DOI:
10.1039/D1SC05112A
(Edge Article)
Chem. Sci., 2021,
12, 15533-15542
Multi-stimuli programmable FRET† based RGB absorbing antennae towards ratiometric temperature, pH and multiple metal ion sensing‡
Received
15th September 2021
, Accepted 15th November 2021
First published on 15th November 2021
Abstract
A red-green-blue (RGB) multichromophoric antenna 1 consisting of energy donors naphthalimides and perylenediimides and a central aza-BODIPY energy acceptor along with two subchromophoric red-blue (RB 6) and green-blue (GB 12) antennae was designed that showed efficient cascade Förster resonance energy transfer (FRET). RGB antenna 1 showed pronounced temperature-dependent emission behaviour where emission intensities in green and red channels could be tuned in opposite directions by temperature giving rise to unique ratiometric sensing with a temperature sensitivity of 0.4% °C. RGB antenna 1 showed reversible absorption modulation selectively in the blue region (RGB ↔ RG) upon acid/base addition giving rise to pH sensing behaviour. Furthermore, RGB antenna 1 was utilized to selectively sense metal ions such as Co2+ and Fe3+ through a FRET turn-off mechanism induced by a redox process at the aza-BODIPY site that resulted in the selective spectral modulation of the red band (i.e., RGB → GB). Model antenna RB 6 showed white light emission with chromaticity coordinates (0.32, 0.33) on acid addition. Antennae 1, 6 and 12 also exhibited solution state electrochromic switching characterized by distinct colour changes upon changing the potential. Finally, antennae 1, 6 and 12 served as reversible fluorescent inks in PMMA/antenna blends whereby the emission colours could be switched or tuned using different stimuli such as acid vapour, temperature and metal ions.
Introduction
Photosynthesis is initiated by capturing of sunlight by light harvesting (LH) antennae followed by rapid excitation energy transfer and has been an area of intense activity since decades.1–5 Natural photosynthetic systems contain LH antennae that absorb in the complementary regions of the solar spectrum ultimately leading to panchromatic absorption.6,7 Efficient harvesting of sunlight can be achieved by using biomimetic architectures,8 with intense and panchromatic absorption in the red-green-blue (RGB) region. Such architectures can be constructed by carefully choosing chromophores absorbing in these three regions of the solar spectrum that show ultrafast intrachromophoric energy transfer and are photochemically robust.8 Multiple reports of artificial LH arrays constructed either through covalent bonds2,9 such as dendrimers,10–13 conjugated polymers,14 multichromophoric arrays8,9,15,16 or non-covalent achitectures8,17–20 are available. However, the majority of the reported covalent multichromophoric LH systems are based on the one step Förster resonance energy transfer (FRET) process between the energy donor and energy acceptor.21 Covalently connected LH systems based on two step energy transfer, panchromatic absorption and large Stokes shift are suitable for multiple sensing and imaging applications.22 Reports on two step cascade energy transfer based multichromophoric RGB LH systems are achieved mostly through dendritic design23–27 such as dendritic solar concentrators,28 arrays,13,29–31 small molecule based funnels,9,32,33 heterotriads34 and templated chromophore assemblies on peptide scaffolds,35 DNA scaffolds36 and organic nanostructures.37 FRET based LH systems have also been utilized in organic photovoltaics,38 photocatalysis,39 photodynamic therapy,40 bioimaging,41 ratiometric temperature sensing and ion sensing21,42–44 in recent years.
Materials which show modulation of physiochemical properties with external stimuli such as pH, heat, chemicals, electricity have been under extensive research in the last few years.45–47 Owing to multiple absorption and emission bands of RGB systems, it is possible to modulate their spectral properties by selectively tuning the bands by some kind of external stimuli such as temperature, pH and so on. RGB systems incorporated into polymers have been successfully utilized for modulating and switching their two- and three-state absorption or emission using external stimuli such as pH, temperature and so on.45,46,48–52 However, to the best of our knowledge, covalently connected small molecule based RGB antennae that exhibit FRET and serve as multiparameter sensors through the selective modulation of the different absorption or emission bands by external stimuli, have not been reported. Covalently connected multichromophoric RGB antenna systems are synthetically much more challenging to achieve compared to supramolecular or polymeric designs. However, the former provides several advantages such as high purity, monodispersity, synthetic scalability, solubility and facile tunability of structural and optical properties over supramolecular or polymeric systems. Herein, we report a RGB LH antenna 1 (Fig. 1) consisting of eleven chromophores that are covalently connected with eight 1,8-naphthalimides (Np)53 (blue absorber) as primary donors, two perylenediimides (PDI)54 (green) as primary acceptors and one aza-BODIPY55 (red) as the secondary acceptor for cascade FRET. 1,8-Np was selected as the blue (B) light absorbing chromophore due to its high fluorescence quantum yield and excellent photothermal and chemical stability.53 PDI being one of the most versatile and well-studied chromophores owing to its near-unity fluorescence quantum yield, outstanding (photo)chemical and thermal stability and intense absorption in the visible region was utilized as the green (G) chromophore.54 Aza-BODIPY with intense absorption in the near-infrared (NIR) region, high fluorescence quantum yield, good photo and thermal stability and wide applications as optical sensors and optoelectronic materials55 was employed as the red (R) chromophore. Np connected to the PDIs through bay positions via the non-conjugated phenoxy linker and further PDI-(Np)4 connected to aza-BODIPY through triazole rings via the click reaction ensure the preclusion of any electronic communication between donor and acceptor chromophores. The RGB antenna 1 showed ∼100% FRET to either PDI or to aza-BODIPY upon photoexcitation of Np. Subchromophoric RB antenna 6 and GB antenna 12 were also synthesized and studied in detail (Fig. 1). In addition to efficient cascade FRET, the designed antennae RGB 1, RB 6 and GB 12 showed selective modulation of their various absorption and emission bands by application of external stimuli such as pH, metal ions, and temperature and redox stimuli. Such prominent multi stimuli responsive absorption and emission modulation leading to pH sensing, ratiometric temperature sensing, multiple metal ion sensing by FRET turn-off and electrochromic switching can lead to pronounced multiparameter sensing in cellular microenvironments using these molecular antennae.
 |
| Fig. 1 Chemical structures of RGB antenna 1, RB 6 and GB 12, and Np 9 and schematic representation of the RGB antenna for multiparameter sensing. | |
Results and discussion
Synthesis
The detailed synthetic routes and experimental procedures for RGB antenna 1 and the model antennae RB 6 and GB 12 are described in Scheme S1–S4 in the ESI.‡ The model antenna RB 6 was synthesized by the copper(I) catalysed azide–alkyne cycloaddition (CuAAC) reaction between Np 4 and aza-BODIPY 5 in 78% yield (Scheme 1) and model antenna GB 12 was synthesized by nucleophilic substitution of PDI compound 11 at its bay positions with Np 9 in 33% yield (Scheme S3‡). Subsequently, GB 12 was converted from the alcoholic to azide functionality for further reaction with aza-BODIPY 5 by the CuAAC reaction to obtain the final RGB antenna 1 in 82% yield as a brown colour solid (Scheme 2). All the intermediates and final compounds were characterized structurally using 1H NMR, 13C NMR and HRMS/MALDI techniques.
 |
| Scheme 1 Synthesis of RB antenna 6. | |
 |
| Scheme 2 Synthesis of RGB antenna 1. | |
Photophysical properties
The photophysical properties of RGB antenna 1, RB 6 and GB 12 were investigated using UV/Vis absorption, steady state fluorescence, fluorescence quantum yield and fluorescence lifetime measurements. The absorption and emission properties of RGB antenna 1, RB 6 and GB 12 were investigated in chloroform (CHCl3) (Fig. 2a and b) and summarized in Table 1. RB 6 exhibited two absorption bands at 442 nm and 688 nm corresponding to Np and aza-BODIPY respectively and GB 12 showed two absorption bands at 436 nm and 564 nm corresponding to Np and PDI respectively. RGB antenna 1 exhibited panchromatic absorption with three well-separated absorption bands at 436 nm, 566 nm and 693 nm corresponding to Np, PDI and aza-BODIPY respectively which further facilitate their selective photoexcitation and it has a brown colour indicating the spectral sum of blue, green and red colours. Constituent chromophores retaining their spectral identity in the final RGB antenna indicate that there is no ground state electronic interaction between them.
 |
| Fig. 2 (a) UV/Vis spectra, (b) emission spectra of Np 9, aza-BODIPY 5, RB 6, GB 12 and RGB 1 in CHCl3 (c ∼ 2 × 10−6 M); (c) fluorescence excitation spectrum of RGB antenna 1 at λem = 721 nm of aza-BODIPY; (d) spectral overlap of emission spectra of energy donors and absorption spectra of energy acceptors for the constituent chromophores of RGB antenna 1. | |
Table 1 Photophysical parameters of Np 9, aza-BODIPY 5, RB 6, GB 12 and RGB 1 in CHCl3
Comp. |
λ
abs (nm) |
ε(M−1 cm−1) |
λ
em (nm) |
9
|
430 |
12 360 |
518 |
5
|
682 |
86 747 |
714 |
6
|
442, 688 |
40 683 (442), 92 619 (688) |
518, 717 (λex = 442 nm), 717 (λex = 688 nm) |
12
|
436, 564 |
66 886 (436), 36 614 (564) |
518, 606 (λex = 436 nm), 606 (λex = 564 nm) |
1
|
436, 566, 693 |
166 285 (436), 87 694 (566), 95 549 (693) |
606, 721 (λex = 436 nm), 606, 721 (λex = 566 nm), 723 (λex = 693 nm) |
The primary energy donor Np 9 showed high emission intensity upon excitation at 430 nm; however, for model antennae RB 6, GB 12 and RGB antenna 1, the emission intensity of Np was quenched by ∼99% and simultaneously we observed emission from PDI and aza-BODIPY moieties which is indicative of FRET from Np to PDI or to aza-BODIPY (Fig. 2b). Further in RGB antenna 1 on direct excitation of primary energy acceptor PDI the emission intensity was quenched by ∼90% compared to model antenna GB 12 indicative of FRET from PDI to aza-BODIPY (Fig. 2b). Fluorescence excitation spectra were recorded for RB 6, GB 12 (Fig. S1‡) and RGB antenna 1 (Fig. 2c) in chloroform by holding emission monochromator at the λem of corresponding energy acceptors. For RGB antenna 1, the excitation spectra (Fig. 2c) consisted of the bands of all the constituent chromophores Np, PDI and aza-BODIPY, recorded at an emission wavelength of 721 nm (aza-BODIPY emission), clearly revealing efficient FRET from Np and PDI to aza-BODIPY. Fig. 2d represents the efficient spectral overlap of emission spectra of the primary donor (Np) and absorption spectra of the primary energy acceptor (PDI), and emission spectra of the secondary donor (PDI) and absorption spectra of the secondary acceptor (aza-BODIPY) which is an essential pre-requisite for efficient FRET.
Further, from the reduction of fluorescence quantum yields (Table S1‡) of the reference energy donors, the energy transfer efficiencies (ETEs) (Table S2‡) were calculated to be ∼100% for model antennae RB 6, GB 12 and RGB antenna 1.
To further confirm the energy transfer process in RB 6, GB 12 and RGB 1, time resolved fluorescence measurements were performed by the time correlated single photon counting (TCSPC) technique in CHCl3 and fluorescence lifetimes are summarised in Table S3.‡ The reference energy donor Np 9 on excitation at 440 nm exhibited a lifetime of 7.93 ns while in RB 6, GB 12 and RGB 1 on excitation at 440 nm (Np excitation), lifetime was reduced to 0.027 ns (99%), 0.006 ns (100%) and 0.008 ns (100%) which could be attributed to energy transfer from donor(s) to the acceptor in all the antennae. Furthermore, RGB 1 on excitation at 560 nm (PDI excitation) exhibited one shorter decay component of 3.59 ns (contribution of 29%) which is due to energy transfer from PDI to aza-BODIPY and a longer decay component of 5.88 ns (65%) due to PDI emission. For RB 6, while monitoring the acceptor emission, lifetime was higher upon donor (Np) excitation (3.08 ns, 100%) compared to direct acceptor (aza-BODIPY) excitation (2.83 ns, 100%) which is indicative of FRET from Np to aza-BODIPY. Even though the spectral overlap of Np emission and aza-BODIPY absorption is minimal, FRET occurs in the present system as is reported for several orthogonally arranged chromophores or chromophores with minimal spectral overlap.56–59 For GB 12, fluorescence lifetime on direct acceptor excitation (5.95 ns, 100%) was almost comparable to donor (Np) excitation (5.69 ns with 86% contribution). For RGB 1, while monitoring the acceptor emission channel, fluorescence lifetimes for direct acceptor (aza-BODIPY) excitation (2.51 ns), donor (Np) excitation (2.27 ns) and donor (PDI) excitation (2.57 ns) were comparable. The ETEs calculated from fluorescence lifetimes for all three antennae are shown in Table S4.‡
Solvent polarity dependent absorption and emission spectra were recorded for antennae RB 6, GB 12 and RGB antenna 1 (Table S5‡) and ETEs were calculated to be ∼90–100% (Table S6‡) by comparing the emission intensities with reference energy donors (Fig. S2–S4‡) which is indicative of the fact that FRET is the major deactivation pathway in all these antennae (explained in detail in the ESI‡).
Ratiometric temperature sensing
Owing to their multiple channel emissions, ratiometric temperature sensing behaviour was elucidated by monitoring the emission in all the channels of these compounds at different temperatures. Such ratiometric sensing has several advantages such as internal self-calibration and inertness to instrumental parameters or light intensity and sample concentration and thus are highly advantageous over single emission intensity-based temperature sensors.43 Temperature-dependent fluorescence spectra were recorded for RB 6, GB 12 and RGB antenna 1 in CHCl3 in the temperature range −10 °C to +60 °C.
For RB 6, an increase in temperature led to a blue shift and a decrease in the emission intensity of the aza-BODIPY band at 717 nm which could be attributed to thermal quenching while emission of Np at 518 nm remained unchanged (Fig. 3a). From the plot of I518/I518 + I717vs. temperature, temperature sensitivity was calculated to be 0.1% °C (Fig. 3b). For GB 12, an increase in temperature led to an increase in the intensity of the PDI band at 606 nm which could be due to an increase in FRET efficiency from Np to PDI with a rise in temperature from Np to PDI (Fig. 3c) and varying the temperature sensitivity from 0.015% °C to 0.001% °C (Fig. 3d and S5‡). For RGB antenna 1, increase in temperature led to an increase in the emission intensity of the PDI band (606 nm) and a concomitant decrease in the intensity of the aza-BODIPY band (721 nm) which indicates FRET modulation i.e., decreased FRET between PDI and aza-BODIPY upon increasing the temperature (Fig. 3e). The FRET modulation could be attributed to temperature induced conformational changes that might change the transition dipole orientations and thus the extent of FRET.44,60 Temperature dependent UV/Vis spectra were mostly unchanged for all compounds (Fig. S6‡).
 |
| Fig. 3 Temperature-dependent emission spectra of (a) RB 6, (c) GB 12 and (e) RGB 1 in CHCl3 (c ∼ 2 × 10−6 M); plot of (b) I518/I518 + 717vs. temperature (RB 6), (d) I518/I518 + 606vs. temperature (GB 12) and (f) I606/I606 + 721vs. temperature (RGB 1). Insets of (c) and (e) show the solution colours at two different temperatures under 365 nm UV lamp illumination. | |
Even though the two bands changed in opposite directions upon increasing the temperature for 1, their ratio I606/I606 + I721 showed a linear dependence with temperature and a temperature sensitivity of 0.4% °C (Fig. 3f). Such ratiometric temperature sensing behaviour with two bands changing in opposite directions has been rarely reported for organic chromophoric systems.61
Electrochemical properties and electrochromism
Redox properties of RGB antenna 1, RB 6 and GB 12 were assessed by cyclic voltammetry measurements (Fig. S7‡) and the frontier molecular orbital energy levels (HOMO and LUMO) were calculated for all molecules (Fig. S8‡ and Table 2). The HOMO levels of RGB antenna 1, RB 6 and GB 12 were obtained as −5.75 eV, −5.76 eV and −5.73 eV. The deep lying HOMO levels of the antenna 1, RB 6 and GB 12 indicate their resistance to aerial oxidation and high ambient stability. Furthermore, LUMO levels of −4.33 eV, −4.53 eV and −4.48 eV were obtained for RGB antenna 1, RB 6 and GB 12 respectively indicative of strong electron deficient behaviour of these compounds, desired pre-requisites for (opto)electronic applications.
Table 2 Redox properties of compounds 9, RB 6, GB 12 and RGB 1
Property |
9
|
6
|
12
|
1
|
HOMO = −(Eonsetox + 4.76) eV, where Eonsetox is first oxidation onset.
LUMO = −(Eonsetred + 4.76) eV, where Eonsetred is first reduction onset.
E
optg = 1241/λonset.
|
E
onsetox (V) |
+1.14 |
+1.00 |
+0.97 |
+0.99 |
E
onsetred (V) |
−1.35 |
−0.23 |
−0.28 |
−0.43 |
λ
onset (nm) |
490 |
735 |
611 |
742 |
HOMOa (eV) |
−5.90 |
−5.76 |
−5.73 |
−5.75 |
LUMOb (eV) |
−3.41 |
−4.53 |
−4.48 |
−4.33 |
E
optg
|
2.53 |
1.69 |
2.03 |
1.67 |
E
cvg (eV) |
2.49 |
1.23 |
1.25 |
1.42 |
In order to elucidate the effect of redox stimuli on spectral properties, spectroelectrochemistry was performed for reference chromophore Np 9 (Fig. S9‡), model antennae RB 6 and GB 12 and final RGB antenna 1. RB antenna 6 during oxidation showed depletion of the absorption band at 440 nm characteristic of Np+˙ formation (Fig. 4a), depletion of the 688 nm band and new band emergence at ∼760 nm corresponding to aza-BODIPY+˙ formation. In the reduction cycle, the depletion of the absorption band at 441 nm indicated the formation of Np−˙ and 688 nm band depletion and new band formation at 831 nm indicated the formation aza-BODIPY−˙ (Fig. 4b). GB antenna 12 showed the Np band depletion at 438 nm corresponding to the formation of Np+˙ and Np−˙ while the PDI band at 564 nm remained unaffected during the oxidation cycle (Fig. 4c). In the reduction cycle, the Np band at 438 nm depleted and the PDI band at 564 nm depleted with concomitant emergence and disappearance of two new bands at 673 nm and 774 nm indicating the formation of PDI−˙ and PDI2−˙ (Fig. 4d).
 |
| Fig. 4 Spectroelectrochemical changes observed for (a), (b) RB 6, (c), (d) GB 12 and (e), (f) RGB antenna 1 in DCM during the oxidation (0 to +2 V) and reduction process (0 to −2 V) respectively. Initial and final spectra are represented by blue and red colours respectively. | |
RGB antenna 1 showed the same effect as that of subchromophoric parts, showing depletion of the Np band at 438 nm during both oxidation and reduction corresponding to the formation of Np+˙ and Np−˙ respectively and the PDI band at 566 nm remained unaffected during the oxidation cycle. In the reduction cycle, the PDI band was depleted and new bands emerged and disappeared at ∼688 nm and at 772 nm corresponding to the formation of PDI−˙ and PDI2−˙. Aza-BODIPY+˙ was characterized by depletion at 692 nm and formation of a shoulder at 760 nm during the oxidation cycle while the reduction cycle showed the depletion of the band at 692 nm and emergence of a new band at 831 nm for aza-BODIPY−˙ formation (Fig. 4e and f). The corresponding changes in colour while applying the redox stimuli on RB 6, GB 12 and RGB 1 are depicted in Fig. 5. RB 6 which was initially light green in the neutral state turned to olive green either on oxidation or on reduction and reverted back to the initial colour in back cycles (Fig. 5). GB 12 was dark orange in the neutral state and it turned pink upon oxidation and bright fluorescent neon-greenish upon reduction and reverted back to the original colour of the neutral state upon reversion of the voltage applied (Fig. 5). RGB 1 was brown in the neutral state that reversibly turned to dark purple upon oxidation and to fluorescent neon-green in the reduction cycle (Fig. 5). Thus, all these compounds serve as reversible electrochromic switches in the solution phase and have potential applications as switchable redox sensors.
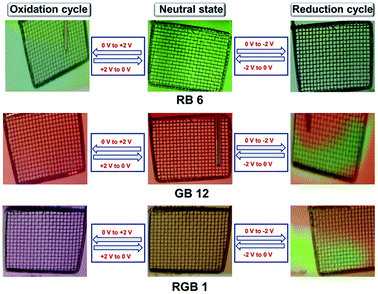 |
| Fig. 5 Images of solution-phase electrochromism behaviour of RB 6, GB 12 and RGB antenna 1 during (a) oxidation and (b) reduction processes during spectroelectrochemistry. | |
Furthermore, electrochemical experiments were performed on thin films of GB 12 and RGB antenna 1 spin coated onto indium tin oxide (ITO) plates using CHCl3 solutions (10−2 M) and a three electrode set-up with ITO as the working electrode, a Pt wire as the counter electrode and Ag/AgCl as the reference electrode and after experiment their absorption and transmittance spectra were recorded.47,62–65 Acetonitrile being an orthogonal solvent was used for performing the CV with addition of tetrabutylammonium hexafluorophosphate (TBAHFP) supporting electrolyte. GB antenna 12 was red in the neutral state and with application of positive potential from 0 to +2 V, the red colour disappeared and changed to colourless while with negative potential from 0 to −2 V, first the red colour changed to green and finally it became colourless (Fig. S10a‡). Similarly, RGB antenna 1 was brown in the neutral state, and with application of positive potential from 0 to +2 V, it changed to colourless and upon applying negative potential from 0 to −2 V, the brown colour changed first to a dark green colour which finally changed to transparent (Fig. S10b‡). The corresponding absorption (Fig. S11‡) and transmittance spectra (Fig. S12‡) for neutral thin films and for oxidized and reduced thin films for GB 12 and RGB 1 are provided in the ESI.‡ This experiment could not be performed with RB 6 because of its solubility in acetonitrile.
Acid/base sensing
The responsiveness of optical properties of RGB antenna 1, RB 6 and GB 12 to acids was investigated by addition of trifluoroacetic acid (TFA) and monitoring their absorption and emission profiles (Fig. 6 and S13–S22‡). Upon increasing the TFA amount from 0 to 74
667 equivalents (equiv.) in a CHCl3 solution of 1, the absorption band of Np at 436 nm showed a drastic decrease53 and a red shift to 447 nm (Fig. 6a). The absorbance of the PDI band showed a negligible red shift of ∼10 nm and absorbance of aza-BODIPY showed a slight blue shift of ∼8 nm and the RGB antenna was thus converted into a predominantly RG antenna (Fig. 6a) and corresponding emission spectra are shown in Fig. 6b. Upon back titrating the solution of antenna 1 with triethylamine (TEA), the blue absorption was recovered completely and thus the RG antenna was reversibly converted to RGB antenna 1 (Fig. S19a‡). The titration of RB 6 with increasing amounts of acid led to depletion of the absorption band at 436 nm (Fig. 6c). For RB antenna 6 with addition of acid, the fluorescence intensities of both PDI and aza-BODIPY decreased upon excitation at 396 nm. The corresponding emission spectra are shown in Fig. 6d. Interestingly, upon excitation at 396 nm, the emission colours showed a transition from green to blue through a single component white light emission with CIE coordinates of (0.32, 0.33) (Fig. 6e) at a particular equiv. of TFA (∼22
003 equiv.). The same solution however when excited at 442 nm, showed emission colours transitioning from green (0.34, 0.56) to yellow (0.40, 0.44) (Fig. 6f). Thus, reversible optical switching through acids and bases could be achieved for RGB 1 (i.e., RGB ↔ RG).
 |
| Fig. 6 Absorption and emission (λex = 396 nm) spectra on titration with TFA (a) and (b) of RGB 1; (c) and (d) of RB 6; chromaticity plot (e) (λex = 396 nm) and (f) (λex = 442 nm) for 6 in CHCl3 (c ∼ 5 μM). Initial and final spectra are represented by blue and red colours respectively. | |
On the addition of acid, the depletion in the Np absorption band can be attributed to the protonation of the amine nitrogen atom which led to conjugation break and hence the loss of colour. For the PDI band, with addition of acid the red shift and loss of intensity are reported in the literature66 where firstly, TFA molecules form H-bonding with imide –C
O units and then with bay position connected –O– atoms of PDI which led to a decrease in the energy gap between the HOMO and LUMO and resulted in a red shift. The loss of fluorescence intensity could be attributed to the electron transfer process between bay position H-bonded TFA molecules and the central PDI moiety.66 For aza-BODIPY, with the addition of TFA, a blue shift occurs presumably due to the H-bonding between TFA molecules and –O– atoms (Fig. 7).
 |
| Fig. 7 Basic representation of protonation/H-bonding sites between TFA molecules and respective Np, PDI and aza-BODIPY moieties. | |
In addition to acid/base sensitivity of these compounds in organic solvents, their pH sensing capabilities were also checked in aqueous solvents, similar to literature known example.67 Thus, pH titration experiments were performed in semi-aqueous solvent combinations (tetrahydrofuran (THF)/water 25/75 v/v) for antennae RB 6, GB 12 and RGB 1 where aqueous buffer was used. The UV/Vis titration at different pH and plots of absorbance vs. pH are shown in Fig. S23 in ESI.‡ Upon decrease in pH, these antennae showed most pronounced depletion of the Np absorption band in the pH range of 7–4 and there by showed reasonable pH sensitivity in the range of pH 4–7.
Metal sensing
To explore the optical response of RGB antenna 1 towards metal ions, spectroscopic studies were performed with addition of metal ions. We recently reported that the aza-BODIPY moiety in FRET cassettes undergo a redox transformation in the presence of Fe3+ and Co2+ that leads to the depletion of its absorption band due to radical cation/anion generation leading to a ratiometric FRET turn-off sensing behavior.68
Different metal ions such as Ag+, Na+, K+, Li+, Mg2+, Ba2+, Mn2+, Ni2+, Pb2+, Co2+, Cu2+, Fe2+, Zn2+, Cd2+, and Al3+ were added to a solution of RGB antenna 1 in THF and the absorption and emission spectra were recorded (Fig. S26 and S27‡). Among all metals, antenna 1 was responsive to only Fe3+ and Co2+ characterized by a significant depletion of the aza-BODIPY band. As a result of depleted aza-BODIPY absorption in the presence of Co2+ and Fe3+, its spectral overlap with PDI reduced drastically leading to FRET turn-off. UV/Vis and fluorescence titrations of 1 with Co2+ (Fig. 8a, b) and Fe3+ (Fig. 8c, d) were performed and the emission data in the two channels were used to calculate the association constants using the Merkx model69 for ratiometric fluorescence in the two emission channels. Accordingly, association constants of 10.25 × 104 M−1 and 2.94 × 104 M−1 (Table S8‡) were obtained for RGB antenna 1 for its binding to Co2+ and Fe3+ respectively (Fig. S28 and S29‡). Thus, metal ion induced modulation of the R band led to conversion of the RGB antenna into a GB antenna (RGB → GB). The corresponding Jobs plots of RGB antenna 1 with Co2+ and Fe3+ led to a possible binding stoichiometry of 1
:
2 for both 1: Co2+ and 1: Fe3+ (Fig. S30‡). In a similar manner, UV/Vis and fluorescence titrations of RB antenna 6 were performed with Co2+ (Fig. S31‡) and Fe3+ (Fig. S33‡). From the emission titration, association constants of 12.90 × 104 M−1 and 4.17 × 104 M−1 (Table S7‡) were obtained respectively for Co2+ (Fig. S32‡) and Fe3+ respectively using the Merkx model (Fig. S34‡). The origin of high selectivity of Fe3+ can be explained based on the calculated charge density value (ρ ∼ 4.30) which is the highest among those of first row transition metals and alkaline earth metals.70 Due to the highest charge density of Fe3+, it can form complexes with electron deficient aza-BODIPY. Furthermore, Fe3+ by virtue of its two accessible oxidation states (i.e., +2 and +3), can participate in electron transfer processes71 which are otherwise not possible if the metal has only one accessible oxidation state. Previously we have elucidated that the sensing of Co2+ and Fe3+ sensing by aza-BODIPY is a redox-driven process.68 The same rationale can be applied to Co2+, since it can readily adopt +2 or +3 states and can therefore participate in charge transfer processes to or from aza-BODIPY. The plausible mechanism for Co2+/Fe3+ ions sensing is provided in the ESI.‡ A comparison table for all the literature reported RGB systems (polymers, self-assembled materials, covalently connected chromophores, peptide scaffolds etc.) with their applications in sensing and in other areas is presented in ESI, Table S8.‡
 |
| Fig. 8 Absorption and emission spectra of RGB antenna 1 (λex = 430 nm) (c ∼ 5 μM) on titration with increasing equivalents of (a) and (b) Co2+; (c) and (d) Fe3+ perchlorate salts in THF. Initial and final spectra are represented by blue and red colours respectively. | |
Switchable or tunable fluorescent inks
The tunability of spectral properties of model antennae RB 6, GB 12 and final RGB antenna 1 with different stimuli inspired us to utilize these antennae as switchable fluorescent inks.72–79 Firstly, antenna-poly(methyl methacrylate (PMMA) composites were prepared by dissolving RB 6, GB 12 and RGB 1 (c ∼ 10−3 M) and PMMA (1 wt%) in chloroform. Filter paper with text written with RB 6-PMMA composite appeared yellowish green under normal light which on exposure to TFA changed to an olive green colour and comes back to the original colour after ∼5 minutes (Fig. 9a). GB 12-PMMA composite coated on filter paper showed bright orange fluorescence which on exposure to TFA changed to pink and after ∼5 minutes returned to the original colour, while with heat (∼60 °C) it changed to a dull orange colour and the original colour was recovered upon cooling down to room temperature (RT) (Fig. 9b). RGB antenna 1-PMMA composite was used to write text on a filter paper that showed reddish emission which on TFA exposure became very faint and almost invisible to the naked eye and was recovered to the initial colour with time. On heating (∼60 °C) RGB 1-PMMA the initial reddish fluorescence changed to orange and after cooling down to RT it reverted back to the original colour. On dipping the filter paper with text written using RGB 1-PMMA in aqueous Fe3+ solution, followed by washing and drying, the fluorescence changed irreversibly from reddish to very pale orange (Fig. 9c). Such fluorescent inks that are responsive to multiple stimuli as demonstrated can potentially serve in anti-counterfeiting applications and technologies.
 |
| Fig. 9 (a) Reversible colour change of text written with the RB 6-PMMA (1 wt%) solution in CHCl3 on filter paper upon TFA exposure (picture taken in day light); similar fluorescent inks based on text written on filter paper with (b) GB 12-PMMA and (c) RGB 1-PMMA solutions in CHCl3 under different stimuli such as TFA vapour, temperature and Fe3+ aqueous solution. (UV light; λex = 365 nm). | |
Conclusions
In summary, a RGB multichromophoric antenna 1 with Np and PDI as energy donors and a central aza-BODIPY energy acceptor was designed that showed efficient cascade FRET of ∼100% from peripheral Nps and PDIs to central aza-BODIPY. RGB antenna 1 showed pronounced temperature-dependent emission behaviour where intensities in two emission channels could be tuned in opposite directions by temperature variation giving rise to a unique ratiometric sensing with a sensitivity of 0.4% °C. RB 6, GB 12 and RGB 1 showed distinct solution phase electrochromism. Upon variation in pH, RGB antenna 1 showed a reversible absorption modulation in the blue absorption region (RGB ↔ RG). Furthermore, RGB antenna 1 was utilized to selectively sense Co2+ and Fe3+ through a FRET turn-off mechanism induced by a redox process at the aza-BODIPY site that manifested as a spectral modulation in the red region (RGB → GB). White light emission with CIE coordinates (0.32, 0.33) was obtained for RB 6 at a particular equiv. of TFA (∼22
003 equiv.). Such a multifunctional FRET based RGB antenna is a powerful sensor for precise multiparameter (temperature, pH, metal ions) detection and sensing. Applications of such multifunctional small molecular sensors in detection of pH, temperature or metal ions in cellular microenvironments are thus envisaged and will be pursued in future. Furthermore, these antennae-PMMA polymer composites were utilized as reversible thermo-responsive, acid sensitive and metal ion sensitive irreversible fluorescent inks.
Data availability
Experimental data associated with this work have been provided in the ESI.‡
Author contributions
The manuscript was written through contributions of all the authors. All authors have given approval to the final version of the manuscript.
Conflicts of interest
There are no conflicts to declare.
Acknowledgements
This work was financially supported by IISER Mohali and DST (DST/TM/SERI/FR/162) and K. R. thanks CSIR for a PhD fellowship. The authors sincerely acknowledge central NMR and mass facilities as well as various departmental facilities of IISER Mohali. The authors like to thank the anonymous reviewers whose suggestions have helped in improving the quality of the manuscript.
Notes and references
- G. D. Scholes, G. R. Fleming, A. Olaya-Castro and R. van Grondelle, Nat. Chem., 2011, 3, 763–774 CrossRef CAS PubMed
.
- A. Harriman, Chem. Commun., 2015, 51, 11745–11756 RSC
.
- T. Mirkovic, E. E. Ostroumov, J. M. Anna, R. van Grondelle, Govindjee and G. D. Scholes, Chem. Rev., 2017, 117, 249–293 CrossRef CAS PubMed
.
- D. Gust, T. A. Moore and A. L. Moore, Acc. Chem. Res., 2001, 34, 40–48 CrossRef CAS PubMed
.
- T. Brixner, R. Hildner, J. Köhler, C. Lambert and F. Würthner, Adv. Energy Mater., 2017, 7, 1–33 Search PubMed
.
- G. McDermott, S. M. Prince, A. A. Freer, A. M. Hawthornthwaite-Lawless, M. Z. Papiz, R. J. Cogdell and N. W. Isaacs, Nature, 1995, 374, 517–521 CrossRef CAS
.
- W. Kühlbrandt, D. N. Wang and Y. Fujiyoshi, Nature, 1994, 367, 614–621 CrossRef PubMed
.
- T. Keijer, T. Bouwens, J. Hessels and J. N. H. Reek, Chem. Sci., 2021, 12, 50–70 RSC
.
- R. Ziessel and A. Harriman, Chem. Commun., 2011, 47, 611–631 RSC
.
- T. T. T. Nguyen, D. Türp, D. Wang, B. Nölscher, F. Laquai and K. Müllen, J. Am. Chem. Soc., 2011, 133, 11194–11204 CrossRef CAS PubMed
.
- K. R. J. Thomas, A. L. Thompson, A. V. Sivakumar, C. J. Bardeen and S. Thayumanavan, J. Am. Chem. Soc., 2005, 127, 373–383 CrossRef CAS PubMed
.
- T. Weil, E. Reuther, C. Beer and K. Müllen, Chem.–Eur. J., 2004, 10, 1398–1414 CrossRef CAS PubMed
.
- N. Aratani, D. Kim and A. Osuka, Acc. Chem. Res., 2009, 42, 1922–1934 CrossRef CAS PubMed
.
- L. Beverina, G. A. Pagani and M. Sassi, Chem. Commun., 2014, 50, 5413–5430 RSC
.
- D. Holten, D. F. Bocian and J. S. Lindsey, Acc. Chem. Res., 2002, 35, 57–69 CrossRef CAS PubMed
.
- Y. Nakamura, N. Aratani and A. Osuka, Chem. Soc. Rev., 2007, 36, 831–845 RSC
.
- S. Sengupta and F. Würthner, Acc. Chem. Res., 2013, 46, 2498–2512 CrossRef CAS PubMed
.
- Q. Zou, K. Liu, M. Abbas and X. Yan, Adv. Mater., 2016, 28, 1031–1043 CrossRef CAS PubMed
.
- K. V. Rao, K. K. R. Datta, M. Eswaramoorthy and S. J. George, Adv. Mater., 2013, 25, 1713–1718 CrossRef CAS PubMed
.
- H. Peng, L. Niu, Y. Chen, L. Wu, C. Tung and Q. Yang, Chem. Rev., 2015, 115, 7502–7542 CrossRef CAS PubMed
.
- J. Fan, M. Hu, P. Zhan and X. Peng, Chem. Soc. Rev., 2013, 42, 29–43 RSC
.
- J.-J. Li, H.-Y. Zhang, X.-Y. Dai, Z.-X. Liu and Y. Liu, Chem. Commun., 2020, 56, 5949–5952 RSC
.
- J. M. Serin, D. W. Brousmiche and J. M. J. Fréchet, Chem. Commun., 2002, 2, 2605–2607 RSC
.
- T. Weil, E. Reuther and K. Müllen, Angew. Chem.,
Int. Ed., 2002, 41, 1900–1904 CrossRef CAS PubMed
.
- W. R. Dichtel, S. Hecht and J. M. J. Fréchet, Org. Lett., 2005, 7, 4451–4454 CrossRef CAS PubMed
.
- R. Métivier, F. Kulzer, T. Weil, K. Müllen and T. Basché, J. Am. Chem. Soc., 2004, 126, 14364–14365 CrossRef PubMed
.
- M. Cotlet, T. Vosch, S. Habuchi, T. Weil, K. Müllen, J. Hofkens and F. De Schryver, J. Am. Chem. Soc., 2005, 127, 9760–9768 CrossRef CAS PubMed
.
- O. Altan Bozdemir, S. Erbas-Cakmak, O. O. Ekiz, A. Dana and E. U. Akkaya, Angew. Chem., Int. Ed., 2011, 50, 10907–10912 CrossRef CAS PubMed
.
- R. Ziessel, G. Ulrich, A. Haefele and A. Harriman, J. Am. Chem. Soc., 2013, 135, 11330–11344 CrossRef CAS PubMed
.
- S. Diring, F. Puntoriero, F. Nastasi, S. Campagna and R. Ziessel, J. Am. Chem. Soc., 2009, 131, 6108–6110 CrossRef CAS PubMed
.
- A. Uetomo, M. Kozaki, S. Suzuki, K. Yamanaka, O. Ito and K. Okada, J. Am. Chem. Soc., 2011, 133, 13276–13279 CrossRef CAS PubMed
.
- A. Harriman, L. J. Mallon, S. Goeb, G. Ulrich and R. Ziessel, Chem.–Eur. J., 2009, 15, 4553–4564 CrossRef CAS PubMed
.
- A. Harriman, L. Mallon and R. Ziessel, Chem.–Eur. J., 2008, 14, 11461–11473 CrossRef CAS PubMed
.
- C. Lambert, J. Hoche, M. H. Schreck, M. Holzapfel, A. Schmiedel, J. Selby, A. Turkin and R. Mitric, J. Phys. Chem. A, 2021, 125, 2504–2511 CrossRef CAS PubMed
.
- L. Rocard, D. Wragg, S. A. Jobbins, L. Luciani, J. Wouters, S. Leoni and D. Bonifazi, Chem.–Eur. J., 2018, 24, 16136–16148 CrossRef CAS PubMed
.
- P. Ensslen and H. A. Wagenknecht, Acc. Chem. Res., 2015, 48, 2724–2733 CrossRef CAS PubMed
.
- O. Kulyk, L. Rocard, L. Maggini and D. Bonifazi, Chem. Soc. Rev., 2020, 49, 8400–8424 RSC
.
- G. J. Hedley, A. Ruseckas and I. D. W. Samuel, Chem. Rev., 2017, 117, 796–837 CrossRef CAS PubMed
.
- Z. Zhang, Z. Zhao, Y. Hou, H. Wang, X. Li, G. He and M. Zhang, Angew. Chem., Int. Ed., 2019, 58, 8862–8866 CrossRef CAS PubMed
.
- M. Li, S. Long, Y. Kang, L. Guo, J. Wang, J. Fan, J. Du and X. Peng, J. Am. Chem. Soc., 2018, 140, 15820–15826 CrossRef CAS PubMed
.
- M. Li, A. Lee, K. L. Kim, J. Murray, A. Shrinidhi, G. Sung, K. M. Park and K. Kim, Angew. Chem., Int. Ed., 2018, 57, 2120–2125 CrossRef CAS PubMed
.
- L. Wu, C. Huang, B. P. Emery, A. C. Sedgwick, S. D. Bull, X. P. He, H. Tian, J. Yoon, J. L. Sessler and T. D. James, Chem. Soc. Rev., 2020, 49, 5110–5139 RSC
.
- M. M. A. Mazza and F. M. Raymo, J. Mater. Chem. C, 2019, 7, 5333–5342 RSC
.
- E. J. McLaurin, L. R. Bradshaw and D. R. Gamelin, Chem. Mater., 2013, 25, 1283–1292 CrossRef CAS
.
- H. Zhang, Y. Zhai, M. Wang, S. Dong, Y. Fang and L. Zhang, Nanoscale, 2019, 11, 3718–3724 RSC
.
- P. Q. Nhien, W. L. Chou, T. T. K. Cuc, T. M. Khang, C. H. Wu, N. Thirumalaivasan, B. T. B. Hue, J. I. Wu, S. P. Wu and H. C. Lin, ACS Appl. Mater. Interfaces, 2020, 12, 10959–10972 CrossRef CAS PubMed
.
- Q. Zhang, C. Y. Tsai, L. J. Li and D. J. Liaw, Nat. Commun., 2019, 10, 2–9 CrossRef PubMed
.
- C. Maiti and D. Dhara, Langmuir, 2017, 33, 12130–12139 CrossRef CAS PubMed
.
- F. C. Liang, C. C. Kuo, B. Y. Chen, C. J. Cho, C. C. Hung, W. C. Chen and R. Borsali, ACS Appl. Mater. Interfaces, 2017, 9, 16381–16396 CrossRef CAS PubMed
.
- C. Li, Y. Zhang, J. Hu, J. Cheng and S. Liu, Angew. Chem., Int. Ed., 2010, 49, 5120–5124 CrossRef CAS PubMed
.
- Z. Xu, S. Peng, Y.-Y. Wang, J.-K. Zhang, A. I. Lazar and D.-S. Guo, Adv. Mater., 2016, 28, 7666–7671 CrossRef CAS PubMed
.
- X. Wan and S. Liu, J. Mater. Chem., 2011, 21, 10321–10329 RSC
.
- H. Q. Dong, T. B. Wei, X. Q. Ma, Q. Y. Yang, Y. F. Zhang, Y. J. Sun, B. B. Shi, H. Yao, Y. M. Zhang and Q. Lin, J. Mater. Chem. C, 2020, 8, 13501–13529 RSC
.
- F. Würthner, C. R. Saha-Möller, B. Fimmel, S. Ogi, P. Leowanawat and D. Schmidt, Chem. Rev., 2016, 116, 962–1052 CrossRef PubMed
.
- Y. Ge and D. F. O'Shea, Chem. Soc. Rev., 2016, 45, 3846–3864 RSC
.
- F. Schweighöfer, L. Dworak, C. A. Hammer, H. Gustmann, M. Zastrow, K. Rück-Braun and J. Wachtveitl, Sci. Rep., 2016, 6, 1–9 CrossRef PubMed
.
- V. Sláma, V. Perlík, H. Langhals, A. Walter, T. Mančal, J. Hauer and F. Šanda, Front. Chem., 2020, 8, 1–12 CrossRef PubMed
.
- H. Langhals, S. Poxleitner, O. Krotz, T. Pust and A. Walter, Eur. J. Org. Chem., 2008, 4559–4562 CrossRef CAS
.
- H. Langhals and A. Walter, J. Phys. Chem. A, 2020, 124, 1554–1560 CrossRef CAS PubMed
.
- W. Zhang, J. Li, H. Lei and B. Li, Opt. Express, 2020, 28, 12450–12459 CrossRef CAS PubMed
.
- Z. Zhang, Z. Zhao, L. Wu, S. Lu, S. Ling, G. Li, L. Xu, L. Ma, Y. Hou, X. Wang, X. Li, G. He, K. Wang, B. Zou and M. Zhang, J. Am. Chem. Soc., 2020, 142, 2592–2600 CrossRef CAS PubMed
.
-
P. Monk, R. Mortimer and D. Rosseinsky, in Electrochromism and Electrochromic Devices, Cambridge University Press, Cambridge, 2009, pp. 1–24 Search PubMed
.
- H. Lim, S. Seo, S. Pascal, Q. Bellier, S. Rigaut, C. Park, H. Shin, O. Maury, C. Andraud and E. Kim, Sci. Rep., 2016, 6, 1–8 CrossRef PubMed
.
- W. Q. Deng, A. H. Flood, J. F. Stoddart and W. A. Goddard, J. Am. Chem. Soc., 2005, 127, 15994–15995 CrossRef CAS PubMed
.
- N. Elool Dov, S. Shankar, D. Cohen, T. Bendikov, K. Rechav, L. J. W. Shimon, M. Lahav and M. E. Van Der Boom, J. Am. Chem. Soc., 2017, 139, 11471–11481 CrossRef CAS PubMed
.
- X. Yu, C. Zhan, X. Ding, S. Zhang, X. Zhang, H. Liu, L. Chen, Y. Wu, H. Fu, S. He, Y. Huang and J. Yao, Phys. Chem. Chem. Phys., 2013, 15, 11960–11965 RSC
.
- Y. Tu, Y. Ju, D. Xiao, J. Liu, Z. Zhao, Z. Liu, J. W. Y. Lam and B. Z. Tang, Adv. Sci., 2020, 7, 1–9 Search PubMed
.
- K. Rani, U. K. Pandey and S. Sengupta, J. Mater. Chem. C, 2021, 9, 4607–4618 RSC
.
- A. M. Hessels and M. Merkx, ACS Sens, 2016, 1, 498–502 CrossRef CAS
.
- S. Huang, P. Du, C. Min, Y. Liao, H. Sun and Y. Jiang, J. Fluoresc., 2013, 23, 621–627 CrossRef CAS PubMed
.
- S. D. Padghan, R. S. Bhosale, S. V. Bhosale, F. Antolasic, M. Al Kobaisi and S. V. Bhosale, Molecules, 2017, 22, 1417 CrossRef PubMed
.
- E. R. Sauvé, C. M. Tonge and Z. M. Hudson, J. Am. Chem. Soc., 2019, 141, 16422–16431 CrossRef PubMed
.
- X. Chen, J. Chen, G. Sun, L. Guo, J. Su and Z. Zhang, ACS Appl. Mater. Interfaces, 2021, 13, 38629–38636 CrossRef CAS PubMed
.
- H. J. Kim, D. R. Whang, J. Gierschner, C. H. Lee and S. Y. Park, Angew. Chem., Int. Ed., 2015, 54, 4330–4333 CrossRef CAS PubMed
.
- S. Liu, J. Wang, F. Tang, N. Wang, L. Li, C. Yao and L. Li, ACS Appl. Mater. Interfaces, 2020, 12, 55269–55277 CrossRef CAS PubMed
.
- P. Q. Nhien, T. T. K. Cuc, T. M. Khang, C. H. Wu, B. T. B. Hue, J. I. Wu, B. W. Mansel, H. L. Chen and H. C. Lin, ACS Appl. Mater. Interfaces, 2020, 12, 47921–47938 CrossRef CAS PubMed
.
- X. C. Yang, Q. Li, M. Tang, Y. L. Yang, W. Yang, J. F. Hu, X. L. Pu, J. Liu, J. T. Zhao and Z. J. Zhang, ACS Appl. Mater. Interfaces, 2020, 12, 20849–20858 CrossRef CAS PubMed
.
- J.-C. Yang, Y.-C. Ho and Y.-H. Chan, ACS Appl. Mater. Interfaces, 2019, 11, 29341–29349 CrossRef CAS PubMed
.
- P. Gayathri, M. Pannipara, A. G. Al-Sehemi, D. Moon and S. P. Anthony, Mater. Adv., 2021, 2, 996–1005 RSC
.
Footnotes |
† Förster resonance energy transfer. |
‡ Electronic supplementary information (ESI) available. See DOI: 10.1039/d1sc05112a |
|
This journal is © The Royal Society of Chemistry 2021 |
Click here to see how this site uses Cookies. View our privacy policy here.