An integrated biorefinery to produce 5-(hydroxymethyl)furfural and alternative fuel precursors from macroalgae and spent coffee grounds†
Received
27th July 2021
, Accepted 31st October 2021
First published on 10th November 2021
Abstract
5-Hydroxymethylfurfural (HMF) is a promising platform chemical produced from the dehydration of C6 sugars, that is a precursor for a range of renewable fuels and polymers. In this study, an integrated macroalgal biorefinery was designed to produce an array of products including HMF, hydrothermal liquefaction (HTL) biocrude and biochar. In this process two different species of macroalgae, Ulva lactuca and Chorda filum, were investigated and co-processed with spent coffee grounds to assess if such blends could be effectively used, with the spent coffee grounds mitigating for lower macroalgae availability throughout the year. U. lactuca and the spent coffee ground blends were effectively used in a biorefinery design for the production of HMF. Interestingly, blends yielded higher amounts of HMF (35–47 g per kg of dry biomass processed) than the separate components alone. This is presumably due to the elevated amount of C6 sugars being available from the macroalgae, coupled with the presence of lipids from the coffee grounds. The lipids likely form a separate organic layer in the dehydration reaction, into which the HMF migrates after being formed in the aqueous fraction, halting further dehydration reactions to levulinic acid. The HTL on the resultant solids from dehydration yielded a relatively similar amount of biocrude (68–78 g per kg of dry biomass) compared to spent coffee grounds (SCG) (90 g per kg of dry biomass). However, the C. filum biorefinery yielded far lower biocrude and HMF, presumably due to the lower lipid and C6 sugar content in this feedstock. Overall, an HMF biorefinery from macroalgae is plausible, with spent coffee grounds being a highly suitable material to make up for seasonal availability. However, the large difference in yields from macroalgal species demonstrates the importance of high lipid content, alongside higher C6 sugar composition, in the macroalgal feedstock.
Introduction
Macroalgae is a promising feedstock for the next generation of biorefineries as it does not compete with food crops, has a higher rate of carbon dioxide fixation than land crops,1 has no freshwater requirement and is simple to process.2 In addition, its cultivation can help to alleviate the eutrophication in seas and oceans and aid in carbon capture and sequestration.3 There are over 30 million tonnes currently cultivated worldwide,4,5 predominantly in China and India. The UK has one of the most extensive coastlines in Europe (approximately 12
500 km) and the ideal water temperature for seaweed production. These conditions and the underdeveloped market present a large opportunity for further development.6 There are a wide range of seaweed species growing around the UK, that inevitably, have different properties and components depending on the type, habitat, cultivation method and harvest time.7,8 However, the predominant feature across almost all species are the elevated carbohydrate levels (65–75%).9
Several authors have demonstrated that macroalgae can be used in the hydrothermal liquefaction process.10 Raikova et al. presented a comprehensive study on a broad range of macroalgae species from the UK and the ideal hydrothermal liquefaction conditions to convert these into bio-crude and nutrient partition into the aqueous phase.11 In a further study the authors also combined plastics present in the ocean with the macroalgae demonstrating that this led to a higher biocrude heating value.12
However, the use of macroalgae in an HTL-based industry presents two key issues. The first is the relatively low production of crude from the majority of species when compared to microalgae.13 This is primarily because of the elevated carbohydrates levels (relative to the more proteinaceous microalgae), that predominantly breakdown into insoluble biochar, a lower quality fuel than the biocrude.14–16
Indeed, the majority of research on macroalgal valorisation has focussed on the conversion of the carbohydrate fraction, mainly through fermentation to ethanol17 and alternative cellular products.18 However, the saccharides must first be extracted and processed into fermentable sugars. There are a high number of competing pathways for carbon in any fermentation and the breadth of sugars that any one organism would need to metabolise make these routes challenging. A simpler, more targeted approach, is the acid catalysed breakdown of the macroalgal saccharides to produce 5-hydroxymethyl furfural (HMF). HMF is a highly promising chemical building block, with a forecasted market of 61 million USD by 2024.19,20 Its production from carbohydrates and the potential to be converted into high value biofuels make this molecule an important intermediate between the carbohydrate and petroleum-based industry.21 In addition the chemical processing of biomass tends to have far lower capital costs than the biochemical processing of a similar size.22 HMF from biomass is usually produced through a sequence of steps: (1) depolymerisation of the feedstock macromolecules into sugars; (2) isomerisation of glucose to fructose; (3) acid-catalysed dehydration of fructose into HMF.23 HMF has the potential to be converted into dimethylfuran (DMF), a biofuel with high energy density; and 2,5-furandicarboxylic acid (FDCA) a building block used in the production of polyethylene 2,5-furandicarboxylate (PEF), an emerging biobased polymer proposed to replace polyethylene terephthalate (PET).24,25 The production of HMF from macroalgae has been demonstrated including from isolated agar26 and the red macroalga Kappaphycus alvarezeii.27 In the latter, Lee et al. demonstrated the production of glucose, galactose, levulinic acid and HMF from the red seaweed in an acid-catalysed hydrothermal process. The authors reached an HMF concentration of 3.02 g L−1. Jeon and Park studied the optimal conditions for the production of glucose, galactose and levulinic acid from the red algae Gelidium amansii.28 In one of their many different studied conditions, the highest HMF concentration achieved was 4.49 g L−1 using an acid-catalysed hydrothermal process at 130 °C for 30 min. Similarly, Kholiya et al. described the extraction of agar/agarose from seaweed producing an aqueous extract followed by its conversion into HMF and levulinic acid.29 In an alternative approach, Gonzales et al. demonstrated that HMF can be sequestrated using granular activated carbon as an adsorbent from hydrolysates from lignocellulose and algal biomass.30
Another key issue is the seasonal growth of macroalgae, which does not lend itself to an effective all year supply, thereby limiting the size or scope of a potential biorefinery.31–34 Recent studies have shown that blending with alternative biomass sources can be used to even out supply, and produce products all year round. For example, Jin et al. showed that co-liquefaction of microalgae and macroalgae was possible, and even increased the bio-crude energy heating value compared to when the feedstocks were processed separately.35 Similarly, we recently demonstrated that microalgal seasonality can be effectively addressed by blending this feedstock with spent coffee grounds (SCG) in periods where the microalgae production is lower during the colder parts of the year.36 In this biorefinery set-up the saccharides were extracted and fermented before the resulting stillage was processed through HTL. Spent coffee grounds are a promising material for bioprocessing, which are available all year round, relatively stable to store, with the worldwide production of SCG being approximately 10 million tonnes in 2019.37 The composition of SCG varies but, similarly to macroalgae, they contain high carbohydrates (42–55 w/w %) with a similar C6 composition.38 We recently demonstrated the suitability of producing HMF from spent coffee grounds in an integrated biorefinery design using an organosolv fractionation to isolate cellulose.23
In this investigation therefore, we aimed to combine these approaches and address two fundamental issues impeding the development of macroalgal biorefineries. To this end, an integrated approach to increase the atom efficiency and produce both HMF and HTL products was demonstrated, co-processing the biomass with SCG to even out seasonality issues and allow steady production all year round.
Experimental
Materials
Spent coffee grounds were acquired from a local café at the University of Bath. A sample was weighed and placed in the oven at 60 °C. After two days the sample was weighed, and moisture content determined.
Ulva lactuca and Chorda filum were sampled from Broadsands Beach, Paignton, UK. Ulva lactuca and Chorda filum were sampled on the 30/07/2019 and 05/08/2019, respectively. Both macroalgae were washed in freshwater, and freeze dried prior to storage.
In addition to the ‘pure’ feedstocks of U. lactuca, C. filum and spent coffee grounds, two blends were prepared for each macroalgae. The blend with 40% macroalga and 60% SCG simulates a season when there is less production of macroalgae (possibly winter – depending on the strain) and the blend with 60% macroalgae and 40% SCG simulate an intermediate season between maximum and minimum macroalgae production (possibly spring and/or autumn). Therefore, the seven feedstocks studied are as follows:
• Pure Ulva lactuca – UL.
• 60% UL + 40% SCG – UL0.6 + SCG0.4.
• 40% UL + 60% SCG – UL0.4 + SCG0.6.
• Pure spent coffee grounds – SCG.
• 40% C. filum + 60% SCG – CF0.4 + SCG0.6.
• 60% C. filum + 40% SCG – CF0.6 + SCG0.4.
• Pure Chorda filum – CF.
Acid dehydration
20 g (dry weight) of feedstock were added to a 300 mL Parr reactor (Parr Company Moline, IL, USA, 4560 mini reactors), followed by the addition of a mixture of 2% (w/w) sulphuric acid (from Sigma-Aldrich) in deionised water to make up a total of 100 g. The reactor and its contents were weighed before the reaction. Agitation was initiated and the reactor heated to 155 °C, at which point the temperature was held for 15 minutes. The reaction mixture was cooled rapidly over 20 minutes using the in-built cooling system operating at −4 °C. The reactor and its contents were weighed to determine gas losses. All the seven feedstocks/scenarios studied were repeated at least three times and the standard deviation calculated.
HMF extraction
The solids from the reactor were separated through filtration. The HMF was extracted from the filtrate in a 500 mL separatory funnel, in a 100 mL using a mixture of dichloromethane (DCM) and 2-butanol (50
:
50 w/w %). Both phases (aqueous and organic phase containing the HMF fraction) were collected separately for further analysis. The recovery ratio of HMF in the extraction is calculated as follows: | 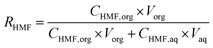 | (1) |
where CHMF,org and CHMF,aq are the concentration of HMF in the organic and aqueous fraction, respectively, in g L−1. Vorg and Vaq are the volume of the organic and aqueous fraction, respectively.
Second acid dehydration
The aqueous phase collected from the HMF extraction was combined with the solids obtained from filtration upstream from the original HMF extraction. This slurry was then added to the reactor, H2SO4 was added and the same reaction procedure was used under the same conditions to produce a further amount of HMF. The aqueous, organic and solids were separated following the procedure given above. The solid fraction was removed, washed in solvent and dried overnight in an oven at 40 °C to reduce moisture content to residual quantities before being used in the hydrothermal liquefaction.
Hydrothermal liquefaction (HTL)
HTL reactions were performed in a 50 mL stainless steel batch reactor, equipped with a pressure gauge, pressure relief valve and a needle valve. 3 g of dried solids were weighed, loaded and mixed with 15 g of deionised water into an HTL reactor. The reactor was sealed and placed in a furnace pre-heated to 800 °C. Temperature was closely monitored using a thermocouple until it reached 350 °C (approximately between 150 and 180 bar). At this temperature the reactor was removed from the furnace and left to cool. Gas phase was measured and collected from the needle valve (using water displacement technique). The contents were separated through a pre-weighed filter paper. The filtrate (aqueous phase) was poured through the funnel into a pre-weighed vial. The vial and the filtrate obtained were weighed for total aqueous fraction weight determination. An aliquot of the aqueous fraction was oven-dried at 60 °C to determine the aqueous fraction residue yield. The reactor and the filtered solids were then thoroughly washed (using the same filter paper) with chloroform into a pre-weighed round bottom flask until the filtrate ran clear. The chloroform was removed in vacuo. On solvent removal, the flask was weighed and the biocrude fraction gravimetric yield was obtained. Solids were dried in an oven at 60 °C. Filtered solids were weighed for biochar gravimetric yield determination.
Carbohydrates and levulinic acid analysis
Aqueous and organic fractions obtained after the first and second extractions were filtered and analysed for carbohydrate content using a high performance liquid chromatography (HPLC), from Agilent Technologies, equipped with an Aminex HPX-87H organic acids column (300 mm × 7.88 mm, Bio-Rad Laboratories) and a refractive index detector (RID) was used to quantify the carbohydrates in this study. 5 mM H2SO4 solution was used as mobile phase at a flow rate of 0.6 mL min−1. Column was heated up to 65 °C. Mobile phase was prepared using sulphuric acid provided from Sigma-Aldrich.
HMF and furfural analysis
An HPLC (Agilent Technologies) equipped with a diode-array detector (DAD) and an Aminex HPX-87H column (300 mm × 7.88 mm, Bio-Rad Laboratories) was used in the HMF and furfural analysis on the aqueous and organic samples obtained after both extractions. The mobile phase (5 mM H2SO4 solution prepared in house using sulphuric acid provided from Sigma-Aldrich) was flowing at 0.6 mL min−1. Column was heated up to 65 °C. DAD signal set at 280 nm.
Lipid analysis
Lipid composition was determined using 1H nuclear magnetic resonance (NMR), detailed information is given in the ESI.†
Results and discussion
The biorefinery approach was designed to produce HMF from the C6 sugar fraction in an acid dehydration. By using a strong acid such as H2SO4, the cleaving of the biomass composite structure, the depolymerisation of polysaccharides into oligo and monosaccharides and the subsequent dehydration of these monomers into HMF is possible.39 This process was followed by an extraction of the produced HMF, where the solvent (dichloromethane
:
2-butanol (50
:
50 w/w %) was recycled. The extracted stillage from this extraction was then fed into a second acid dehydration to produce further HMF from the unused carbohydrates of the first reaction. The reactor effluent was subject to a second HMF extraction with the solvent being recycled. The resulting waste stream from the entire process was then submitted to hydrothermal liquefaction to produce biocrude and biochar (Fig. 1).
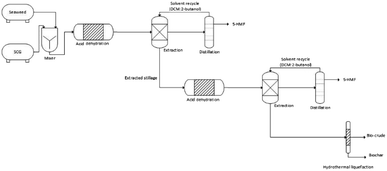 |
| Fig. 1 Proposed process configuration of biorefinery for HMF production. | |
SCG and U. lactuca have a carbohydrate content of approximately 47.8% and 45.6%, respectively. Most of the carbohydrates present in these feedstocks were C6 sugars (89% and 80% of the total carbohydrates, respectively – Table 1). Such results suggest that these feedstocks can have a high potential for conversion into HMF.23,40 On the other hand, C. filum has a 24.8% carbohydrate content, from which only 25% of the total carbohydrates were C6 sugars.
Table 1 Main monosaccharides content in the original feedstocks, data determined using the laboratory analytical procedure developed by Van Wychen and Laurens41
(%) |
SCG |
U. lactuca
|
C. filum
|
Glucose |
20.1 ± 0.6 |
34.8 ± 1.7 |
5.7 ± 0.3 |
Galactose/mannose |
22.6 ± 0.3 |
1.7 ± 0.2 |
0.5 ± 0.0 |
Arabinose |
5.2 ± 0.6 |
9.1 ± 0.7 |
18.6 ± 0.7 |
Acid dehydration for HMF production
The initial sulphuric acid treatment depolymerised a portion of the saccharide feedstock, and some HMF production was observed. The SCG produced the most with, 2.69 g L−1 produced, a yield of 2.8% from the saccharide portion. U. lactuca produced 1.76 g L−1 (1.9%) whereas C. filum produced 0.24 g L−1 (0.5%) Fig. 2a. Yields of 6.9% and 7.0% were achieved for UL0.6 + SCG0.4 and UL0.4 + SCG0.6, respectively, while 6.7% and 3.9% were obtained for the blends of SCG with C. filum (CF0.4 + SCG0.6 and CF0.6 + SCG0.4, respectively) (Table 2).
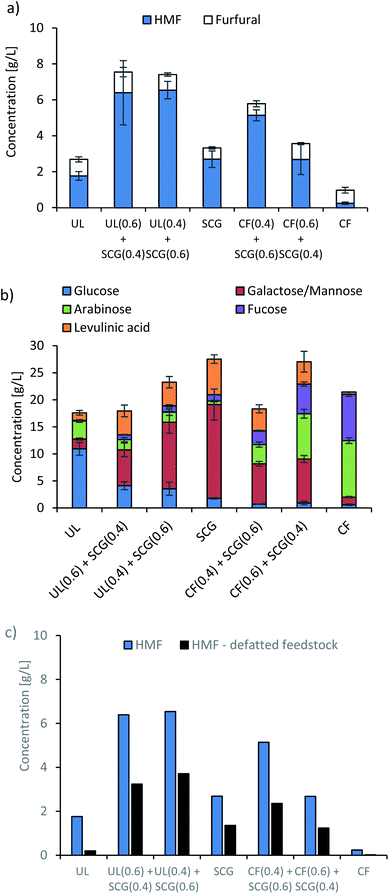 |
| Fig. 2 (a) HMF and furfural production in 1st dehydration; (b) monosaccharides concentration after 1st dehydration; (c) comparison of the HMF produced when using the raw feedstocks and when using a defatted feedstock. | |
Table 2 HMF recovery yields of the various feedstocks and blends. Values obtained considering the theoretical carbohydrate content from literature and the HMF concentration obtained on the first dehydration
|
UL |
UL0.6 + SCG0.4 |
UL0.4 + SCG0.6 |
SCG |
CF0.4 + SCG0.6 |
CF0.6 + SCG0.4 |
CF |
HMF yield (%) |
2.5 |
7.7 |
7.3 |
2.6 |
6.0 |
3.5 |
0.4 |
HMF recovery (%) |
86.7 |
80.5 |
83.1 |
83.1 |
82.3 |
90.4 |
78.3 |
Interestingly, the blends of Ulva and SCG produced far more HMF than either of the raw materials when processed separately. This was also observed for blends of SCG and C. filum. This is potentially due to the macroalgal species having more glucose, which is more readily released than in SCG, while the far higher content of lipids in SCG forms an organic layer.9,38,42,43 It has been previously observed, that a biphasic system can increase stability and yields by partitioning HMF as it is formed away from the aqueous phase.44 This is supported by a reduction of approximately 50% on the HMF produced from the blend when defatted SCG were used in the same series of experiments (Fig. 2c).
Apart from the C. filum system, all the other systems examined have low C5 sugar content, and accordingly lower furfural production. The reactor effluent was analysed for carbohydrates (Fig. 2b), and still showed a relatively high content in carbohydrates (approximately 14–23 g L−1 depending on feedstock). The analysis also showed that galactose/mannose is the monosaccharide most abundant in the SCG-rich feedstocks, while higher concentrations of glucose are observed in U. lactuca-rich feedstocks and arabinose and fucose in C. filum. Levulinic acid was also present in these slurries, mostly in the feedstocks containing SCG, suggesting that there are significant levels of HMF to dehydrate with the systems containing lipids, with far less observed for the two macroalgae species on their own. The presence of such high concentrations of monosaccharides in this stream indicated that these can be further processed in a second acid dehydration to produce more HMF.
Prior to the second dehydration the organic fraction, containing HMF, was removed. This was to prevent further dehydration of the HMF product while also allowing for more HMF to migrate to the organic layer formed by the lipids. The extraction system, a mixture of DCM and 2-butanol in a ratio of 1
:
1, showed relatively high recovery ratios for the HMF – approximately 80 to 90% of the HMF was recovered into the extraction solvent, while the rest remained in the aqueous fraction (Table 2) this is similar to the results reported by Tan et al.45
Subsequent acid dehydration to HMF
A second acid dehydration was undertaken on the combined solid and aqueous phase once the HMF had been removed. This led to higher production of HMF for the aqueous phase produced from the pure feedstocks of U. lactuca (6.1 g L−1) and SCG (5.1 g L−1), though the HMF from the C. filum (0.2 g L−1) and blends of this macroalgae with SCG were greatly reduced (Fig. 3a). This is presumably because there is a large pool of glucose and mannose that can be dehydrated in the SCG and U. lactuca and far less in the C. filum. In addition, the lack of lipids in C. filum does not allow for a large production of HMF, similarly to what happened in the first dehydration. However, the HMF produced in the blends of C. filum is considerably lower than what was achieved in the first dehydration. This might be due to the low amounts of C6 sugars left in solution after the first dehydration (b). When compared to the sugars present in solution after the second dehydration (b), sugars such as arabinose and fucose were barely consumed. The sugar analysis demonstrates that most of the glucose and mannose are consumed in the dehydration into HMF, while fucose and arabinose are somewhat more stable.
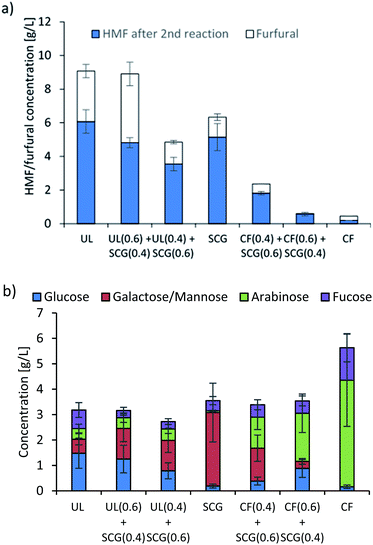 |
| Fig. 3 (a) HMF and furfural concentration in the slurry after the second acid dehydration; (b) sugar concentration in the slurry after the second acid dehydration. | |
Lipid analysis
The streams of HMF (both after first and second dehydrations) and the stream of stillage after the second dehydration were analysed for lipid content. Lipids were only found in the stream of stillage after the second dehydration.
This demonstrated that no lipids were extracted with the HMF, which confirms the lipids form an organic phase in both acid dehydrations, corroborating the results obtained by Wang et al. and Prates Pereira et al.23,40 This double layer presumably allows for the HMF to move into the organic phase, once produced in the aqueous phase. The presence of lipids in the stillage after the second dehydration would also potentially allow for a lipid extraction prior to the HTL reaction. The extraction of the lipids as a product in a separate stream could add further value to this biorefinery approach. The analysis on the lipids present in the stillage after the second dehydration show a high content of saturated fatty acids as well as linoleic and linolenic acids (Table 3).
Table 3 Type and content of fatty acids found in the lipids in the extracted stillage after the second acid dehydration. MUFA stands for mono-unsaturated fatty acids. Analysis and quantification of fatty acids in U. lactuca and C. filum was difficult due to the low content of fatty acids in these samples. However, in addition to the fatty acids shown below, C. filum revealed the presence of an unusual component, most probably stearidonic acid
|
Saturated |
Mono unsaturated |
Linoleic acid |
Linolenic acid |
UL |
— |
— |
— |
— |
UL0.6 + SCG0.4 |
58.60 |
4.10 |
30.92 |
6.38 |
UL0.4 + SCG0.6 |
54.35 |
16.40 |
27.27 |
1.98 |
SCG |
56.45 |
10.18 |
32.35 |
1.02 |
CF0.4 + SCG0.6 |
63.67 |
0.10 |
30.75 |
5.48 |
CF0.6 + SCG0.4 |
66.57 |
0.00 |
27.67 |
10.87 |
CF |
— |
— |
— |
— |
Hydrothermal liquefaction (HTL)
On the extraction of HMF, the resulting solid was converted into further products through hydrothermal liquefaction (Fig. 4). The U. lactuca, SCG and respective blends yielded relatively similar bio-crude and biochar yields, with a slight increase in biocrude with higher percentage of SCG in the blends. This is presumably due to the higher content of lipids in SCG, which is converted to biocrude in this process, as described by Madsen et al.46. These results demonstrate that blends of U. lactuca with SCG can be used in an effective HTL process without substantial reduction in main product yields (biocrude and biochar).
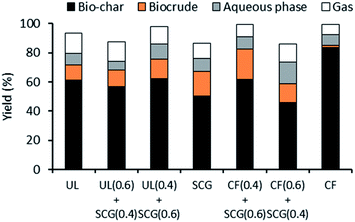 |
| Fig. 4 Mass balance of the extracted stillage used in HTL reaction. Gravimetric yields calculated based on the dry weight of the extracted stillage fed into the reaction. Gas phase calculated assuming a 100% content in CO2, while the aqueous gravimetric yield was calculated considering the solids in this phase. | |
The same trend was observed with SCG and C. filum, where a higher percentage of SCG in the blend associated with a higher biocrude yield. This is presumably due to the higher lipid content in SCG (converted into biocrude in HTL). On the other hand, the biochar yield increases with higher percentages of C. filum. This is presumably due to the breakdown of the carbohydrates not used in any of the dehydration processes and were broken down into biochar in the HTL. In contrast to U. lactuca, C. filum blends with SCG led to considerable differences in the biocrude and biochar yields. Therefore, if SCG were to be blended with C. filum in a biorefinery, the processes downstream to HTL would need to be prepared to handle different volumes of biocrude and biochar, depending on the season, thus on the percentages of the blends used.
Overall yields
The overall product yields were compared across the different blends and feedstocks (Table 4). The total HMF presented is the sum of the HMF extracted after the first and second dehydrations. Yields were extrapolated to 1 kg of dry biomass fed into the process. The biocrude and biochar amounts were calculated based on the amount of solids obtained after 2nd dehydration and on the HTL yields presented in Fig. 4. These were also extrapolated to 1 kg of dry biomass supplied to the entire system.
Table 4 Overall yield of the fuel products and precursors including total produced HMF, lipid production, biocrude and biochar from the proposed biorefinery, all values are given as kg per tonne and calculated on a dry ash free basis
|
Total HMF [g kgbiomass−1] |
Biocrude [g kgbiomass−1] |
Biochar [g kgbiomass−1] |
Mass all fuel products (%) |
Mass all fuel products (DAF) (%) |
UL |
31.2 |
83.5 |
489.8 |
60.5 |
72.0 |
UL0.6 + SCG0.4 |
46.6 |
78.2 |
390.2 |
51.5 |
57.3 |
UL0.4 + SCG0.6 |
35.3 |
67.6 |
314.2 |
41.7 |
45.0 |
SCG |
30.2 |
89.5 |
264.2 |
38.4 |
39.0 |
CF0.4 + SCG0.6 |
28.3 |
109.7 |
355.5 |
49.4 |
51.9 |
CF0.6 + SCG0.4 |
13.1 |
77.5 |
230.5 |
32.1 |
34.4 |
CF |
1.5 |
14.6 |
757.2 |
77.3 |
86.1 |
A higher amount of HMF was produced from UL0.6 + SCG0.4 (46.6 g kgbiomass−1) and UL0.4 + SCG0.6 (35.3 g kgbiomass−1). These are the blends intended to replace U. lactuca in periods of intermediate and lower supply of this seaweed. However, when processed separately, U. lactuca and SCG only produce 31.2 and 30.2 g kgbiomass−1, respectively, demonstrating that the blending of U. lactuca and SCG creates improved conditions for HMF production. A higher level of bio-crude was produced for the SCG (89.5 g kgbiomass−1) presumably due to the higher content of lipids in this feedstock, though a similar conversion was observed for all biomass blends. However, the higher the percentages of U. lactuca in the blends, the higher the production of biochar. This is mainly due to the higher content of ash in the macroalgae. Overall, both UL0.6 + SCG0.4 and UL0.4 + SCG0.6 demonstrate that SCG is a good replacement of U. lactuca in periods of lower seaweed supply.
Unlike the U. lactuca blends, when taking both extractions into account, the highest level of HMF was produced from the SCG and the lowest from the C. filum. The blends of SCG and C. filum were proportional to this. Such low production of HMF from this macroalgae is due to the low content of C6 sugars and lipids in this feedstock. This result has a high impact on the HTL results as the carbohydrates that were not dehydrated into HMF were then broken down into char, resulting in a high production of char of 757.2 g kgbiomass−1. In addition to this, the low content of lipids in this feedstock led to a lower biocrude production (14.6 g kgbiomass−1) when compared to SCG (89.5 g kgbiomass−1).
Conclusions and future perspectives
In this study a biorefinery producing 5-(hydroxymethyl)furfural (HMF), biocrude and biochar from macroalgae and spent coffee grounds blends was developed. U. lactuca was found to be a suitable species, containing elevated C6 sugars that could be converted into HMF. Interestingly, the addition of spent coffee grounds increased the production substantially, in comparison to the macroalgae or spent coffee grounds alone. This was presumably due to the formation of a lipid layer in the aqueous phase that reduced the decomposition of HMF to levulinic acid. The stillage from the reaction was also further converted into fuel products through HTL, yielding 46.6 g kg−1 HMF, 78.2 g kg−1 biocrude, 390 g kg−1 biochar in the optimised system. The same system with C. filum was less productive, presumably due to lower lipid and C6 sugars in the macroalgae. The aim of this work was achieved as it demonstrates that an integrated HMF biorefinery is possible using U. lactuca, and that the addition of spent coffee grounds not only would allow all year round production, but could also increase the yield of specific target products, such as HMF and HTL products. Indeed, blending with spent coffee grounds has potential to improve process versatility making biomasses considered unsuitable alone (such as Chorda filum) into viable feedstocks. Furthermore, the current work demonstrates that the production of HMF can be performed in a single-step reaction rather than in three-steps as widely presented in the literature. Further perspectives to be considered in future work include the optimisation of the HMF production in the dehydration process. A techno-economic analysis is also suggested to determine the profitability of the second acid dehydration process, possibly a third acid dehydration to use the unused sugars, and the inclusion of a lipid extraction process upstream to the hydrothermal liquefaction.
Author contributions
André Prates Pereira: conceptualization, investigation, writing – original draft. Timothy J. Woodman: investigation, data curation, formal analysis. Christopher J. Chuck: conceptualization, supervision, writing – review and editing.
Conflicts of interest
There are no conflicts to declare.
Acknowledgements
We extend our thanks to Michael, Rosie and Archie Allen for supplying the Ulva lactuca and Chorda filum feedstocks used in this work, and to the University of Bath URS scheme for funding.
Notes and references
- M. R. Tabassum, A. Xia and J. D. Murphy, Potential of seaweed as a feedstock for renewable gaseous fuel production in Ireland, Renewable Sustainable Energy Rev., 2017, 68, 136–146, DOI:10.1016/J.RSER.2016.09.111.
- E. S. Jones, S. Raikova, S. Ebrahim, S. Parsons, M. J. Allen and C. J. Chuck, Saltwater based fractionation and valorisation of macroalgae, J. Chem. Technol. Biotechnol., 2020, 95(8), 2098–2109, DOI:10.1002/jctb.6443.
- K. Balina, F. Romagnoli and D. Blumberga, Seaweed biorefinery concept for sustainable use of marine resources, Energy Procedia, 2017, 128, 504–511, DOI:10.1016/J.EGYPRO.2017.09.067.
-
The State of World Fisheries and Aquaculture 2012, FAO, Italy, 2012 Search PubMed.
-
The State of World Fisheries and Aquaculture 2018, FAO, Italy, 2018 Search PubMed.
-
E. Capuzzo and T. McKie, Seaweed in the UK and Abroad - Status, Products, Limitations, Gaps and Cefas Role, 2016 Search PubMed.
-
J. Murphy, B. Drosg, E. Allen, J. Jerney, A. Xia and C. Herrmann, A Perspective on Algal Biogas, 2016, DOI: DOI:10.13140/RG.2.1.4268.7127.
-
F. Bunker, Seaweeds of Britain and Ireland, Wild Nature Press, Plymouth, UK, 2nd edn, 2017 Search PubMed.
- T. A. Beacham, I. S. Cole and L. S. DeDross,
et al. Analysis of seaweeds from South West England as a biorefinery feedstock, Appl. Sci., 2019, 9, 4456 CrossRef CAS.
- S. Raikova, M. J. Allen and C. J. Chuck, Hydrothermal liquefaction of macroalgae for the production of renewable biofuels, Biofuels, Bioprod. Biorefin., 2019, 13(6), 1483–1504 CrossRef CAS.
- S. Raikova, C. D. Le, T. A. Beacham, R. W. Jenkins, M. J. Allen and C. J. Chuck, Towards a marine biorefinery through the hydrothermal liquefaction of macroalgae native to the United Kingdom, Biomass Bioenergy, 2017, 107, 244–253, DOI:10.1016/j.biombioe.2017.10.010.
- S. Raikova, T. D. J. Knowles, M. J. Allen and C. J. Chuck, Co-liquefaction of macroalgae with common marine plastic pollutants, ACS Sustainable Chem. Eng., 2019, 7(7), 6769–6781, DOI:10.1021/acssuschemeng.8b06031.
- M. Piccini, S. Raikova, M. J. Allen and C. J. Chuck, A synergistic use of microalgae and macroalgae for heavy metal bioremediation and bioenergy production through hydrothermal liquefaction, Sustainable Energy Fuels, 2019, 3(1), 292–301 RSC.
- P. Biller and A. B. Ross, Potential yields and properties of oil from the hydrothermal liquefaction of microalgae with different biochemical con tent, Bioresour. Technol., 2011, 102(1), 215–225, DOI:10.1016/j.biortech.2010.06.028.
- W. Yang, X. Li, Z. Li, C. Tong and L. Feng, Understanding low-lipid algae hydrothermal liquefaction characteristics and pathways through hydrothermal liquefaction of algal major components: Crude polysaccharides , crude proteins and their binary mixtures, Bioresour. Technol., 2015, 196, 99–108, DOI:10.1016/j.biortech.2015.07.020.
-
G. Yu, Y. Zhang, L. Schideman, T. L. Funk and Z. Wang, Hydrothermal Liquefaction of Low Lipid Content Microalgae into Bio-Crude Oil, 2011 Search PubMed.
- M. Ghadiryanfar, K. A. Rosentrater, A. Keyhani and M. Omid, A review of macroalgae production, with potential applications in biofuels and bioenergy, Renewable Sustainable Energy Rev., 2016, 54, 473–481, DOI:10.1016/j.rser.2015.10.022.
- F. Abeln, J. Fan and V. L. Budarin,
et al., Lipid production through the single-step microwave hydrolysis of macroalgae using the oleaginous yeast Metschnikowia pulcherrima, Algal Res., 2019, 38, 101411 CrossRef.
- H. Zhao, J. E. Holladay, H. Brown and Z. C. Zhang, Metal Chlorides in Ionic Liquid Solvents Convert Sugars to 5-Hydroxymethylfurfural, Science, 2007, 316(5831), 1597–1600, DOI:10.1126/science.1141199.
-
National Science Foundation, Breaking the Chemical and Engineering Barriers to Lignocellulosic Biofuels: Next Generation Hydrocarbon Biorefineries, 2008 Search PubMed.
- M. Bicker, J. Hirth and H. Vogel, Dehydration of fructose to 5-hydroxymethylfurfural in sub- and supercritical acetone, Green Chem., 2003, 5(2), 280–284, 10.1039/B211468B.
- A. H. Motagamwala, K. Huang, C. T. Maravelias and J. A. Dumesic, Solvent system for effective near-term production of hydroxymethylfurfural (HMF) with potential for long-term process improvement, Energy Environ. Sci., 2019, 12(7), 2212–2222 RSC.
- A. Prates Pereira, T. J. Woodman, P. Brahmbhatt and C. J. Chuck, The optimised production of 5-(hydroxymethyl)furfural and related products from spent coffee grounds, Appl. Sci., 2019, 9, 3369 CrossRef.
- Y. Roman-Leshkov, C. J. Barrett, Z. Y. Liu and J. A. Dumesic, Production of Dimethylfuran for Liquid Fuels from Biomass-Derived Carbohydrates, Nature, 2007, 447, 982–985, DOI:10.1038/nature05923.
- F. A. Kucherov, E. G. Gordeev, A. S. Kashin and V. P. Ananikov, Three-Dimensional Printing with Biomass-Derived PEF for Carbon-Neutral Manufacturing, Angew. Chem., Int. Ed., 2017, 56(50), 15931–15935, DOI:10.1002/anie.201708528.
- B. Kim, J. Jeong and S. Shin,
et al., Facile Single-Step Conversion of Macroalgal Polymeric Carbohydrates into Biofuels, ChemSusChem, 2010, 3(11), 1273–1275, DOI:10.1002/cssc.201000192.
- S.-B. Lee, S.-K. Kim, Y.-K. Hong and G.-T. Jeong, Optimization of the production of platform chemicals and sugars from the red macroalga, Kappaphycus alvarezii, Algal Res., 2016, 13, 303–310, DOI:10.1016/j.algal.2015.12.013.
- G.-T. Jeong and D.-H. Park, Production of Sugars and Levulinic Acid from Marine Biomass Gelidium amansii, Appl. Biochem. Biotechnol., 2010, 161(1), 41–52, DOI:10.1007/s12010-009-8795-5.
- F. Kholiya, M. R. Rathod, D. R. Gangapur, S. Adimurthy and R. Meena, An integrated effluent free process for the production of 5-hydroxymethyl furfural (HMF), levulinic acid (LA) and KNS-ML from aqueous seaweed extract, Carbohydr. Res., 2020, 490, 107953, DOI:10.1016/j.carres.2020.107953.
- R. R. Gonzales, Y. Hong, J. H. Park, G. Kumar and S. H. Kim, Kinetics and equilibria of U_hydroxymethylfurfural (U_HMF) sequestration from algal hydrolyzate using granular activated carbon, J. Chem. Technol. Biotechnol., 2016, 91, 1157–1163 CrossRef.
- P. Malea, A. Chatziapostolou and T. Kevrekidis, Trace element seasonality in marine macroalgae of different functional-form groups, Mar. Environ. Res., 2015, 103, 18–26, DOI:10.1016/j.marenvres.2014.11.004.
- C. Lefèvre and D. Bellwood, Seasonality and dynamics in coral reef macroalgae: Variation in condition and susceptibility to herbivory, Mar. Biol., 2010, 157, 955–965, DOI:10.1007/s00227-009-1376-x.
- C. J. Fulton, M. Depczynski and T. H. Holmes,
et al. Sea temperature shapes seasonal fluctuations in seaweed biomass within the Ningaloo coral reef ecosystem, Limnol. Oceanogr., 2014, 59(1), 156–166, DOI:10.4319/lo.2014.59.1.0156.
- R. Ferrari, M. Gonzalez-Rivero, J. C. Ortiz and P. J. Mumby, Interaction of herbivory and seasonality on the dynamics of Caribbean macroalgae, Coral Reefs, 2012, 31(3), 683–692, DOI:10.1007/s00338-012-0889-9.
- B. Jin, P. Duan, Y. Xu, F. Wang and Y. Fan, Co-liquefaction of micro- and macroalgae in subcritical water, Bioresour. Technol., 2013, 149, 103–110, DOI:10.1016/j.biortech.2013.09.045.
- A. Prates Pereira, T. Dong, E. P. Knoshaug, N. Nagle, R. Spiller, B. Panczak, C. J. Chuck and P. T. Pienkos, An alternative biorefinery approach to address microalgal seasonality: blending with spent coffee grounds, Sustainable Energy Fuels, 2020, 4(7), 3400–3408 RSC.
-
Coffee: World markets and trade, 2019/20 Forecast Overview, United States Department of Agriculture, Foreign Agricultural Service, December 2019 Search PubMed.
- J. Massaya, A. Prates-Pereira, B. Mills-Lamptey, J. Benjamin and C. J. Chuck, Conceptualization of a spent coffee grounds biorefinery: a review of existing valorisation approaches, Food Bioprod. Process., 2019, 118, 149–166 CrossRef.
- F. Menegazzo, E. Ghedini and M. Signoretto, 5-Hydroxymethylfurfural (HMF) Production from Real Biomasses, Molecules, 2018, 23(9), 2201, DOI:10.3390/molecules23092201.
- W. Wang, A. Mittal, H. Pilath, X. Chen, M. P. Tucker and D. K. Johnson, Simultaneous upgrading of biomass-derived sugars to HMF/furfural via enzymatically isomerized ketose intermediates, Biotechnol. Biofuels, 2019, 12(1), 253, DOI:10.1186/s13068-019-1595-4.
-
S. Van Wychen and L. M. L. Laurens, Determination of Total Carbohydrates in Algal Biomass: Laboratory Analytical Procedure (LAP), 2016 Search PubMed.
- H. Yaich, H. Garna, S. Besbes, M. Paquot, C. Blecker and H. Attia, Chemical composition and functional properties of Ulva lactuca seaweed collected in Tunisia, Food Chem., 2011, 128(4), 895–901, DOI:10.1016/j.foodchem.2011.03.114.
- S. V. Khotimchenko, Fatty acids of brown algae from the Russian far east, Phytochemistry, 1998, 49(8), 2363–2369, DOI:10.1016/S0031-9422(98)00240-4.
- J. M. R. Gallo, D. M. Alonso, M. A. Mellmer and J. A. Dumesic, Production and upgrading of 5-hydroxymethylfurfural using heterogeneous catalysts and biomass-derived solvents, Green Chem., 2013, 15(1), 85–90, 10.1039/C2GC36536G.
- F. Liu, S. Sivoththaman and Z. Tan, Solvent extraction of 5-HMF from simulated hydrothermal conversion product, Sustainable Environ. Res., 2014, 24(2), 149–157 Search PubMed.
- R. B. Madsen, H. Zhang, P. Biller, A. H. Goldstein and M. Glasius, Characterizing Semivolatile Organic Compounds of Biocrude from Hydrothermal Liquefaction of Biomass, Energy Fuels, 2017, 31(4), 4122–4134, DOI:10.1021/acs.energyfuels.7b00160.
Footnote |
† Electronic supplementary information (ESI) available. See DOI: 10.1039/d1se01142a |
|
This journal is © The Royal Society of Chemistry 2021 |
Click here to see how this site uses Cookies. View our privacy policy here.