DOI:
10.1039/D1SC04825B
(Edge Article)
Chem. Sci., 2021,
12, 14855-14862
Enzyme-activated near-infrared fluorogenic probe with high-efficiency intrahepatic targeting ability for visualization of drug-induced liver injury†
Received
1st September 2021
, Accepted 17th October 2021
First published on 20th October 2021
Abstract
Hepatotoxicity is a serious problem faced by thousands of clinical drugs, and drug-induced liver injury (DILI) caused by chronic administration or overdose has become a major biosafety issue. However, the near-infrared (NIR) fluorescent probes currently used for liver injury detection still suffer from poor liver targeting ability and low sensitivity. Enzyme-activated fluorogenic probes with powerful in situ targeting ability are the key to improving the imaging effect of liver injury. Herein, we rationally designed a leucine aminopeptidase (LAP) activated fluorogenic probe hCy-CA-LAP, which greatly improved the hepatocyte-targeting capability by introducing a cholic acid group. The probe hCy-CA-LAP is converted into a high-emission hCy-CA fluorophore in the presence of LAP, showing high selectivity, high sensitivity and low detection limit (0.0067 U mL−1) for LAP, and successfully realizes the sensitive detection of small fluctuations of LAP in living cells. Moreover, the probe can achieve effective in situ accumulation in the liver, thereby achieving precise imaging and evaluation of two different types of drug-induced hepatotoxicity in vivo. Therefore, the probe hCy-CA-LAP may be a potential tool for exploring the roles of LAP and evaluating the degree of DILI.
Introduction
As the primary metabolic organ of the human body, the liver is responsible for the biotransformation of endogenous metabolites and exogenous drugs.1,2 Currently, more than one thousand drugs in clinical use are known to have hepatotoxicity.3 Overdose or chronic administration of drugs has become the major safety concern in drug-induced liver injuries (DILIs), such as acute liver injury, hepatitis, fibrosis, and cholestatic liver injury.4 For the diagnosis of DILI, the abnormal activities of alanine aminotransferase (ALT) and aspartate aminotransferase (AST) in serum are considered to be the clinical standard, but their appearance in muscle injury and kidney injury may lead to false positives in the diagnosis.5,6 In addition, with their secretion and accumulation, when these two indicators rise to an identifiable level, patients has already suffered from severe liver disease, and has lost the opportunity for timely treatment and preventing disease progression.7,8 With the conspicuous advancement of imaging technology, fluorescent probes that can non-invasively visualize physiological and pathological processes at the molecular level have become an irreplaceable strategy for early and accurate diagnosis of diseases, thereby effectively preventing advanced diseases.9–14
Leucine aminopeptidase (LAP), widely distributed in organisms, catalyzes the crucial step in the synthesis of bioactive peptides.15,16 In DILI, hepatic protection mechanisms help to significantly up-regulate the activity of LAP, even in the early stages.17,18 In view of these key features, LAP is often selected as an in situ biomarker and specific trigger to develop activatable fluorescent probes for accurate diagnosis of DILI at an early stage.17,19,20 Compared with always-on probes, activatable fluorogenic probes with high selectivity and low background signal provide a high signal-to-background ratio (SBR) when detecting biomarkers of interest.21–25 At the same time, in order to reduce organic autofluorescence and scattering, and improve the sensitivity, NIR probes facilitate in vivo high SBR imaging, because they have numerous advantages such as minimum photodamage to biological samples, low interference from tissue autofluorescence and deeper signal feedback depth.26–29 He et al. successfully reported an activatable NIR fluorogenic probe for high contrast in situ imaging of LAP activity in a DILI mouse model.30 Yuan et al. screened and designed two chemically stable activatable NIR fluorogenic probes for in vivo imaging of DILI-related biomarkers (LAP and ONOO−), and visually evaluated the therapeutic effects of clinical hepatoprotective drugs.31 Wang et al. designed LAP-activated fluorescent probes based on intramolecular rearrangement for high SBR detection of LAP in living systems.32,33
Although the currently reported probes provide the primary merits of high selectivity, real-time feedback and tailor-made features, small molecular fluorogenic probes for visualization of drug-induced liver injury still have obvious disadvantages.11,34,35 Most imaging probes enter the liver through passive targeting and suffer from rapid clearance in the liver, which makes it difficult for those probes to accumulate adequately inside the liver within a short time, resulting in low signal strength and sensitivity in the liver region.36,37 Active targeting strategies can significantly improve the accumulation of small molecular probes in the region of interest by introducing functional groups.27,38–41 Therefore, it is highly desirable to design probes with stronger intrahepatic targeting capability to improve the in situ accumulation and imaging sensitivity of the probe in the liver, which can apparently facilitate the diagnosis of DILI as well as further research on the clinical biological characteristics of biomarkers. As a signalling molecule, cholic acid is involved in multiple pathways in the liver, and can be actively transported into hepatocytes, showing inherent hepatocyte targeting.42–44 In view of the key features, a few dyes and prodrugs modified with cholic acid have been designed to enhance their in situ delivery in the liver.36,45,46 Therefore, cholic acid can serve as a potential group to develop probes with efficient hepatic targeting ability.
In this work, we report a cholic acid-modified NIR fluorogenic probe (hCy-CA-LAP) for fluorescence imaging of DILI. The introduction of cholic acid can improve the intrahepatic targeting capability of hCy-CA-LAP, thereby significantly increasing the enrichment of probe molecules in the liver.36,47hCy-CA-LAP is composed of an NIR fluorescence signaling moiety (hemicyanine fluorophore, hCy), a hepatocyte-targeting moiety (cholic acid) for efficient hepatic enrichment, and a LAP recognition moiety (L-leucine amide). The recognition part is connected to the fluorophore hCy-CA through a self-immolative linker, and serves as the LAP-responsive part and fluorescence quencher (Scheme 1). The probe hCy-CA-LAP can achieve effective in situ accumulation in the liver, where it is converted into a high-emission hCy-CA fluorophore by the pathological level of LAP in DILI. The combination of activatability, NIR fluorescence and highly targeted accumulation can effectively improve the in vivo imaging effect and realize the highly sensitive and intensive diagnosis of DILI in the early stage.
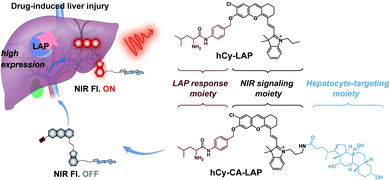 |
| Scheme 1 Chemical structures of hCy-CA-LAP and hCy-LAP, and schematic illustration of hCy-CA-LAP for fluorescence imaging of LAP in DILI. | |
Results and discussion
Design and synthesis of hCy-CA-LAP and hCy-LAP
Here, we designed an NIR fluorogenic probe hCy-CA-LAP with efficient intrahepatic targeting and LAP response characteristics. The hCy skeleton was chosen as the signal reporter because of its strong NIR fluorescence and multiple modifiable sites for introducing the targeting group and analyte response group.35hCy-NH2 was synthesized according to the reported method.48 First, the intrahepatic targeting group cholic acid was incorporated into the fluorophore to obtain hCy-CA, which is the precursor of the probe hCy-CA-LAP. hCy-CA consists of a hydroxyl group at the end of the NIR fluorophore, which is the reason for its high quantum yield. Subsequently, Fmoc-Leu-Br was introduced into the precursor through a nucleophilic substitution reaction, and finally the probe hCy-CA-LAP was obtained after Fmoc deprotection, which we hoped would give rise to a fluorogenic LAP-reactive probe (Scheme S1†). The reduction in the fluorescence of the probe hCy-CA-LAP is attributed to the intramolecular photoinduced electron transfer (PeT) process from the excited fluorophore to the free amine. As a prediction, upon incubation with LAP, L-leucine amide in hCy-CA-LAP will be eliminated by the hydrolysis of LAP, and then a 1,6-elimination of the self-immolative linker will lead to the regeneration of free hCy-CA, leading to fluorescence recovery. At the same time, hCy-LAP without the hepatocyte-targeting moiety was constructed as a control probe. All chemical structures are fully characterized by nuclear magnetic resonance (NMR) and high-resolution electrospray ionization mass spectrometry (HRMS-ESI), as shown in the ESI (Fig. S15–S22†).
Selective detection of LAP in vitro
The optical properties of the two probes and their responsiveness to LAP were evaluated. As shown in Fig. 1, hCy-CA-LAP and hCy-LAP had maximum absorption peaks at 615 nm and 603 nm and negligible fluorescence intensity due to the quenching effect of the free amine in the reactive group (Φ < 0.01). After reacting with LAP, the maximum absorption peaks of both probes produced a bathochromic shift to about 680 nm. The fluorescence intensity of hCy-CA-LAP at 710 nm dramatically increased by 27 times (Φ = 0.20) compared with that of hCy-CA-LAP alone. Under the same conditions, the fluorescence intensity of the control probe hCy-LAP at 705 nm increased by 78-times (Φ = 0.27) in response to LAP stimulation (Table S1†). The NIR fluorescence images of both probes also showed high-contrast fluorescence changes, with no fluorescence in the probe-only sample and significantly increased fluorescence in the LAP-treated sample (Fig. 1B and D inner images). This indicates that both probes are converted into high emission fluorophores with high SBR fluorescence in the NIR region, which shows favorable sensitivity to LAP. Meanwhile, the maximum fluorescence peaks of the two probes differ by 5 nm. It is speculated that the introduction of the hydrophobic cholic acid group weakens the water solubility of the probe hCy-CA-LAP, which is related to the affinity to LAP and optical performance in aqueous solution. In addition, hCy-CA-LAP, hCy-LAP and their products displayed satisfactory photostability after 3 hours of continuous irradiation, suggesting the possibility of using them as LAP-activated NIR probes (Fig. S1†).
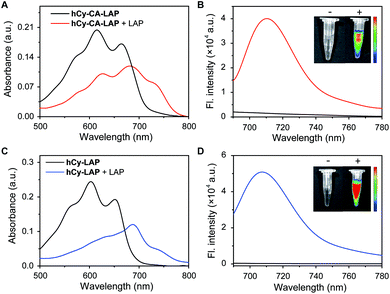 |
| Fig. 1
In vitro response of hCy-CA-LAP and hCy-LAP toward LAP. Absorption spectra and fluorescence spectra of hCy-CA-LAP (A and B) and hCy-LAP (C and D) (10 µM) in the absence and presence of LAP (0.2 U mL−1), λex = 680 nm. (Insets B and D) NIR fluorescence images of the two probes before and after reacting with LAP, λex = 640 nm and λem = 740 nm. All spectra were recorded in PBS buffer (10 mM, pH = 7.4, containing 1% DMSO) at 37 °C. | |
A more detailed in vitro selective detection of LAP was performed. As shown in Fig. 2A, the time curve of the fluorescence intensity of hCy-CA-LAP and different concentrations of LAP at 710 nm showed that the reaction reached a plateau within 1 h. Under the same conditions, the fluorescence intensity of hCy-LAP at 705 nm reached a plateau in about 40 min (Fig. S2A†). Taking into account the difference in response equilibrium times of the two probes, the incubation time was 60 min for hCy-CA-LAP and 40 min for hCy-LAP throughout the in vitro experiment. The fluorescence changes of hCy-CA-LAP in the presence of different concentrations of LAP are presented in Fig. 2B. A higher concentration of LAP can promote the reaction with hCy-CA-LAP and the release of the fluorophore. At the same time, the fluorescence intensity of hCy-CA-LAP at 710 nm gradually increased with incremental concentrations of LAP, and reached a basic equilibrium when the LAP concentration was 0.075 U mL−1, and exhibited good linearity at low concentrations of LAP (0–0.075U mL−1) (Fig. 2C and D). A similar trend in fluorescence response to different concentrations of LAP was also observed when testing hCy-LAP (Fig. S2†). The limit of detection (LOD) of hCy-CA-LAP and hCy-LAP was determined to be 0.0067 U mL−1 and 0.002 U mL−1 calculated using the formula 3σ/k, respectively (Table S1†). Therefore, hCy-CA-LAP and hCy-LAP are capable of well quantifying the content and activity of LAP based on the changes in fluorescence.
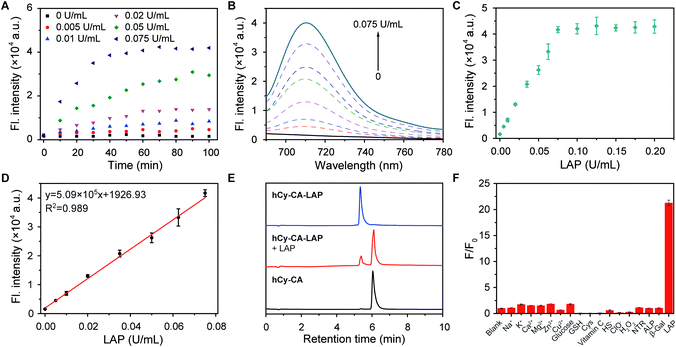 |
| Fig. 2
In vitro response of hCy-CA-LAP toward LAP. (A) The sensing kinetics of hCy-CA-LAP (F710 nm) and (B) fluorescence responses of hCy-LAP (10 µM) to different concentrations of LAP (0–0.075 U mL−1). (C) Fluorescence intensity of hCy-CA-LAP (10 µM) at 710 nm with different concentrations of LAP (0–0.2 U mL−1). (D) Linear correlation between the fluorescence intensity of hCy-CA-LAP at 710 nm and low concentrations of LAP (0–0.075 U mL−1). (E) Chromatograms of hCy-CA-LAP, hCy-CA-LAP reacting with LAP and hCy-CA, λabs = 600 nm. (F) Fluorescence response of hCy-CA-LAP (10 µM) to different analytes (analytes: 1 mM, enzyme: 0.1 U mL−1) in PBS buffer (pH 7.4) at 37 °C. λex = 680 nm, λem = 705 nm. | |
High-performance liquid chromatography (HPLC) and HRMS-ESI were used to further verify the above reaction mechanism. HPLC results showed that probe hCy-CA-LAP had a clear characteristic peak at 5.341 min. After the addition of LAP, the probe peak decreased significantly and a new peak appeared (retention time, TR = 6.048 min), which was the same as that of the product hCy-CA (Fig. 2E). Synchronous mass spectrometry further confirmed the results. The mass spectrum clearly showed the characteristic peak of hCy-CA-LAP with a molecular weight of m/z = 1069.6185 [M]+ (calculated m/z = 1069.6180 [M]+) (Fig. S3†). After treating with LAP, a new mass peak appeared at m/z = 851.4788 [M]+, which was consistent with the expected product hCy-CA (calculated m/z = 851.4760, [M]+) (Fig. S4†). This is strong evidence confirming the successful release of hCy-CA by the LAP-catalyzed elimination of the L-leucine amide moiety and the spontaneous elimination of the self-immolative linker, which is consistent with the expected reaction mechanism. HPLC and HRMS-ESI data also demonstrated the successful release of hCy (TR = 5.975 min, m/z = 446.1889 [M]+) from hCy-LAP (TR = 5.300 min, m/z = 664.3382 [M]+) after incubating with LAP (Fig. S5–S7†).
Subsequently, to demonstrate the potential for unique recognition of LAP, the selectivity of the LAP-activated probe in the presence of other potential coexisting interferents was evaluated, including ions (Na+, K+, Ca2+, Mg2+, Zn2+, Cu2+), biomolecules (glucose, glutathione, cysteine, vitamin C), redox species (HS−, ClO−, H2O2) and related enzymes (nitroreductase, alkaline phosphatase, β-galactosidase). The results showed that fluorescence intensity was negligible after incubation of hCy-CA-LAP or hCy-LAP with those potential interferents (Fig. 2F and S8†). Both hCy-CA-LAP and hCy-LAP can react rapidly with the individual enzyme LAP, with a strong fluorescence enhancement, rather than with other potential interference species. In addition, a change in pH from 5.0 to 9.0 had little effect on the response of the two probes to LAP, indicating that both probes performed well under physiological conditions (Fig. S9†). Therefore, they can serve as effective probes for selective detection of LAP under complex physiological conditions.
Fluorescence imaging of endogenous LAP in living cells
Inspired by the outstanding performance in vitro, we evaluated the potential of these two probes to monitor endogenous LAP in living cells. The biocompatibility of the probe was evaluated by exposing LO2 or HepG2 cells to various concentrations of hCy-CA-LAP or hCy-LAP for 24 h, respectively. As shown in Fig. S10,† more than 95% of LO2 and HepG2 cells survived even at 10 µM hCy-CA-LAP, indicating that the cytotoxicity of hCy-CA-LAP to living cells was negligible. To our surprise, for the control probe, 10 µM hCy-LAP significantly reduced the cell viability, where the viability of LO2 cells was 47% and that of HepG2 cells was only 34%. Therefore, only hCy-CA-LAP with good biocompatibility was further used to detect and monitor endogenous LAP in LO2 and HepG2 cells.
Acetaminophen (APAP) treatment at a toxic dosage has been proved to induce hepatocyte injury, accompanied by a complex cascade of hepatic self-protection pathways.49,50 Several experiments have reported that the up-regulation of LAP is a biomarker that is significantly related to hepatocyte injury.51–53 Therefore, we tested the activity level of LAP in cells stimulated by drugs. As shown in Fig. 3A and C, the fluorescence of LO2 cells was weak after treatment with hCy-CA-LAP, which is due to the relatively low concentration of LAP in normal LO2 cells under physiological conditions. To manifest the specificity of the probe, bestatin, an activity inhibitor of LAP, was used in further study.54 After pretreatment with bestatin for 1 h, the LO2 cells incubated with hCy-CA-LAP had almost no fluorescence. Compared with the control group without bestatin, the significantly suppressed fluorescence intensity proved that the fluorescence response of hCy-CA-LAP in cells was closely related to the enzymatic activity of LAP. In the APAP stimulation group, the fluorescence of LO2 cells increased significantly after 12 h of APAP pretreatment, while the fluorescence of the APAP + bestatin + hCy-CA-LAP group was significantly suppressed compared with the group treated with APAP only. After that, the protective effect of N-acetylcysteine (NAC), a hepatic protectant used to repair APAP-induced hepatocyte injury, was tested.31 The results showed that NAC pretreatment also significantly suppressed the fluorescence intensity. A similar trend in fluorescence changes after incubation with hCy-CA-LAP was also observed in HepG2 cells (Fig. 3B and D). Therefore, the release of free hCy-CA and the unique fluorescence response of hCy-CA-LAP are indeed caused by the specific cleavage by LAP in cells. As a further proof of the concept, a comparison of fluorescence intensity was observed in two cell lines with different LAP enzyme activities. Compared with LO2 cells with a physiological level of LAP activity, after treatment with hCy-CA-LAP, brighter fluorescence was observed in HepG2 cells with highly expressed LAP. Therefore, this dramatically fluctuant fluorescence intensity was closely related to the activity and content of LAP in cells, proving that hCy-CA-LAP had excellent selectivity and sensitivity for imaging endogenous LAP in living cells.
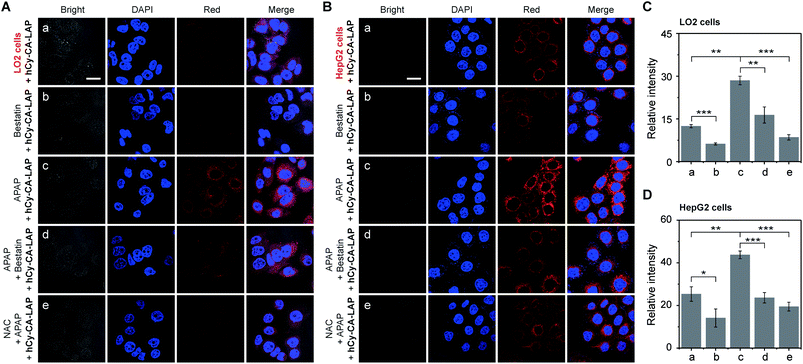 |
| Fig. 3 Fluorescence imaging of LO2 cells (A) and HepG2 cells (B) incubated with hCy-CA-LAP (10 µM) for 30 min: (a) untreated cells; (b) cells pretreated with 100 µM bestatin for 1 h; (c) cells pretreated with 1 mM APAP for 12 h; (d) cells pretreated with 1 mM APAP for 12 h, and then treated with 100 µM bestatin for 1 h; (e) cells pretreated with 100 µM NAC for 1 h, and then treated with 1 mM APAP for 12 h. (C) and (D) Relative fluorescence intensity of the corresponding fluorescence images in panels A and B, λex = 639 nm, λem = 663–738 nm. Scale bar: 25 µm. *P < 0.05, **P < 0.01, and ***P < 0.001. | |
Fluorescence imaging of LAP in a mouse model of APAP-induced acute liver injury
Acute liver injury may occur in healthy individuals without a history of related diseases or in patients who have already suffered from serious diseases.55 If left untreated, DILI can develop into severe damage.56,57 It is very important to develop intrahepatic targeted molecular probes for accurate diagnosis of acute liver injury in vivo. Although the probe hCy-LAP has shown toxicity to LO2 cells in previous cell experiments, in vivo imaging of hCy-LAP can still be used as a control probe to observe whether the additional cholic acid has a beneficial effect on imaging performance in vivo. Balb/c female mice were intraperitoneally injected with an overdose of APAP (300 mg kg−1) for 1 h as the model of acute liver injury, and mice injected with an equal volume of PBS solution were used as a control group. All mice were then injected intravenously with hCy-CA-LAP or hCy-LAP (50 µM, 100 µL) for in vivo fluorescence imaging (Fig. 4A). As shown in Fig. 4B and C, after the administration of hCy-CA-LAP, the fluorescence signal in the liver region gradually increased over time, indicating the rapid intrahepatic accumulation of hCy-CA-LAP and its rapid response to intrahepatic LAP. Throughout the imaging process, significantly higher fluorescence signals were observed in APAP-stimulated mice compared to the control group, achieving high-performance in vivo imaging of DILI with high sensitivity. The fluorescence intensity of the dissected liver which was administered hCy-CA-LAP showed a more significant increase between APAP and PBS-treated mice (Fig. 4D). The in vivo biodistribution of hCy-CA-LAP indicated that the probe was mainly distributed in the liver (Fig. S11†). Moreover, the liver tissues stained with hematoxylin & eosin (H&E) were also analyzed to evaluate liver injury. There was no obvious morphological change in the liver tissue of PBS-treated mice, while swollen hepatocytes and Kupffer cell proliferation were observed in that of APAP-treated mice (Fig. 4E). In addition, mice treated with APAP and PBS injected intravenously with hCy-LAP showed a similar trend of fluorescence change to hCy-CA-LAP (Fig. S12†). However, in contrast to the faster response and better performance of hCy-LAP than hCy-CA-LAP throughout the in vitro test, when applied to in vivo fluorescence imaging of DILI mice, the enriched imaging of the probe hCy-CA-LAP in the liver showed stronger fluorescence and higher hepatic targeting ability than hCy-LAP under the same APAP administration conditions. Although hCy-LAP showed a relatively stronger fluorescence signal in DILI mice than normal mice, further quantitative data showed that hCy-CA-LAP had a significantly stronger imaging signal at the liver site than hCy-LAP (Fig. S13†). In vivo results showed that the additional cholic acid group in the structure of hCy-CA-LAP as an intrahepatic targeting moiety can increase the enrichment of probe molecules in the liver, which will significantly improve the imaging sensitivity in the liver. Therefore, this design provides a promising strategy for effective intrahepatic targets of in vivo imaging probes and prodrugs.
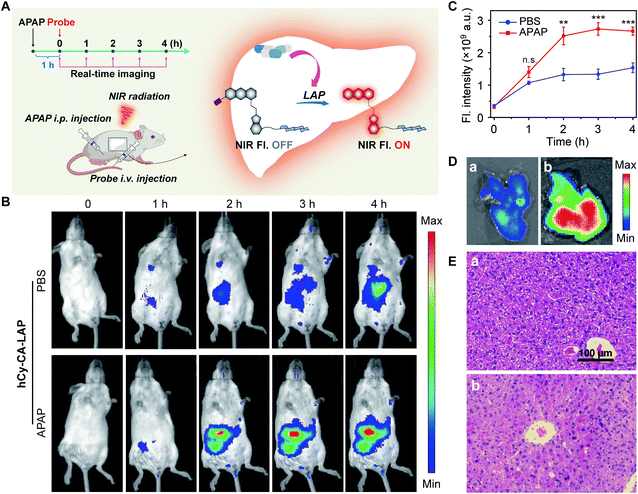 |
| Fig. 4
In vivo fluorescence imaging of APAP-induced acute liver injury. (A) Schematic diagram of APAP-induced acute liver injury mouse model construction and in vivo fluorescence imaging of LAP. (B) Time-dependent in vivo fluorescence imaging. Balb/c female mice were pretreated with APAP (300 mg kg−1, 200 µL) or PBS (200 µL) for 1 h, and then injected intravenously with hCy-CA-LAP (50 µM, 100 µL). (C) Quantification of the fluorescence signal in (B) **P < 0.01, and ***P < 0.001, and n.s.: not significant, compared with the control group (PBS-treated group). (D) Ex vivo imaging of liver dissected from mice after intravenously injected with hCy-CA-LAP (50 µM, 100 µL) for 3 h. (E) Histological study of liver tissues from Balb/c mice treated with APAP (300 mg kg−1, 200 µL) and PBS (200 µL), λex = 640 nm, λem = 740 nm. | |
Fluorescence imaging of LAP in a mouse model of RFP-induced cholestatic liver injury
Rifampicin (RFP) is a first-line oral agent for the treatment of tuberculosis.58 However, hepatotoxicity is a well-known side effect of improper use of RFP, which poses a risk to patients who take RFP for a long period.59 Biliary excretion disorder is the primary pathological symptom caused by long-term use of RFP. If left untreated, it can develop into cholestatic liver injury cirrhosis.60 Therefore, it is of great significance to develop highly sensitive and accurate methods for early detection of cholestatic liver injury.
Our aim was to image and map the enzymatic activity of LAP in a mouse model of cholestatic liver injury. Cholestatic liver injury leads to an elevated activity of intrahepatic LAP. In this experiment, untreated female Balb/c mice were randomly divided into three groups. After fasting overnight, the mice were given RFP intragastrically at a hepatotoxic dosage (300 mg kg−1 d−1) for one or three days. The control group was given carboxymethylcellulose sodium (CMC-Na) solution (200 µL) intragastrically. hCy-CA-LAP was then intravenously administered 6 h after the last drug treatment (Fig. 5A). Initially, there was no fluorescence signal in the abdomen of the mice receiving a single dose of RFP, but over time, the fluorescence signal in the upper abdomen gradually increased and matched well with the liver region, indicating elevated intrahepatic LAP levels (Fig. 5B). Compared with the single-dose RFP group, a higher fluorescence signal was observed in the upper abdomen of mice treated with 3 times the dose of RFP. In contrast, obviously weak fluorescence was observed throughout the imaging process in the CMC-Na-treated group. Similarly, the quantitative signal also showed a higher fluorescence signal than the CMC-Na-treated group for imaging RFP-induced cholestatic liver injury, indicating that the probe hCy-CA-LAP with an intrahepatic target can map the hepatic LAP level (Fig. 5C). The fluorescence intensity of the dissected liver which was administered hCy-CA-LAP showed significant differences between mice treated with RFP for one or three days and control mice (Fig. 5D). The histological analysis of liver tissue was also conducted to evaluate liver injury. There were no obvious pathological changes in the CMC-Na-treated group, indicating that 5% CMC-Na aqueous solution has no hepatotoxicity. In contrast, swollen hepatocytes were observed in mice that received a single dose of RFP. In addition to the swollen hepatocytes, more obvious pathological changes such as lipid vacuoles, cholestasis, nuclear debris and hepatocyte necrosis indicated that the liver injury in mice treated with 3 times the dose of RFP was more severe (Fig. 5E). The in vivo biodistribution of hCy-CA-LAP showed that the probe was mainly distributed in the liver (Fig. S14†). Due to its excellent imaging features, hCy-CA-LAP can serve as an effective strategy for early and accurate diagnosis of RFP-induced cholestatic liver injury and in further research on the clinical biological characteristics of biomarkers.
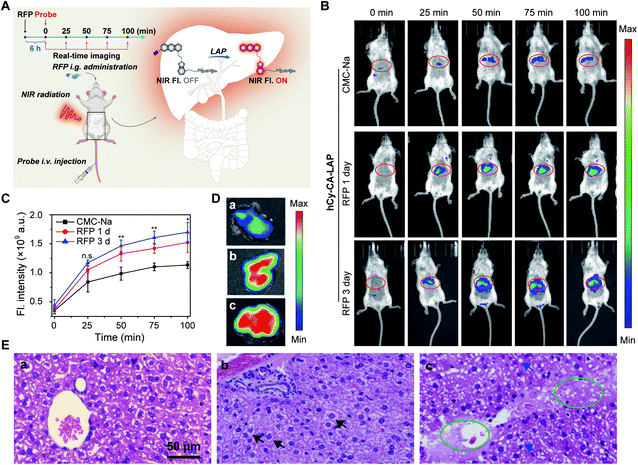 |
| Fig. 5
In vivo fluorescence imaging of RFP-induced cholestatic liver injury. (A) Schematic diagram of hCy-CA-LAP for fluorescence imaging of LAP in a mouse model of RFP-induced cholestatic liver injury. (B) Time-dependent in vivo fluorescence imaging. Balb/c female mice were given RFP (300 mg kg−1 d−1, dissolved in 200 µL aqueous solution of 0.5% CMC-Na) intragastrically for 1 or 3 days, and RFP-free CMC-Na aqueous solution (200 µL) was intragastrically given as control, and then intravenously injected with hCy-CA-LAP (50 µM, 100 µL) at 6 h after the last drug treatment. λex = 640 nm, λem = 740 nm. (C) Quantification of fluorescence signal in (B). *P < 0.05, and **P < 0.01, and n.s.: not significant, compared with the control group (CMC-Na treated group). (D) Ex vivo imaging of livers dissected from mice after intravenously injected with hCy-CA-LAP for 60 min. (E) Histology studies of liver tissues from Balb/c mice treated with RFP for 1 or 3 days and CMC-Na solution. Black arrows: swollen hepatocytes; blue arrows: lipid vacuoles; green circles: nuclear debris and necrosis of hepatocytes. | |
Conclusions
In summary, we have constructed a LAP-activated NIR fluorogenic probe with high hepatocyte-targeting ability for accurate and sensitive imaging of LAP in DILI. Probe hCy-CA-LAP provided a low detection limit for LAP (0.0067 U mL−1) and achieved sensitive detection of small fluctuations of LAP in living cells. The introduction of cholic acid realized the high-efficiency intrahepatic targeting of hCy-CA-LAP, which contributed to the highly sensitive and high-contrast imaging of LAP in vivo. Furthermore, hCy-CA-LAP further demonstrated the ability to visualize the pathological level of LAP in the mouse model of APAP or RFP induced liver injury. Therefore, our work may provide an effective strategy for facilitating DILI diagnosis, medicine evaluation and further research on the clinical and biological characteristics of biomarkers.
Data availability
Primary data for probe synthesis, characterization, photophysical measurements, and fluorescence imaging data are provided in the ESI.†
Author contributions
D. S., X. G. designed, supervised and analyzed the experiments. Y. Z., X. C. and Q. Y. contributed to the initial implementation, data analysis and manuscript preparation. Y. B., M. L. and Y. W. contributed to the synthesis and characterization. The manuscript was written through the contributions of all authors. All authors have given approval to the final version of the manuscript.
Conflicts of interest
There are no conflicts to declare.
Acknowledgements
This work was supported by the National Natural Science Foundation of China (No. 21708029, U2067214, 21727817), Beijing Municipal Education Commission-Beijing Natural Science Foundation joint funding project (KZ202010005006). All animal procedures were performed in accordance with the Guidelines for Care and Use of Laboratory Animal of Beijing University of Technology, and the animal experiment was approved by the Ethics Committee of Beijing University of Technology, China. The approval number is HS202108001.
Notes and references
- D. E. Williams, R. L. Reed, B. Kedzierski, G. A. Dannan, F. P. Guengerich and D. R. Buhler, Drug Metab. Dispos., 1989, 17, 387–392 CAS.
- J. Chen, D. Huang, M. She, Z. Wang, X. Chen, P. Liu, S. Zhang and J. Li, ACS Sens., 2021, 6, 628–640 CrossRef CAS PubMed.
- A. Pandit, T. Sachdeva and P. Bafna, J. Appl. Pharm. Sci., 2012, 2, 233–243 Search PubMed.
- C. Y. Chang and T. D. Schiano, Aliment. Pharmacol. Ther., 2007, 25, 1135–1151 CrossRef CAS PubMed.
- J. Peng, A. Samanta, X. Zeng, S. Han, L. Wang, D. Su, D. T. Loong, N. Y. Kang, S. J. Park, A. H. All, W. Jiang, L. Yuan, X. Liu and Y. T. Chang, Angew. Chem., Int. Ed., 2017, 56, 4165–4169 CrossRef CAS PubMed.
- R. A. Nathwani, S. Pais, T. B. Reynolds and N. Kaplowitz, Hepatology, 2005, 41, 380–382 CrossRef CAS PubMed.
- M. C. Kew, Lancet, 2000, 355, 591–592 CrossRef CAS.
- D. Cheng, W. Xu, X. Gong, L. Yuan and X. B. Zhang, Acc. Chem. Res., 2021, 54, 403–415 CrossRef CAS PubMed.
- X. Chen, Y. Bian, M. Li, Y. Zhang, X. Gao and D. Su, Chem.–Asian J., 2020, 15, 3983–3994 CrossRef CAS PubMed.
- D. Su, X. Chen, Y. Zhang and X. Gao, TrAC, Trends Anal. Chem., 2020, 133, 116112 CrossRef CAS.
- H. W. Liu, L. Chen, C. Xu, Z. Li, H. Zhang, X. B. Zhang and W. Tan, Chem. Soc. Rev., 2018, 47, 7140–7180 RSC.
- H. Xiao, C. Wu, P. Li, W. Gao, W. Zhang, W. Zhang, L. Tong and B. Tang, Chem. Sci., 2017, 8, 7025–7030 RSC.
- X. Ai, Z. Wang, H. Cheong, Y. Wang, R. Zhang, J. Lin, Y. Zheng, M. Gao and B. Xing, Nat. Commun., 2019, 10, 1087 CrossRef PubMed.
- P. Cheng, Q. Miao, J. Huang, J. Li and K. Pu, Anal. Chem., 2020, 92, 6166–6172 CrossRef CAS PubMed.
- M. Matsui, J. H. Fowler and L. L. Walling, Biol. Chem., 2006, 387, 1535–1544 CAS.
- M. Tsujimoto, Y. Goto, M. Maruyama and A. Hattori, Heart Fail. Rev., 2008, 13, 285–291 CrossRef CAS PubMed.
- Y. Huang, Y. Qi, C. Zhan, F. Zeng and S. Wu, Anal. Chem., 2019, 91, 8085–8092 CrossRef CAS PubMed.
- J. R. Senior, Clin. Pharmacol. Ther., 2012, 92, 332–339 CrossRef CAS PubMed.
- Y. Liu, L. Teng, C. Xu, H. W. Liu, S. Xu, H. Guo, L. Yuan and X. B. Zhang, Chem. Sci., 2019, 10, 10931–10936 RSC.
- T. Liu, M. Tian, J. Wang, X. Tian, J. Liu, L. Feng, X. Ma and J. Cui, Spectrochim. Acta, Part A, 2021, 251, 119362 CrossRef CAS PubMed.
- A. Razgulin, N. Ma and J. Rao, Chem. Soc. Rev., 2011, 40, 4186–4216 RSC.
- P. Zhang, X.-f. Jiang, X. Nie, Y. Huang, F. Zeng, X. Xia and S. Wu, Biomaterials, 2016, 80, 46–56 CrossRef CAS PubMed.
- W. Zhang, J. Liu, P. Li, X. Wang, S. Bi, J. Zhang, W. Zhang, H. Wang and B. Tang, Biomaterials, 2019, 225, 119499 CrossRef CAS PubMed.
- Z. Wang, X. Ai, Z. Zhang, Y. Wang, X. Wu, R. Haindl, E. K. L. Yeow, W. Drexler, M. Gao and B. Xing, Chem. Sci., 2019, 11, 803–811 RSC.
- L. Wu, J. Huang, K. Pu and T. D. James, Nat. Rev. Chem., 2021, 5, 406–421 CrossRef CAS.
- J. Ou-Yang, Y. Li, W. L. Jiang, S. Y. He, H. W. Liu and C. Y. Li, Anal. Chem., 2019, 91, 1056–1063 CrossRef CAS PubMed.
- M. Gao, F. Yu, C. Lv, J. Choo and L. Chen, Chem. Soc. Rev., 2017, 46, 2237–2271 RSC.
- H.-W. Liu, L. Chen, C. Xu, Z. Li, H. Zhang, X.-B. Zhang and W. Tan, Chem. Soc. Rev., 2018, 47, 7140–7180 RSC.
- Z. Lei, C. Sun, P. Pei, S. Wang, D. Li, X. Zhang and F. Zhang, Angew. Chem., Int. Ed., 2019, 58, 8166–8171 CrossRef CAS PubMed.
- X. He, L. Li, Y. Fang, W. Shi, X. Li and H. Ma, Chem. Sci., 2017, 8, 3479–3483 RSC.
- D. Cheng, J. Peng, Y. Lv, D. Su, D. Liu, M. Chen, L. Yuan and X. Zhang, J. Am. Chem. Soc., 2019, 141, 6352–6361 CrossRef CAS PubMed.
- T. Wang, Q. Sun, H. Xiong, C. Ma, C. Lu, J. Nie, G. Yang, Z. Chen, Y. Zhang, J. Ren, F. Wang and W.-H. Zhu, Sens. Actuators, B, 2020, 321, 128631 CrossRef CAS.
- Z. Zhou, F. Wang, G. Yang, C. Lu, J. Nie, Z. Chen, J. Ren, Q. Sun, C. Zhao and W. H. Zhu, Anal. Chem., 2017, 89, 11576–11582 CrossRef CAS PubMed.
- R. Yan, Y. Hu, F. Liu, S. Wei, D. Fang, A. J. Shuhendler, H. Liu, H. Y. Chen and D. Ye, J. Am. Chem. Soc., 2019, 141, 10331–10341 CrossRef CAS PubMed.
- Z. Huang, R. An, S. Wei, J. Wang and D. Ye, Analyst, 2021, 146, 1865–1871 RSC.
- Y. Wu, S. Huang, J. Wang, L. Sun, F. Zeng and S. Wu, Nat. Commun., 2018, 9, 3983 CrossRef PubMed.
- D.-Q. Wu, B. Lu, C. Chang, C.-S. Chen, T. Wang, Y.-Y. Zhang, S.-X. Cheng, X.-J. Jiang, X.-Z. Zhang and R.-X. Zhuo, Biomaterials, 2009, 30, 1363–1371 CrossRef CAS PubMed.
- X. Chen, Y. Zhang, Q. Yuan, M. Li, Y. Bian, D. Su and X. Gao, J. Mater. Chem. B, 2021, 9, 6614–6622 RSC.
- P. Gao, W. Pan, N. Li and B. Tang, Chem. Sci., 2019, 10, 6035–6071 RSC.
- N. Choi, S.-M. Kim, K. S. Hong, G. Cho, J.-H. Cho, C. Lee and E. K. Ryu, Biomaterials, 2011, 32, 7151–7158 CrossRef CAS PubMed.
- H. Wang, C. Liu, Z. He, P. Li, W. Zhang, W. Zhang and B. Tang, Anal. Chem., 2021, 93, 6551–6558 CrossRef CAS PubMed.
- M. Watanabe, S. M. Houten, C. Mataki, M. A. Christoffolete, B. W. Kim, H. Sato, N. Messaddeq, J. W. Harney, O. Ezaki, T. Kodama, K. Schoonjans, A. C. Bianco and J. Auwerx, Nature, 2006, 439, 484–489 CrossRef CAS PubMed.
- G. A. Kullak-Ublick, B. Stieger and P. J. Meier, Gastroenterology, 2004, 126, 322–342 CrossRef CAS PubMed.
- B. Hagenbuch and C. Gui, Xenobiotica, 2008, 38, 778–801 CrossRef CAS PubMed.
- D. Q. Chen, X. Wang, L. Chen, J. X. He, Z. H. Miao and J. K. Shen, Acta Pharmacol. Sin., 2011, 32, 664–672 CrossRef CAS PubMed.
- F. Holzinger, C. D. Schteingart, H.-T. Ton-Nu, C. Cerrè, J. H. Steinbach, H.-Z. Yeh and A. F. Hofmann, Hepatology, 1998, 28, 510–520 CrossRef CAS PubMed.
- Y. Wu, L. Sun, F. Zeng and S. Wu, Photoacoustics, 2019, 13, 6–17 CrossRef PubMed.
- Z. Luo, Z. Huang, K. Li, Y. Sun, J. Lin, D. Ye and H.-Y. Chen, Anal. Chem., 2018, 90, 2875–2883 CrossRef CAS PubMed.
- R. Yang, S. Zhang, A. Cotoia, N. Oksala, S. Zhu and J. Tenhunen, BMC Gastroenterol., 2012, 12, 45 CrossRef CAS PubMed.
- A. Ramachandran, M. R. McGill, Y. Xie, H.-M. Ni, W.-X. Ding and H. Jaeschke, Hepatology, 2013, 58, 2099–2108 CrossRef CAS PubMed.
- J. Ghosh, J. Das, P. Manna and P. C. Sil, Free Radic. Biol. Med., 2010, 48, 535–553 CrossRef CAS PubMed.
- D. Cheng, W. Xu, L. Yuan and X. Zhang, Anal. Chem., 2017, 89, 7693–7700 CrossRef CAS PubMed.
- X. Jie, M. Wu, H. Yang and W. Wei, Anal. Chem., 2019, 91, 13174–13182 CrossRef CAS PubMed.
- H. Umezawa, M. Ishizuka, T. Aoyagi and T. Takeuchi, J. Antibiot., 1976, 29, 857–859 CrossRef CAS PubMed.
- A. J. Hanley, K. Williams, A. Festa, L. E. Wagenknecht, R. B. D'Agostino, J. Kempf, B. Zinman and S. M. Haffner, Diabetes, 2004, 53, 2623–2632 CrossRef CAS.
- G. Abboud and N. Kaplowitz, Drug Saf., 2007, 30, 277–294 CrossRef CAS PubMed.
- P. Cheng, Q. Miao, J. Li, J. Huang, C. Xie and K. Pu, J. Am. Chem. Soc., 2019, 141, 10581–10584 CrossRef CAS PubMed.
- W. Zhang, L. Chen, H. Feng, W. Wang, Y. Cai, F. Qi, X. Tao, J. Liu, Y. Shen, X. Ren, X. Chen, J. Xu and Y. Shen, Free Radic. Biol. Med., 2017, 112, 24–35 CrossRef CAS PubMed.
- J. H. Kim, W. S. Nam, S. J. Kim, O. K. Kwon, E. J. Seung, J. J. Jo, R. Shresha, T. H. Lee, T. W. Jeon, S. H. Ki, H. S. Lee and S. Lee, Int. J. Mol. Sci., 2017, 18, 1417 CrossRef.
- P. Wang, Y. Yang, G. Pang, C. Zhang, C. Wei, X. Tao, J. Liu, J. Xu, W. Zhang and Y. Shen, Free Radic. Biol. Med., 2021, 162, 283–297 CrossRef CAS PubMed.
Footnote |
† Electronic supplementary information (ESI) available: Experimental details, synthesis and characterization data, and additional spectra and imaging data. See DOI: 10.1039/d1sc04825b |
|
This journal is © The Royal Society of Chemistry 2021 |