Technol., 2020, 8, 1901284 CrossRef CAS.
- G. Mathiazhagan, A. Seeber, T. Gengenbach, S. Mastroianni, D. Vak, A. S. R. Chesman, M. Gao, D. Angmo and A. Hinsch, Sol. RRL, 2020, 4, 2000262 CrossRef CAS.
- T. Bu, J. Li, F. Zheng, W. Chen, X. Wen, Z. Ku, Y. Peng, J. Zhong, Y.-B. Cheng and F. Huang, Nat. Commun., 2018, 9, 4609 CrossRef PubMed.
- C. Gong, S. Tong, K. Huang, H. Li, H. Huang, J. Zhang and J. Yang, Sol. RRL, 2020, 4, 1900204 CrossRef CAS.
- X. Chang, J. Fang, Y. Fan, T. Luo, H. Su, Y. Zhang, J. Lu, L. Tsetseris, T. D. Anthopoulos and S. Liu, Adv. Mater., 2020, 32, 2001243 CrossRef CAS PubMed.
- Y. Peng, F. Zeng, Y. Cheng, C. Wang, K. Huang, P. Xie, H. Xie, Y. Gao and J. Yang, Org. Electron., 2020, 83, 105736 CrossRef CAS.
- V. Rohnacher, F. Ullrich, H. Eggers, F. Schackmar, S. Hell, A. Salazar, C. Huck, G. Hernandez-Sosa, U. W. Paetzold, W. Jaegermann and A. Pucci, Adv. Mater. Technol., 2021, 6, 2000282 CrossRef CAS.
- Y. Lin, J. Chen, M. M. Tavakoli, Y. Gao, Y. Zhu, D. Zhang, M. Kam, Z. He and Z. Fan, Adv. Mater., 2019, 31, 1804285 CrossRef PubMed.
- Z. Guo, A. K. Jena, I. Takei, G. M. Kim, M. A. Kamarudin, Y. Sanehira, A. Ishii, Y. Numata, S. Hayase and T. Miyasaka, J. Am. Chem. Soc., 2020, 142, 9725–9734 CAS.
- Z. Guo, S. Teo, Z. Xu, C. Zhang, Y. Kamata, S. Hayase and T. Ma, J. Mater. Chem. A, 2019, 7, 1227–1232 RSC.
- J. Duan, Q. W. Yue, Q. Xiong, L. Wang, L. Zhu, K. Zhang, J. Zhang and H. Wang, Appl. Surf. Sci., 2019, 470, 613–621 CrossRef CAS.
- D. Zhang, H. Tian, S. Bu, T. Yan and Z. Ge, J. Alloys Compd., 2020, 831, 154717 CrossRef CAS.
- K. Zhang, J. Duan, F. Liu, J. Zhang and H. Wang, J. Mater. Sci., 2021, 56, 677–690 CrossRef CAS.
- Q. Dong, Y. Shi, C. Zhang, Y. Wu and L. Wang, Nano Energy, 2017, 40, 336–344 CrossRef CAS.
- X. Qiu, B. Yang, H. Chen, G. Liu, Y. Liu, Y. Yuan, H. Huang, H. Xie, D. Niu and Y. Gao, Org. Electron., 2018, 58, 126–132 CrossRef CAS.
- H. Tang, Q. Cao, Z. He, S. Wang, J. Han, T. Li, B. Gao, J. Yang, D. Deng and X. Li, Sol. RRL, 2020, 4, 1900415 CrossRef CAS.
- X. Xu, Z. Xu, J. Tang, X. Zhang, L. Zhang, J. Wu and Z. Lan, Chem. Eng. J., 2018, 351, 391–398 CrossRef CAS.
- U. Akpan and B. Hameed, Appl. Catal., A, 2010, 375, 1–11 CrossRef CAS.
- A. E. Danks, S. R. Hall and Z. Schnepp, Mater. Horiz., 2016, 3, 91–112 RSC.
- A. Kołodziejczak-Radzimska and T. Jesionowski, Materials, 2014, 7, 2833–2881 CrossRef PubMed.
- W. K. Tan, H. Muto, G. Kawamura, Z. Lockman and A. Matsuda, Nanomaterials, 2021, 11, 181 CrossRef CAS PubMed.
- H. B. Lee, N. Kumar, M. M. Ovhal, Y. J. Kim, Y. M. Song and J. W. Kang, Adv. Funct. Mater., 2020, 30, 2001559 CrossRef CAS.
- H. Xu, Z. Hu, Y. Wang, C. Yang, C. Gao, H. Zhang, J. Zhang and Y. Zhu, Nanotechnology, 2020, 31, 315205 CrossRef CAS PubMed.
- P.-H. Lee, T.-T. Wu, K.-Y. Tian, C.-F. Li, C.-H. Hou, J.-J. Shyue, C.-F. Lu, Y.-C. Huang and W.-F. Su, ACS Appl. Mater. Interfaces, 2020, 12, 45936–45949 CrossRef CAS PubMed.
- S. Jeong, S. Seo, H. Park and H. Shin, Chem. Commun., 2019, 55, 2433–2436 RSC.
- M. Abulikemu, M. Neophytou, J. M. Barbé, M. L. Tietze, A. El Labban, D. H. Anjum, A. Amassian, I. McCulloch and S. Del Gobbo, J. Mater. Chem. A, 2017, 5, 7759–7763 RSC.
- Z. Zhu, Y. Bai, X. Liu, C. C. Chueh, S. Yang and A. K. Y. Jen, Adv. Mater., 2016, 28, 6478–6484 CrossRef CAS PubMed.
- M. Zhu, W. Liu, W. Ke, S. Clark, E. B. Secor, T.-B. Song, M. G. Kanatzidis, X. Li and M. C. Hersam, J. Mater. Chem. A, 2017, 5, 24110–24115 RSC.
- L. Huang, X. X. Sun, C. Li, J. Xu, R. Xu, Y. Y. Du, J. Ni, H. K. Cai, J. Li, Z. Y. Hu and J. J. Jianjun, ACS Appl. Mater. Interfaces, 2017, 9, 21909–21920 CrossRef CAS PubMed.
- X. Zhang, F. Zabihi, H. Xiong, M. Eslamian and Q. Zhang, Chem. Eng. J., 2020, 394, 124887 CrossRef CAS.
- Q. Jiang, Z. Chu, P. Wang, X. Yang, H. Liu, Y. Wang, Z. Yin, J. Wu, X. Zhang and J. You, Adv. Mater., 2017, 29, 1703852 CrossRef PubMed.
- P. Zhu, S. Gu, X. Luo, Y. Gao, S. Li, J. Zhu and H. Tan, Adv. Energy Mater., 2020, 10, 1903083 CrossRef CAS.
- Y. Zhao, L. Zhang, J. Liu, K. Adair, F. Zhao, Y. Sun, T. Wu, X. Bi, K. Amine, J. Lu and X. Sun, Chem. Soc. Rev., 2021, 50, 3889–3956 RSC.
- Z. Xing, J. Xiao, T. Hu, X. Meng, D. Li, X. Hu and Y. Chen, Small Methods, 2020, 4, 2000588 CrossRef CAS.
- Y. Lee, S. Lee, G. Seo, S. Paek, K. T. Cho, A. J. Huckaba, M. Calizzi, D. w. Choi, J. S. Park and D. Lee, Adv. Sci., 2018, 5, 1800130 CrossRef PubMed.
- J. Bing, S. Huang and A. W. Ho-Baillie, Energy Technol., 2020, 8, 1901114 CrossRef CAS.
- Y. Gu, C. Ye, X. Yin, J. Han, H. Shen, J. Li, X. Hao and H. Lin, Chem. Eng. J., 2018, 351, 791–798 CrossRef CAS.
- Y. Zhou, X. Li and H. Lin, Small, 2020, 16, 1902579 CrossRef CAS PubMed.
- M. J. Jeong, K. M. Yeom, S. J. Kim, E. H. Jung and J. H. Noh, Energy Environ. Sci., 2021, 14, 2419–2428 RSC.
- Y. Ko, Y. Kim, C. Lee, T. Kim, S. Kim, Y. J. Yun, H. j. Gwon, N. H. Lee and Y. Jun, ChemSusChem, 2020, 13, 4051–4063 CrossRef CAS PubMed.
- L. Xiong, Y. Guo, J. Wen, H. Liu, G. Yang, P. Qin and G. Fang, Adv. Funct. Mater., 2018, 28, 1802757 CrossRef.
- J. J. Ma, X. L. Zheng, H. W. Lei, W. J. Ke, C. Chen, Z. L. Chen, G. Yang and G. J. Fang, Sol. RRL, 2017, 1, 1700118 CrossRef.
- Z. Song, W. Bi, X. Zhuang, Y. Wu, B. Zhang, X. Chen, C. Chen, Q. Dai and H. Song, Sol. RRL, 2020, 4, 1900266 CrossRef CAS.
- J. Li, L. Li, W. Chen, Q. Yi and G. Zou, Nanotechnology, 2020, 32, 025606 CrossRef PubMed.
- E. Aydin, J. Troughton, M. De Bastiani, E. Ugur, M. Sajjad, A. Alzahrani, M. Neophytou, U. Schwingenschlögl, F. Laquai and D. Baran, ACS Appl. Energy Mater., 2018, 1, 6227–6233 CrossRef.
- M. K. Otoufi, M. Ranjbar, A. Kermanpur, N. Taghavinia, M. Minbashi, M. Forouzandeh and F. Ebadi, Sol. Energy, 2020, 208, 697–707 CrossRef CAS.
- Y. Mo, J. Shi, P. Zhou, S. Li, T. Bu, Y.-B. Cheng and F. Huang, Sol. RRL, 2019, 3, 1900209 CrossRef.
- L. Qiu, Z. Liu, L. K. Ono, Y. Jiang, D. Y. Son, Z. Hawash, S. He and Y. Qi, Adv. Funct. Mater., 2019, 29, 1806779 CrossRef CAS.
- G. Bai, Z. Wu, J. Li, T. Bu, W. Li, W. Li, F. Huang, Q. Zhang, Y.-B. Cheng and J. Zhong, Sol. Energy, 2019, 183, 306–314 CrossRef CAS.
- J.-Y. Chen, C.-C. Chueh, Z. Zhu, W.-C. Chen and A. K.-Y. Jen, Sol. Energy Mater. Sol. Cells, 2017, 164, 47–55 CrossRef CAS.
- Y. S. Seo, E.-Y. Ahn, J. Park, T. Y. Kim, J. E. Hong, K. Kim, Y. Park and Y. Park, Nanoscale Res. Lett., 2017, 12, 7 CrossRef PubMed.
- M.-G. Kim, M. G. Kanatzidis, A. Facchetti and T. J. Marks, Nat. Mater., 2011, 10, 382–388 CrossRef CAS PubMed.
- X. Liu, K. W. Tsai, Z. Zhu, Y. Sun, C. C. Chueh and A. K. Y. Jen, Adv. Mater. Interfaces, 2016, 3, 1600122 CrossRef.
- L. Mai, D. Zanders, E. Subasi, E. Ciftyurek, C. Hoppe, D. Rogalla, W. Gilbert, T. L. Arcos, K. Schierbaum, G. Grundmeier, C. Bock and A. Devi, ACS Appl. Mater. Interfaces, 2019, 11, 3169–3180 CrossRef CAS PubMed.
- C. Wang, L. Guan, D. Zhao, Y. Yu, C. R. Grice, Z. Song, R. A. Awni, J. Chen, J. Wang and X. Zhao, ACS Energy Lett., 2017, 2, 2118–2124 CrossRef CAS.
- C. Wang, C. Xiao, Y. Yu, D. Zhao, R. A. Awni, C. R. Grice, K. Ghimire, I. Constantinou, W. Liao and A. J. Cimaroli, Adv. Energy Mater., 2017, 7, 1700414 CrossRef.
- C. Wang, D. Zhao, C. R. Grice, W. Liao, Y. Yu, A. Cimaroli, N. Shrestha, P. J. Roland, J. Chen and Z. Yu, J. Mater. Chem. A, 2016, 4, 12080–12087 RSC.
- M. Singh, A. Ng, Z. Ren, H. Hu, H.-C. Lin, C.-W. Chu and G. Li, Nano Energy, 2019, 60, 275–284 CrossRef CAS.
- M. F. M. Noh, N. A. Arzaee, J. Safaei, N. A. Mohamed, H. P. Kim, J. Jang and M. A. M. Teridi, J. Alloys Compd., 2019, 773, 997–1008 CrossRef.
- H. Sun, Y. Zhou, Y. Xin, K. Deng, L. Meng, J. Xiong and L. Li, Adv. Funct. Mater., 2019, 29, 1808667 CrossRef.
- Y. Guo, X. Yin, J. Liu, W. Chen, S. Wen, M. Que, H. Xie, Y. Yang, W. Que and B. Gao, Org. Electron., 2019, 65, 207–214 CrossRef CAS.
- X. Zhang, Y. Rui, Y. Wang, J. Xu, H. Wang, Q. Zhang and P. Müller-Buschbaum, J. Power Sources, 2018, 402, 460–467 CrossRef CAS.
- K. Mahmood, A. Khalid, F. Nawaz and M. T. Mehran, J. Colloid Interface Sci., 2018, 532, 387–394 CrossRef CAS PubMed.
- Z. Chen, G. Yang, X. Zheng, H. Lei, C. Chen, J. Ma, H. Wang and G. Fang, J. Power Sources, 2017, 351, 123–129 CrossRef CAS.
- J. Jia, J. Dong, J. Wu, H. Wei and B. Cao, J. Alloys Compd., 2020, 844, 156032 CrossRef CAS.
- Y. Zhao, J. Zhu, B. He and Q. Tang, ACS Appl. Mater. Interfaces, 2021, 13, 11058–11066 CrossRef CAS PubMed.
- G. S. Han, H. S. Chung, D. H. Kim, B. J. Kim, J.-W. Lee, N.-G. Park, I. S. Cho, J.-K. Lee, S. Lee and H. S. Jung, Nanoscale, 2015, 7, 15284–15290 RSC.
- S. S. Mali, J. V. Patil, H. Kim and C. K. Hong, Nanoscale, 2018, 10, 8275–8284 RSC.
- Y. Lv, P. Wang, B. Cai, Q. Ma, X. Zheng, Y. Wu, Q. Jiang, J. Liu and W. H. Zhang, Sol. RRL, 2018, 2, 1800133 CrossRef.
- Y. E. Kim, U. C. Baek, J. H. Kim, W. S. Chi and J. T. Park, Mater. Chem. Phys., 2020, 254, 123538 CrossRef CAS.
- P. Zhou, J. Wu, Y. Tu, M. Zhen, J. Huo, Y. Wei and Z. Lan, Sol. Energy, 2016, 137, 579–584 CrossRef CAS.
- K. Deng, H. Lu, Z. Shi, Q. Liu and L. Li, ACS Appl. Mater. Interfaces, 2013, 5, 7845–7851 CrossRef CAS PubMed.
- S. Gubbala, V. Chakrapani, V. Kumar and M. K. Sunkara, Adv. Funct. Mater., 2008, 18, 2411–2418 CrossRef CAS.
- Z. Li, Y. Zhou, W. Mao and Z. Zou, J. Power Sources, 2015, 274, 575–581 CrossRef CAS.
- I. J. Park, S. Park, D. H. Kim, H. Jeong and S. Lee, Mater. Lett., 2017, 202, 48–51 CrossRef CAS.
- X. Hou, Y. Hu, H. Jiang, J. Huo, Y. Li and C. Li, J. Mater. Chem. A, 2013, 1, 13814–13820 RSC.
- A. Ashok, G. Gopakumar, S. Vijayaraghavan, S. V. Nair and M. Shanmugam, IEEE J. Photovolt., 2018, 8, 1044–1050 Search PubMed.
- Q. Wali, Z. H. Bakr, N. A. Manshor, A. Fakharuddin and R. Jose, Sol. Energy, 2016, 132, 395–404 CrossRef CAS.
- Y.-F. Wang, K.-N. Li, W.-Q. Wu, Y.-F. Xu, H.-Y. Chen, C.-Y. Su and D.-B. Kuang, RSC Adv., 2013, 3, 13804–13810 RSC.
- P. Chen, X. Yin, M. Que, Y. Yang and W. Que, RSC Adv., 2016, 6, 57996–58002 RSC.
- A. Fakharuddin, F. Di Giacomo, A. L. Palma, F. Matteocci, I. Ahmed, S. Razza, A. D'Epifanio, S. Licoccia, J. Ismail, A. Di Carlo, T. M. Brown and R. Jose, ACS Nano, 2015, 9, 8420–8429 CrossRef CAS PubMed.
- K. Mahmood, B. S. Swain and A. Amassian, Adv. Energy Mater., 2015, 5, 1500568 CrossRef.
- P. Ruankham, D. Wongratanaphisan, A. Gardchareon, S. Phadungdhitidhada, S. Choopun and T. Sagawa, Appl. Surf. Sci., 2017, 410, 393–400 CrossRef CAS.
- C. Liu, R. Zhu, A. Ng, Z. Ren, S. H. Cheung, L. Du, S. K. So, J. A. Zapien, A. B. Djurišić and D. L. Phillips, J. Mater. Chem. A, 2017, 5, 15970–15980 RSC.
- U. V. Desai, C. Xu, J. Wu and D. Gao, J. Phys. Chem. C, 2013, 117, 3232–3239 CrossRef CAS.
- D. J. Kim, S. H. Ahn, C. S. Lee and J. H. Kim, J. Mater. Chem. A, 2015, 3, 17644–17651 RSC.
- H. J. Snaith and C. Ducati, Nano Lett., 2010, 10, 1259–1265 CrossRef CAS PubMed.
- P. Tiwana, P. Docampo, M. B. Johnston, H. J. Snaith and L. M. Herz, ACS Nano, 2011, 5, 5158–5166 CrossRef CAS PubMed.
- C. Gao, S. Yuan, B. Cao and J. Yu, Chem. Eng. J., 2017, 325, 378–385 CrossRef CAS.
- Q. Liu, M. C. Qin, W. J. Ke, X. L. Zheng, Z. Chen, P. L. Qin, L. B. Xiong, H. W. Lei, J. W. Wan and J. Wen, Adv. Funct. Mater., 2016, 26, 6069–6075 CrossRef CAS.
- W. Q. Wu, D. Chen, Y. B. Cheng and R. A. Caruso, Sol. RRL, 2017, 1, 1700117 CrossRef.
- G. Yang, H. W. Lei, H. Tao, X. L. Zheng, J. J. Ma, Q. Liu, W. J. Ke, Z. L. Chen, L. B. Xiong, P. L. Qin, Z. Chen, M. C. Qin, X. H. Lu, Y. F. Yan and G. J. Fang, Small, 2017, 13, 1601769 CrossRef PubMed.
- H. Liu, Z. Chen, H. Wang, F. Ye, J. Ma, X. Zheng, P. Gui, L. Xiong, J. Wen and G. Fang, J. Mater. Chem. A, 2019, 7, 10636–10643 RSC.
- S. Y. Park, M. Y. Baek, Y. Ju, D. H. Kim, C. S. Moon, J. H. Noh and H. S. Jung, J. Phys. Chem. Lett., 2018, 9, 5460–5467 CrossRef CAS PubMed.
- E. Wang, P. Chen, X. Yin, Y. Wu and W. Que, Sol. RRL, 2019, 3, 1900041 CrossRef.
- L. Xiong, M. Qin, C. Chen, J. Wen, G. Yang, Y. Guo, J. Ma, Q. Zhang, P. Qin and S. Li, Adv. Funct. Mater., 2018, 28, 1706276 CrossRef.
- Y. Zhao, J. Duan, H. Yuan, Y. Wang, X. Yang, B. He and Q. Tang, Sol. RRL, 2019, 3, 1800284 CrossRef.
- S. Vijayaraghavan, J. Wall, L. Li, G. Xing, Q. Zhang and F. Yan, Mater. Today Phys., 2020, 13, 100204 CrossRef.
- Z. Wang, T. Wu, L. Xiao, P. Qin, X. Yu, L. Ma, L. Xiong, H. Li, X. Chen and Z. Wang, J. Power Sources, 2021, 488, 229451 CrossRef CAS.
- Q. Jiang, L. Wang, C. Yan, C. Liu, Z. Guo and N. Wang, Eng. Sci., 2018, 1, 64–68 Search PubMed.
- J. Chen, H. Dong, L. Zhang, J. Li, F. Jia, B. Jiao, J. Xu, X. Hou, J. Liu and Z. Wu, J. Mater. Chem. A, 2020, 8, 2644–2653 RSC.
- L. S. Huang, X. W. Zhou, R. Xue, P. F. Xu, S. L. Wang, C. Xu, W. Zeng, Y. Xiong, H. Q. Sang and D. Liang, Nano-Micro Lett., 2020, 12 CAS.
- H. H. Niu, C. L. Fang, X. T. Wei, H. Wang, L. Wan, Y. Li, X. L. Mao, J. Z. Xu and R. Zhou, Dalton Trans., 2021, 50, 6477–6487 RSC.
- J. Duan, Q. Xiong, B. Feng, Y. Xu, J. Zhang and H. Wang, Appl. Surf. Sci., 2017, 391, 677–683 CrossRef CAS.
- W. Gong, H. Guo, H. Zhang, J. Yang, H. Chen, L. Wang, F. Hao and X. Niu, J. Mater. Chem. C, 2020, 8, 11638–11646 RSC.
- J. Liang, Z. Chen, G. Yang, H. Wang, F. Ye, C. Tao and G. Fang, ACS Appl. Mater. Interfaces, 2019, 11, 23152–23159 CrossRef CAS PubMed.
- X. Gong, Q. Sun, S. Liu, P. Liao, Y. Shen, C. Grätzel, S. M. Zakeeruddin, M. Grätzel and M. Wang, Nano Lett., 2018, 18, 3969–3977 CrossRef CAS PubMed.
- Q. Jiang, F. Liu, T. Li and T. Xu, J. Mater. Chem. C, 2014, 2, 618–621 RSC.
- J. Zhuang, P. Mao, Y. Luan, N. Chen, X. Cao, G. Niu, F. Jia, F. Wang, S. Cao and J. Wang, Adv. Funct. Mater., 2021, 2010385 CrossRef CAS.
- I. Mondal, G. Bahuguna, M. K. Ganesha, M. Verma, R. Gupta, A. K. Singh and G. U. Kulkarni, ACS Appl. Mater. Interfaces, 2020, 12, 54203–54211 CrossRef CAS PubMed.
- E. P. Nascimento, H. C. Firmino, A. M. Santos, H. B. Sales, V. D. Silva, D. A. Macedo, G. A. Neves, E. S. Medeiros and R. R. Menezes, J. Am. Ceram. Soc., 2021, 104, 1297–1308 CrossRef CAS.
- W. Ahmad, D. Liu, J. Wu, W. Ahmad, Y. Wang, P. Zhang, T. Zhang, H. Zheng, L. Chen, Z. D. Chen and S. Li, IEEE J. Photovolt., 2019, 9, 1273–1279 Search PubMed.
- Y. Huang, S. Li, C. Wu, S. Wang, C. Wang and R. Ma, Chem. Phys. Lett., 2020, 745, 137220 CrossRef CAS.
- Y. Qiang, Y. Xie, Y. Qi, P. Wei, H. Shi, C. Geng and H. Liu, Sol. Energy, 2020, 201, 523–529 CrossRef CAS.
- N. Zhou, Q. Cheng, L. Li and H. Zhou, J. Phys. D: Appl. Phys., 2018, 51, 394001 CrossRef.
- L. Xiong, M. Qin, G. Yang, Y. Guo, H. Lei, Q. Liu, W. Ke, H. Tao, P. Qin and S. Li, J. Mater. Chem. A, 2016, 4, 8374–8383 RSC.
- H. Chen, D. Liu, Y. Wang, C. Wang, T. Zhang, P. Zhang, H. Sarvari, Z. Chen and S. Li, Nanoscale Res. Lett., 2017, 12, 1–6 CrossRef PubMed.
- P. Sakthivel, S. Foo, M. Thambidurai, P. Harikesh, N. Mathews, R. Yuvakkumar, G. Ravi and C. Dang, J. Power Sources, 2020, 471, 228443 CrossRef CAS.
- H. Ye, Z. Liu, X. Liu, B. Sun, X. Tan, Y. Tu, T. Shi, Z. Tang and G. Liao, Appl. Surf. Sci., 2019, 478, 417–425 CrossRef CAS.
- Y. Bai, Y. Fang, Y. Deng, Q. Wang, J. Zhao, X. Zheng, Y. Zhang and J. Huang, ChemSusChem, 2016, 9, 2686–2691 CrossRef CAS PubMed.
- Q. Cao, Z. Li, J. Han, S. Wang, J. Zhu, H. Tang, X. Li and X. Li, Sol. RRL, 2019, 3, 1900333 CrossRef CAS.
- J. Bahadur, A. H. Ghahremani, B. Martin, T. Druffel, M. K. Sunkara and K. Pal, Org. Electron., 2019, 67, 159–167 CrossRef CAS.
- S. Akin, ACS Appl. Mater. Interfaces, 2019, 11, 39998–40005 CrossRef CAS PubMed.
- Z. Ma, W. Zhou, Z. Xiao, H. Zhang, Z. Li, J. Zhuang, C. Peng and Y. Huang, Org. Electron., 2019, 71, 98–105 CrossRef CAS.
- B. Roose and R. H. Friend, Adv. Mater. Interfaces, 2019, 6, 1801788 CrossRef.
- Y. W. Noh, J. H. Lee, I. S. Jin, S. H. Park and J. W. Jung, Nano Energy, 2019, 65, 104014 CrossRef CAS.
- X. Zhang, X. Liu, H. Ning, W. Yuan, Y. Deng, X. Zhang, S. Wang, J. Wang, R. Yao and J. Peng, Superlattices Microstruct., 2018, 123, 330–337 CrossRef CAS.
- E. Halvani Anaraki, A. Kermanpur, M. T. Mayer, L. Steier, T. Ahmed, S.-H. Turren-Cruz, J. Seo, J. Luo, S. M. Zakeeruddin, W. R. Tress, T. Edvinsson, M. Grätzel, A. Hagfeldt and J.-P. Correa-Baena, ACS Energy Lett., 2018, 3, 773–778 CrossRef CAS.
- X. Ren, D. Yang, Z. Yang, J. Feng, X. Zhu, J. Niu, Y. Liu, W. Zhao and S. F. Liu, ACS Appl. Mater. Interfaces, 2017, 9, 2421–2429 CrossRef CAS PubMed.
- S. Wang, W. Shen, J. Liu, T. Ouyang, Y. Wu, W. Li, M. Chen, P. Qi, Y. Lu and Y. Tang, Nanotechnology, 2021, 32, 145403 CrossRef CAS PubMed.
- Q. Liu, X. Zhang, C. Li, H. Lu, Z. Weng, Y. Pan, W. Chen, X.-C. Hang, Z. Sun and Y. Zhan, Appl. Phys. Lett., 2019, 115, 143903 CrossRef.
- J. Song, W. Zhang, D. Wang, K. Deng, J. Wu and Z. Lan, Sol. Energy, 2019, 185, 508–515 CrossRef CAS.
- Z. Xu, S. H. Teo, L. Gao, Z. Guo, Y. Kamata, S. Hayase and T. Ma, Org. Electron., 2019, 73, 62–68 CrossRef CAS.
- J. Tian, J. Zhang, X. Li, B. Cheng, J. Yu and W. Ho, Sol. RRL, 2020, 4, 2000090 CrossRef CAS.
- M. Hu, L. Zhang, S. She, J. Wu, X. Zhou, X. Li, D. Wang, J. Miao, G. Mi and H. Chen, Sol. RRL, 2020, 4, 1900331 CrossRef CAS.
- N. Li, J. Yan, Y. Ai, E. Jiang, L. Lin, C. Shou, B. Yan, J. Sheng and J. Ye, Sci. China Mater., 2020, 63, 207–215 CrossRef CAS.
- J. Dagar, S. Castro-Hermosa, G. Lucarelli, A. Zampetti, F. Cacialli and T. M. Brown, IEEE J. Photovolt., 2019, 9, 1309–1315 Search PubMed.
- H. Dong, S. Pang, Y. Xu, Z. Li, Z. Zhang, W. Zhu, D. Chen, H. Xi, Z. Lin and J. Zhang, ACS Appl. Mater. Interfaces, 2020, 12, 54703–54710 CrossRef CAS PubMed.
- Y. W. Noh, I. S. Jin, K. S. Kim, S. H. Park and J. W. Jung, J. Mater. Chem. A, 2020, 8, 17163–17173 RSC.
- D. Wang, C. Wu, W. Luo, X. Guo, B. Qu, L. Xiao and Z. Chen, ACS Appl. Energy Mater., 2018, 1, 2215–2221 CrossRef CAS.
- Y. Yang, T. Wang, Y. Zhang, X. Zhang, N. Li, P. Wang, Y. Qian, Q. Rong, L. Shui and G. Zhou, Sol. Energy, 2020, 196, 22–26 CrossRef CAS.
- H. Yi, D. Wang, M. A. Mahmud, F. Haque, M. B. Upama, C. Xu, L. Duan and A. Uddin, ACS Appl. Energy Mater., 2018, 1, 6027–6039 CrossRef.
- F. Guo, X. Sun, B. Liu, Z. Yang, J. Wei and D. Xu, Angew. Chem., Int. Ed., 2019, 58, 18460–18465 CrossRef CAS PubMed.
- S. S. Mali, J. V. Patil, H. Arandiyan and C. K. Hong, J. Mater. Chem. A, 2019, 7, 17516–17528 RSC.
- P. Wang, R. Li, B. Chen, F. Hou, J. Zhang, Y. Zhao and X. Zhang, Adv. Mater., 2020, 32, 1905766 CrossRef CAS PubMed.
- F. Wang, Y. Zhang, M. Yang, J. Du, L. Xue, L. Yang, L. Fan, Y. Sui, J. Yang and X. Zhang, Nano Energy, 2019, 63, 103825 CrossRef CAS.
- J. Ye, Y. Li, A. A. Medjahed, S. Pouget, D. Aldakov, Y. Liu and P. Reiss, Small, 2021, 17, 2005671 CrossRef CAS PubMed.
- W. Chen, Y. Wu, Y. Yue, J. Liu, W. Zhang, X. Yang, H. Chen, E. Bi, I. Ashraful and M. Grätzel, Science, 2015, 350, 944–948 CrossRef CAS PubMed.
- Y. Hou, X. Du, S. Scheiner, D. P. McMeekin, Z. Wang, N. Li, M. S. Killian, H. Chen, M. Richter and I. Levchuk, Science, 2017, 358, 1192–1197 CrossRef CAS PubMed.
- O. Malinkiewicz, A. Yella, Y. H. Lee, G. M. Espallargas, M. Graetzel, M. K. Nazeeruddin and H. J. Bolink, Nat. Photonics, 2014, 8, 128–132 CrossRef CAS.
- Y. Shao, Z. Xiao, C. Bi, Y. Yuan and J. Huang, Nat. Commun., 2014, 5, 1–7 Search PubMed.
- F. Xie, C.-C. Chen, Y. Wu, X. Li, M. Cai, X. Liu, X. Yang and L. Han, Energy Environ. Sci., 2017, 10, 1942–1949 RSC.
- X. Zheng, B. Chen, J. Dai, Y. Fang, Y. Bai, Y. Lin, H. Wei, X. C. Zeng and J. Huang, Nat. Energy, 2017, 2, 1–9 Search PubMed.
- W. Ke, D. Zhao, C. Xiao, C. Wang, A. J. Cimaroli, C. R. Grice, M. Yang, Z. Li, C.-S. Jiang, M. Al-Jassim, K. Zhu, M. G. Kanatzidis, G. Fang and Y. Yan, J. Mater. Chem. A, 2016, 4, 14276–14283 RSC.
- K. Liu, S. Chen, J. Wu, H. Zhang, M. Qin, X. Lu, Y. Tu, Q. Meng and X. Zhan, Energy Environ. Sci., 2018, 11, 3463–3471 RSC.
- J. Wang, K. Datta, C. H. Weijtens, M. M. Wienk and R. A. Janssen, Adv. Funct. Mater., 2019, 29, 1905883 CrossRef CAS.
- K. Liu, S. Chen, J. Wu, H. Zhang, M. Qin, X. Lu, Y. Tu, Q. Meng and X. Zhan, Energy Environ. Sci., 2018, 11, 3463–3471 RSC.
- C. Tian, K. Lin, J. Lu, W. Feng, P. Song, L. Xie and Z. Wei, Small Methods, 2020, 4, 1900476 CrossRef CAS.
- J. A. Raiford, C. C. Boyd, A. F. Palmstrom, E. J. Wolf, B. A. Fearon, J. J. Berry, M. D. McGehee and S. F. Bent, Adv. Energy Mater., 2019, 9, 1902353 CrossRef CAS.
- A. F. Palmstrom, J. A. Raiford, R. Prasanna, K. A. Bush, M. Sponseller, R. Cheacharoen, M. C. Minichetti, D. S. Bergsman, T. Leijtens and H. P. Wang, Adv. Energy Mater., 2018, 8, 1800591 CrossRef.
- R. Cheacharoen, N. Rolston, D. Harwood, K. A. Bush, R. H. Dauskardt and M. D. McGehee, Energy Environ. Sci., 2018, 11, 144–150 RSC.
- N. Rolston, B. L. Watson, C. D. Bailie, M. D. McGehee, J. P. Bastos, R. Gehlhaar, J.-E. Kim, D. Vak, A. T. Mallajosyula and G. Gupta, Extreme Mech. Lett., 2016, 9, 353–358 CrossRef.
- J. Liu, Y. Guo, M. Zhu, Y. Li and X. Li, J. Power Sources, 2020, 476, 228648 CrossRef CAS.
- Q. Luo, H. Ma, F. Hao, Q. Hou, J. Ren, L. Wu, Z. Yao, Y. Zhou, N. Wang, K. Jiang, H. Lin and Z. Guo, Adv. Funct. Mater., 2017, 27, 1703068 CrossRef.
- Y. Wang, C. Duan, J. Li, W. Han, M. Zhao, L. Yao, Y. Wang, C. Yan and T. Jiu, ACS Appl. Mater. Interfaces, 2018, 10, 20128–20135 CrossRef CAS PubMed.
- P. Fabiola Mendez, S. K. M. Muhammed, E. M. Barea, S. Masi and I. Mora-Sero, Sol. RRL, 2019, 3, 1900191 CrossRef.
- K. Jung, J. Kim, S. Ko, J. W. Choi, K. C. Kim, S.-G. Lee and M.-J. Lee, J. Mater. Sci. Technol., 2020, 59, 195–202 CrossRef.
- F. Li, M. Xu, X. Ma, L. Shen, L. Zhu, Y. Weng, G. Yue, F. Tan and C. Chen, Nanoscale Res. Lett., 2018, 13, 1–7 CrossRef PubMed.
- J. Xie, K. Huang, X. Yu, Z. Yang, K. Xiao, Y. Qiang, X. Zhu, L. Xu, P. Wang, C. Cui and D. Yang, ACS Nano, 2017, 11, 9176–9182 CrossRef CAS PubMed.
- Y. Ai, W. Liu, C. Shou, J. Yan, N. Li, Z. Yang, W. Song, B. Yan, J. Sheng and J. Ye, Sol. Energy, 2019, 194, 541–547 CrossRef CAS.
- Z. Liu, K. Deng, J. Hu and L. Li, Angew. Chem., Int. Ed., 2019, 58, 11497–11504 CrossRef CAS PubMed.
- E. H. Jung, B. Chen, K. Bertens, M. Vafaie, S. Teale, A. Proppe, Y. Hou, T. Zhu, C. Zheng and E. H. Sargent, ACS Energy Lett., 2020, 5, 2796–2801 CrossRef CAS.
- C. Huang, P. Lin, N. Fu, K. Sun, M. Ye, C. Liu, X. Zhou, L. Shu, X. Hao, B. Xu, X. Zeng, Y. Wang and S. Ke, J. Mater. Chem. A, 2018, 6, 22086–22095 RSC.
- D. Yang, R. Yang, K. Wang, C. Wu, X. Zhu, J. Feng, X. Ren, G. Fang, S. Priya and S. F. Liu, Nat. Commun., 2018, 9, 1–11 CrossRef PubMed.
- Z. Zhang, J. Zhang, N. Chen and L. Qu, Energy Environ. Sci., 2012, 5, 8869–8890 RSC.
- J. Chen, H. Dong, L. Zhang, J. Li, F. Jia, B. Jiao, J. Xu, X. Hou, J. Liu and Z. Wu, J. Mater. Chem. A, 2020, 8, 2644–2653 RSC.
- W. Hui, Y. Yang, Q. Xu, H. Gu, S. Feng, Z. Su, M. Zhang, J. Wang, X. Li and J. Fang, Adv. Mater., 2020, 32, 1906374 CrossRef CAS PubMed.
- J. Jia, C. Qian, Y. Dong, Y. F. Li, H. Wang, M. Ghoussoub, K. T. Butler, A. Walsh and G. A. Ozin, Chem. Soc. Rev., 2017, 46, 4631–4644 RSC.
- S. Y. Abate, D.-C. Huang and Y.-T. Tao, Org. Electron., 2020, 78, 105583 CrossRef CAS.
- F. Ali, C. Roldán-Carmona, M. Sohail and M. K. Nazeeruddin, Adv. Energy Mater., 2020, 10, 2002989 CrossRef CAS.
- F. Han, G. Hao, Z. Wan, J. Luo, J. Xia and C. Jia, Electrochim. Acta, 2019, 296, 75–81 CrossRef CAS.
- S. Y. Kim, S. J. Cho, S. E. Byeon, X. He and H. J. Yoon, Adv. Energy Mater., 2020, 10, 2002606 CrossRef CAS.
- G. Yang, C. Wang, H. Lei, X. Zheng, P. Qin, L. Xiong, X. Zhao, Y. Yan and G. Fang, J. Mater. Chem. A, 2017, 5, 1658–1666 RSC.
- J. Yan, Z. Lin, Q. Cai, X. Wen and C. Mu, ACS Appl. Energy Mater., 2020, 3, 3504–3511 CrossRef CAS.
- G.-W. Kim, Y. Choi, H. Choi, J. Min, T. Park and S. Song, J. Mater. Chem. A, 2020, 8, 21721–21728 RSC.
- H. Anizelli, T. W. David, P. Tyagi, E. Laureto and J. Kettle, Sol. Energy, 2020, 203, 157–163 CrossRef CAS.
- G. Tumen-Ulzii, T. Matsushima, D. Klotz, M. R. Leyden, P. Wang, C. Qin, J.-W. Lee, S.-J. Lee, Y. Yang and C. Adachi, Communications Materials, 2020, 1, 1–7 CrossRef.
- P. Huang, Q. Chen, K. Zhang, L. Yuan, Y. Zhou, B. Song and Y. Li, J. Mater. Chem. A, 2019, 7, 6213–6219 RSC.
- W. Wang, Z. Su, B. Sun, L. Tao, H. Gu, W. Hui, Q. Wei, W. Shi, X. Gao and Y. Xia, Adv. Mater. Interfaces, 2021, 8, 2001683 CrossRef CAS.
- Y. Wang, P. Xiang, A. Ren, H. Lai, Z. Zhang, Z. Xuan, Z. Wan, J. Zhang, X. Hao and L. Wu, ACS Appl. Mater. Interfaces, 2020, 12, 53973–53983 CrossRef CAS PubMed.
- L. Yang, Y. Dall'Agnese, K. Hantanasirisakul, C. E. Shuck, K. Maleski, M. Alhabeb, G. Chen, Y. Gao, Y. Sanehira and A. K. Jena, J. Mater. Chem. A, 2019, 7, 5635–5642 RSC.
- K. Hantanasirisakul and Y. Gogotsi, Adv. Mater., 2018, 30, 1804779 CrossRef PubMed.
- M. Naguib, M. Kurtoglu, V. Presser, J. Lu, J. J. Niu, M. Heon, L. Hultman, Y. Gogotsi and M. W. Barsoum, Adv. Mater., 2011, 23, 4248–4253 CrossRef CAS PubMed.
- H. Xu, A. B. Ren, J. Wu and Z. M. Wang, Adv. Funct. Mater., 2020, 30, 2000907 CrossRef CAS.
- A. Agresti, A. Pazniak, S. Pescetelli, A. Di Vito, D. Rossi, A. Pecchia, M. Auf der Maur, A. Liedl, R. Larciprete, D. V. Kuznetsov, D. Saranin and A. Di Carlo, Nat. Mater., 2019, 18, 1228–1234 CrossRef CAS PubMed.
- Z. Guo, L. Gao, Z. Xu, S. Teo, C. Zhang, Y. Kamata, S. Hayase and T. Ma, Small, 2018, 14, e1802738 CrossRef PubMed.
- X. Liu, P. Li, Y. Zhang, X. Hu, Y. Duan, F. Li, D. Li, G. Shao and Y. Song, J. Power Sources, 2019, 413, 459–466 CrossRef CAS.
- X. Meng, X. Cui, M. Rager, S. Zhang, Z. Wang, J. Yu, Y. W. Harn, Z. Kang, B. K. Wagner and Y. Liu, Nano Energy, 2018, 52, 123–133 CrossRef CAS.
- F. Zhang, X. Yang, M. Cheng, J. Li, W. Wang, H. Wang and L. Sun, J. Mater. Chem. A, 2015, 3, 24272–24280 RSC.
- Q. Guo, F. Yuan, B. Zhang, S. Zhou, J. Zhang, Y. Bai, L. Fan, T. Hayat, A. Alsaedi and Z. a. Tan, Nanoscale, 2019, 11, 115–124 RSC.
- I. Jeon, A. Shawky, S. Seo, Y. Qian, A. Anisimov, E. I. Kauppinen, Y. Matsuo and S. Maruyama, J. Mater. Chem. A, 2020, 8, 11141–11147 RSC.
- Z. Li, C. Liu, G. Ren, W. Han, L. Shen and W. Guo, Sol. RRL, 2020, 4, 1900369 CrossRef CAS.
- Q. Luo, H. Ma, Q. Hou, Y. Li, J. Ren, X. Dai, Z. Yao, Y. Zhou, L. Xiang and H. Du, Adv. Funct. Mater., 2018, 28, 1706777 CrossRef.
- A. Mei, X. Li, L. Liu, Z. Ku, T. Liu, Y. Rong, M. Xu, M. Hu, J. Chen, Y. Yang, M. Gratzel and H. Han, Science, 2014, 345, 295–298 CrossRef CAS PubMed.
- M. Paszkiewicz-Gawron, E. Kowalska, M. Endo-Kimura, J. Zwara, A. Pancielejko, K. Wang, W. Lisowski, J. Łuczak, A. Zaleska-Medynska and E. Grabowska-Musiał, Appl. Surf. Sci., 2021, 541, 148425 CrossRef CAS.
- S. Wang, Y. Zhu, B. Liu, C. Wang and R. Ma, J. Mater. Chem. A, 2019, 7, 5353–5362 RSC.
- S. Zhang, H. Si, W. Fan, M. Shi, M. Li, C. Xu, Z. Zhang, Q. Liao, A. Sattar and Z. Kang, Angew. Chem., Int. Ed., 2020, 59, 11573–11582 CrossRef CAS PubMed.
- X. Zhao, L. Tao, H. Li, W. Huang, P. Sun, J. Liu, S. Liu, Q. Sun, Z. Cui, L. Sun, Y. Shen, Y. Yang and M. Wang, Nano Lett., 2018, 18, 2442–2449 CrossRef CAS PubMed.
- A. F. Castro-Méndez, J. Hidalgo and J. P. Correa-Baena, Adv. Energy Mater., 2019, 9, 1901489 CrossRef.
- B. Chen, M. Yang, S. Priya and K. Zhu, J. Phys. Chem. Lett., 2016, 7, 905–917 CrossRef CAS PubMed.
- H.-W. Chen, N. Sakai, M. Ikegami and T. Miyasaka, J. Phys. Chem. Lett., 2015, 6, 164–169 CrossRef CAS PubMed.
- J. Wei, Y. Zhao, H. Li, G. Li, J. Pan, D. Xu, Q. Zhao and D. Yu, J. Phys. Chem. Lett., 2014, 5, 3937–3945 CrossRef CAS PubMed.
- P. Calado, A. M. Telford, D. Bryant, X. Li, J. Nelson, B. C. O'Regan and P. R. F. Barnes, Nat. Commun., 2016, 7, 13831 CrossRef CAS PubMed.
- Z. Li, C. Xiao, Y. Yang, S. P. Harvey, D. H. Kim, J. A. Christians, M. Yang, P. Schulz, S. U. Nanayakkara, C.-S. Jiang, J. M. Luther, J. J. Berry, M. C. Beard, M. M. Al-Jassim and K. Zhu, Energy Environ. Sci., 2017, 10, 1234–1242 RSC.
- T. Zhang, H. Chen, Y. Bai, S. Xiao, L. Zhu, C. Hu, Q. Xue and S. Yang, Nano Energy, 2016, 26, 620–630 CrossRef CAS.
- T. Zhang, C. Hu and S. Yang, Small Methods, 2020, 4, 1900552 CrossRef CAS.
- Q. Jiang, M. Chen, J. Li, M. Wang, X. Zeng, T. Besara, J. Lu, Y. Xin, X. Shan and B. Pan, ACS Nano, 2017, 11, 1073–1079 CrossRef CAS PubMed.
- S. Cao, H. Wang, H. Li, J. Chen and Z. Zang, Chem. Eng. J., 2020, 394, 124903 CrossRef CAS.
- Q. JIANG, Nat. Energy, 2016, 2, 1–7 CrossRef.
- K. Jung, W.-S. Chae, Y. C. Park, N.-G. Park and M.-J. Lee, Chem. Eng. J., 2021, 409, 128215 CrossRef CAS.
- J. Chen, J. Zhang, C. Huang, Z. Bi, X. Xu and H. Yu, Chem. Eng. J., 2021, 410, 128436 CrossRef CAS.
- L. Lin, T. W. Jones, J. T. W. Wang, A. Cook, N. D. Pham, N. W. Duffy, B. Mihaylov, M. Grigore, K. F. Anderson and B. C. Duck, Small, 2020, 16, 1901466 CrossRef CAS PubMed.
- D. Aidarkhanov, Z. Ren, C.-K. Lim, Z. Yelzhanova, G. Nigmetova, G. Taltanova, B. Baptayev, F. Liu, S. H. Cheung and M. Balanay, Sol. Energy Mater. Sol. Cells, 2020, 215, 110648 CrossRef CAS.
- H. Wang, F. Li, P. Wang, R. Sun, W. Ma, M. Chen, W. Miao, D. Liu and T. Wang, Adv. Energy Mater., 2020, 10, 2000615 CrossRef CAS.
- X. Ye, H. Cai, J. Su, J. Yang, J. Ni, J. Li and J. Zhang, J. Mater. Sci. Technol., 2020, 61, 213–220 CrossRef.
- D.-Y. Son, S.-G. Kim, J.-Y. Seo, S.-H. Lee, H. Shin, D. Lee and N.-G. Park, J. Am. Chem. Soc., 2018, 140, 1358–1364 CrossRef CAS PubMed.
- Z. Tang, S. Uchida, T. Bessho, T. Kinoshita, H. Wang, F. Awai, R. Jono, M. M. Maitani, J. Nakazaki and T. Kubo, Nano Energy, 2018, 45, 184–192 CrossRef CAS.
- M. Gratzel, Nature, 2001, 414, 338–345 CrossRef CAS PubMed.
- Q. Jiang, D. Rebollar, J. Gong, E. L. Piacentino, C. Zheng and T. Xu, Angew. Chem., 2015, 127, 7727–7730 CrossRef.
- M. Zhang, F. Wu, D. Chi, K. Shi and S. Huang, Mater. Adv., 2020, 1, 617–624 RSC.
- X. Huang, J. Du, X. Guo, Z. Lin, J. Ma, J. Su, L. Feng, C. Zhang, J. Zhang and J. Chang, Sol. RRL, 2020, 4, 1900336 CrossRef CAS.
- Y. Wang, C. Duan, X. Zhang, N. Rujisamphan, Y. Liu, Y. Li, J. Yuan and W. Ma, ACS Appl. Mater. Interfaces, 2020, 12, 31659–31666 CrossRef CAS PubMed.
- Z. Chen, Z. Zhang, J. Yang, W. Chen, Z. L. Teh, D. Wang, L. Yuan, J. Zhang, J. A. Stride and G. J. Conibeer, J. Mater. Chem. C, 2018, 6, 9861–9866 RSC.
- M. Zhu, X. Liu, S. Liu, C. Chen, J. He, W. Liu, J. Yang, L. Gao, G. Niu, J. Tang and J. Zhang, ACS Appl. Mater. Interfaces, 2020, 12, 2566–2571 CrossRef CAS PubMed.
- N. H. Nickel, F. Lang, V. V. Brus, O. Shargaieva and J. Rappich, Adv. Electron. Mater., 2017, 3, 1700158 CrossRef.
- H. Shan, E. Rezaee, X. Leng, X. Wang, Q. Chen and Z. X. Xu, ChemSusChem, 2018, 11, 3000–3006 CrossRef CAS PubMed.
- H. Hussain, G. Tocci, T. Woolcot, X. Torrelles, C. Pang, D. Humphrey, C. Yim, D. Grinter, G. Cabailh and O. Bikondoa, Nat. Mater., 2017, 16, 461–466 CrossRef CAS PubMed.
- B. Roose, J.-P. C. Baena, K. C. Gödel, M. Graetzel, A. Hagfeldt, U. Steiner and A. Abate, Nano Energy, 2016, 30, 517–522 CrossRef CAS.
- B. Roose, C. M. Johansen, K. Dupraz, T. Jaouen, P. Aebi, U. Steiner and A. Abate, J. Mater. Chem. A, 2018, 6, 1850–1857 RSC.
- R. Liu, L. Wang, Y. Fan, Z. Li and S. Pang, RSC Adv., 2020, 10, 11551–11556 RSC.
- P. Hang, J. Xie, G. Li, Y. Wang, D. Fang, Y. Yao, D. Xie, C. Cui, K. Yan and J. Xu, Iscience, 2019, 21, 217–227 CrossRef CAS PubMed.
- M. M. Tavakoli, F. Giordano, S. M. Zakeeruddin and M. Gratzel, Nano Lett., 2018, 18, 2428–2434 CrossRef CAS PubMed.
- D. P. McMeekin, G. Sadoughi, W. Rehman, G. E. Eperon, M. Saliba, M. T. Hörantner, A. Haghighirad, N. Sakai, L. Korte and B. Rech, Science, 2016, 351, 151–155 CrossRef CAS PubMed.
- J. Chen, X. Zhao, S.-G. Kim and N.-G. Park, Adv. Mater., 2019, 31, 1902902 CrossRef PubMed.
- K. Choi, J. Lee, H. I. Kim, C. W. Park, G.-W. Kim, H. Choi, S. Park, S. A. Park and T. Park, Energy Environ. Sci., 2018, 11, 3238–3247 RSC.
- Y. Guo, H. Lei, C. Wang, J. Ma, C. Chen, X. Zheng, G. Yang, L. Xiong and Z. Tan, Sol. RRL, 2020, 4, 1900482 CrossRef CAS.
- S. Mu, Q. Ye, X. Zhang, S. Huang and J. You, Front. Optoelectron., 2020, 13, 265–271 CrossRef.
- C. Tian, K. Lin, J. Lu, W. Feng, P. Song, L. Xie and Z. Wei, Small Methods, 2020, 4, 1900476 CrossRef CAS.
- W. Zhang, Y. Li, X. Liu, D. Tang, X. Li and X. Yuan, Chem. Eng. J., 2020, 379, 122298 CrossRef CAS.
- Y.-W. Jang, S. Lee, K. M. Yeom, K. Jeong, K. Choi, M. Choi and J. H. Noh, Nat. Energy, 2021, 6, 63–71 CrossRef CAS.
- Q. Dong, M. Chen, Y. Liu, F. T. Eickemeyer, W. Zhao, Z. Dai, Y. Yin, C. Jiang, J. Feng, S. Jin, S. Liu, S. M. Zakeeruddin, M. Grätzel, N. P. Padture and Y. Shi, Joule, 2021, 5, 1587–1601 CrossRef CAS.
- Q. Dong, C. Zhu, M. Chen, C. Jiang, J. Guo, Y. Feng, Z. Dai, S. K. Yadavalli, M. Hu, X. Cao, Y. Li, Y. Huang, Z. Liu, Y. Shi, L. Wang, N. P. Padture and Y. Zhou, Nat. Commun., 2021, 12, 973 CrossRef CAS PubMed.
- N. Ren, B. Chen, R. Li, P. Wang, S. Mazumdar, B. Shi, C. Zhu, Y. Zhao and X. Zhang, Sol. RRL, 2021, 5, 2000795 CrossRef CAS.
- Y. Zhou, S. Yang, X. Yin, J. Han, M. Tai, X. Zhao, H. Chen, Y. Gu, N. Wang and H. Lin, J. Mater. Chem. A, 2019, 7, 1878–1888 RSC.
- Q. Dong, J. Li, Y. Shi, M. Chen, L. K. Ono, K. Zhou, C. Zhang, Y. Qi, Y. Zhou and N. P. Padture, Adv. Energy Mater., 2019, 9, 1900834 CrossRef.
- D.-K. Lee, D.-N. Jeong, T. K. Ahn and N.-G. Park, ACS Energy Lett., 2019, 4, 2393–2401 CrossRef CAS.
- K.-S. Lim, D.-K. Lee, J.-W. Lee and N.-G. Park, J. Mater. Chem. A, 2020, 8, 9345–9354 RSC.
- B. Taheri, F. De Rossi, G. Lucarelli, L. A. Castriotta, A. Di Carlo, T. M. Brown and F. Brunetti, ACS Appl. Energy Mater., 2021, 4, 4507–4518 CrossRef CAS PubMed.
- S. Tian, J. Li, S. Li, T. Bu, Y. Mo, S. Wang, W. Li and F. Huang, Sol. Energy, 2019, 183, 386–391 CrossRef CAS.
- T. Bu, X. Liu, J. Li, W. Huang, Z. Wu, F. Huang, Y.-B. Cheng and J. Zhong, Sol. RRL, 2020, 4, 1900263 CrossRef CAS.
- G. Jang, H. C. Kwon, S. Ma, S. C. Yun, H. Yang and J. Moon, Adv. Energy Mater., 2019, 9, 1901719 CrossRef.
|
This journal is © The Royal Society of Chemistry 2021 |