DOI:
10.1039/D1SC03516A
(Perspective)
Chem. Sci., 2021,
12, 12234-12247
Cancer therapeutic strategies based on metal ions
Received
28th June 2021
, Accepted 1st September 2021
First published on 2nd September 2021
Abstract
As a necessary substance to maintain the body's normal life activities, metal ions are ubiquitous in organisms and play a major role in various complex physiological and biochemical processes, such as material transportation, energy conversion, information transmission, metabolic regulation, etc. Their abnormal distribution/accumulation in cells can interfere with these processes, causing irreversible physical damage to cells or activating biochemical reactions to induce cell death. Therefore, metal ions can be exploited against a wide spectrum of cancers with high efficiency and without drug resistance, which can effectively inhibit the growth of cancer cells by triggering biocatalysis, breaking the osmotic balance, affecting metabolism, interfering with signal transduction, damaging DNA, etc. This perspective systematically summarizes the latest research progress of metal ion-based anti-tumor therapy, and emphasizes the challenges and development directions of this type of therapeutic strategy, hoping to provide a general implication for future research.
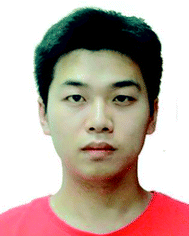 Yang Liu | Yang Liu received his BSc degree in material chemistry from Heilongjiang University in 2016. Afterwards, he joined the group of Prof. Hongjie Zhang at Changchun Institute of Applied Chemistry, Chinese Academy of Sciences (CAS) as a PhD candidate. Currently, his research interests focus on design and preparation of multifunctional stimuli-responsive nanomaterials for effective tumor diagnosis and therapy. |
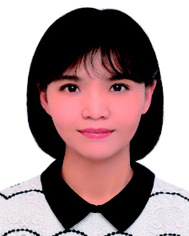 Yinghui Wang | Yinghui Wang received her PhD degree in condensed matter physics from Changchun Institute of Optics, Fine Mechanics and Physics, Chinese Academy of Sciences in 2011. Then, she worked as a postdoctoral researcher at University of Amsterdam (Netherlands) and Changchun Institute of Applied Chemistry, Chinese Academy of Sciences (CAS). She is working as associate professor at Changchun Institute of Applied Chemistry, CAS. Her research interests focus on design and synthesis the multifunctional upconversion nanoparticles and their bioapplications. |
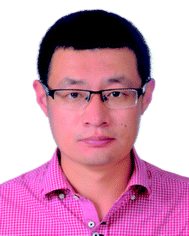 Shuyan Song | Shuyan Song received his BSc degree in Chemistry in 2003 and MSc degree in Inorganic Chemistry in 2006, both from Northeast Normal University. He joined the group of Prof. Hongjie Zhang at Changchun Institute of Applied Chemistry, Chinese Academy of Sciences (CAS), where he received his PhD degree in Inorganic Chemistry in 2009. He is working as a full professor at Changchun Institute of Applied Chemistry, CAS. His research focus is primarily on the development of functional rare-earth materials for catalysis, chemical sensing and photoluminescence. |
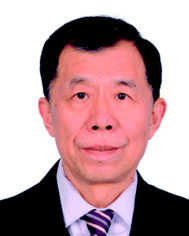 Hongjie Zhang | Hongjie Zhang received his BS (1978) from Peking University and MS (1985) from Changchun Institute of Applied Chemistry (CAS). Then, he worked as an assistant professor at the same institute from 1985–1989. He then studied at University of Bordeaux I (France), where he received his PhD degree in 1993. He joined Changchun Institute of Applied Chemistry (CAS), as a professor in 1994. He was elected as an academician of the Chinese Academy of Science in 2013, and a member of The World Academy of Science (TWAS) in 2015. His current research interests include synthesis and application of lanthanide functional materials. |
1. Introduction
Metal ions have a very important impact on life systems, and they play a necessary role that other chemical molecules cannot replace.1 Some of them are used as signal factors and co-catalytic factors for biological macromolecules, and some are important structural components of biological macromolecules, participating in a variety of chemical reactions and signal transduction in life systems and other important physiological activities.2 The various properties of cells will be affected by the types and distribution of metal ions. Excess, deficiency, and abnormal distribution of metal ions will seriously affect various physiological properties of cells. Therefore, stimulating tumor cells with exogenous metal ions or interfering with the function of their endogenous metal ions can effectively affect the activity of cancer cells. Compared with traditional chemotherapy drugs, most metal ions can be exploited against a wide spectrum of tumors with high efficacy and without drug resistance.3 Moreover, most metal ion therapy does not depend on external energy, and can effectively avoid radiation damage caused by therapies such as radiotherapy.4 However, tumor cells can adjust the abnormal distribution of ions through a self-regulating mechanism to maintain their physiological homeostasis. Therefore, how to achieve effective ion enrichment in tumor cells is a key problem of exogenous metal ion-based therapy.
The emergence of nanotechnology has brought great hopes for this. Unlike ions and small molecules, nanomaterials can enter the cell through special methods, such as endocytosis and large pinocytosis.5 In response to specific stimuli, they can quickly release excess metal ions that exceed the self-regulation threshold, causing sudden changes in the distribution of intracellular and extracellular ions, imbalances in osmolarity and redox, and inactivation of functional proteins. Additionally, interfering with endogenous metal ions is also an effective strategy, which not only can trigger cell oxidative stress, but also further affects cell metabolism. For example, using the intracellular labile iron pool to trigger biocatalysis,6 or interfering with mitochondrial copper to affect cell metabolism.7,8 Therefore, these nanomedicines that contain metal ions or can regulate the function of endogenous metal ions greatly promote the therapeutic effect of metal-ion therapy and make it show good application potential.
This perspective article will start from the regulation of endogenous and exogenous ions, and systematically summarize the latest research progress and development trends of anti-tumor therapy based on metal ions, specifically including the following five aspects (Fig. 1): (1) metal ion therapeutic strategy based on biocatalysis; (2) metal ion therapeutic strategy based on osmolarity; (3) metal ion therapeutic strategy based on metabolism; (4) metal ion therapeutic strategy based on signal transduction; and (5) metal ion therapeutic strategy based on cell replication and transcription.
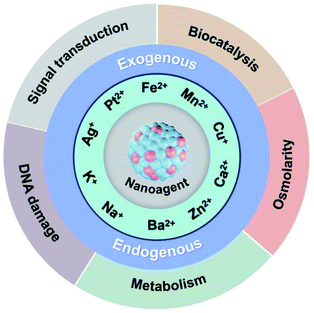 |
| Fig. 1 A schematic overview of metal ion-based therapeutic strategy. | |
2. Metal ion therapeutic strategy based on biocatalysis
With the increasing development of nanotechnology, some nanocatalysts based on metal ions have been successively used in the field of biomedicine, which can change the chemical microenvironment by triggering the specific catalytic reaction in the lesion site, thereby realizing effective treatment of some major diseases, such as cancer, Alzheimer's disease, myocardial infarction, stroke, and wound infection, etc.9–13 Biocatalysis is mainly attributed to the redox ability of nanomaterials or metal ions under external physical or chemical stimuli.9 By generating or eliminating reactive oxygen species in specific physiological areas, it can achieve local regulation of oxidative stress, and ultimately realize the regulation of physiological functions of cancer cells or normal cells. At present, biocatalytic treatment based on metal ions is mainly achieved through the following two ways: (1) delivery of exogenous metal ions to specifically trigger the therapeutic effect at the tumor site; (2) regulation of endogenous metal ions at the tumor site to achieve catalytic therapy.
2.1 Exogenous ion biocatalysis
Compared with normal tissues, solid tumors have a specific tumor microenvironment (TME), such as hypoxia, weak acidity, excessive hydrogen peroxide (H2O2) and glutathione (GSH), etc.14 Therefore, TME not only provides a suitable environment and nutrition for tumor development and metastasis, but also provides a “gate” for selective and effective cancer therapy.15 The use of biocatalysts to in situ generate toxic molecules in tumors through biocatalysis may be an ideal way for highly specific and selective tumor treatment. Although H2O2 is a strong oxidant, it is not very reactive with most biomolecules. However, H2O2 can be activated by many catalysts to become more reactive oxidants, leading to severe oxidative stress and cell death. Fe2+, Cu+, Mn2+, and other transition metal ions can catalyze the excessive low oxidizing H2O2 in the TME to highly oxidizing and toxic hydroxyl radicals (˙OH) through Fenton or Fenton-like reaction, which can disturb the intracellular redox balances state, eventually leading to cell apoptosis.16–19
As an exogenous chemical-stimuli-driven therapeutic modality, chemodynamic therapy (CDT) based on transition metal ions confers cancer treatment with high specificity, efficacy, and biosafety.20–22 Currently, a large number of nanomaterials based on transition metal ions have been reported for CDT, such as Fe(0), Fe3O4, CuFe2O4, Fe2O3, FeS2, CuO2, Cu-Cys NPs, and MnO2, et al.23–30 Our research group synthesized one-dimensional ferrous phosphide (Fe2P) nanorods (FP NRs) by a high-temperature method and further modified them by PTMP-PMAA to improve their hydrophilicity and biocompatibility.31 FP NRs can be ionized into Fe2+ in an acidic microenvironment, and then catalyze H2O2 to generate highly toxic ˙OH, realizing Fe2+ ion interference anti-tumor therapy. Moreover, this Fe2+-based CDT effect can be significantly enhanced under photothermal and ultrasound stimulation (Fig. 2a–d). Under the laser irradiation, the reaction rate of the Fenton reaction and ionization process could be promoted as the temperature rises. And under the ultrasound irradiation, the conversion rate of Fe3+ to Fe2+ could be accelerated. Although Fe2+-based metal ion therapy strategies have been extensively studied, the amount of generated ROS is not enough to effectively kill the cancer cells since they need much lower pH conditions (pH = 3–4) than that in TME (pH = 6.5–6.9).32 Therefore, the main challenge for the future development of iron-based ion therapy is to improve the biocatalytic activity of iron-based nanomaterials. In addition, Overholtzer's group demonstrated for the first time that iron-containing nanoparticles can kill cancer cells through ferroptotic pathway.33,34 Ferroptosis is an iron-dependent programmed cell death that is different from apoptosis, cell necrosis, and autophagy. It is closely related to oxidative stress, cystine metabolism, and accumulation of lipid peroxidation products.35 It has also been reported that iron oxide nanoparticles can inhibit tumor growth by inducing pro-inflammatory macrophage polarization in tumour tissues.36
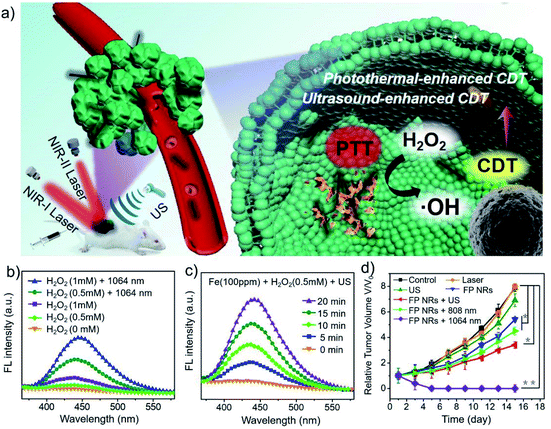 |
| Fig. 2 (a) Schematic illustration of the FP NRs for multimode imaging-guided PTT and ultrasound/PT-enhanced CDT. (b and c) Photothermal and ultrasound enhanced Fenton properties. (d) Tumor volume curves of mice after different treatments. Reproduced with permission from ref. 31. Copyright 2019, John Wiley and Sons. | |
Because the biocatalytic efficiency of Fe-based nanomaterials is limited by the weakly acidic TME, our group further developed a metal ion therapy strategy based on Cu+. We reported a cuprous phosphide (Cu3P) Fenton-like agent (CP NCs) with the synergistic therapeutic effect of CDT and photothermal therapy.37 Similar to Fe2+-based biocatalysis, CP NCs can be ionized into Cu+ in an acidic TME, and then catalyze H2O2 to generate ˙OH, realizing Cu+ ion interference anti-tumor therapy. The Cu+-based catalytic reaction is faster than the Fe2+-based catalytic reaction, and it is more suitable for the actual pH value of the weakly acidic TME. Moreover, the efficiency of the Fenton-like reaction will be further enhanced as the temperature rises. In addition, excessive GSH in TME will further reduce Cu2+ produced by Cu-based Fenton-like reaction to Cu+, further enhancing the Cu+-based CDT therapeutic effect. Recently, Li's group has constructed a variety of multifunctional metal–DNA hybrid nanospheres through the coordination-driven self-assembly strategy, such as Fe–DNA nanospheres and Cu–DNAzyme nanohybrids.38,39 Cu–DNAzyme nanohybrids can effectively transport DNAzyme and Cu2+ into cancer cells for synergistic catalytic therapy. The released Cu2+ can be reduced to Cu+ and catalyze endogenous H2O2 to produce ˙OH, while DNAzyme can cut vascular endothelial growth factor receptor 2 (VEGFR2) mRNA and activate gene silencing for gene therapy.39 Although Cu-based nanomaterials have a good therapeutic effect on tumors, their high biocatalytic properties also bring non-negligible toxicity to normal cells. Therefore, the simultaneous consideration of biosafety and high biocatalytic properties is of great significance for the further development of Cu-based nanoagents. In addition, Chen's group reported that manganese dioxide (MnO2) has Fenton-like and GSH depletion properties, and can be used as an intelligent CDT agent for Mn2+-based metal ion therapy (Fig. 3a–c).30 This work not only shows that multifunctional MnO2 with enhanced biocatalytic properties has great potential in cancer diagnosis and therapy, but also provides an example for the design of metal-based nanoagents with the ability to consume intracellular GSH.
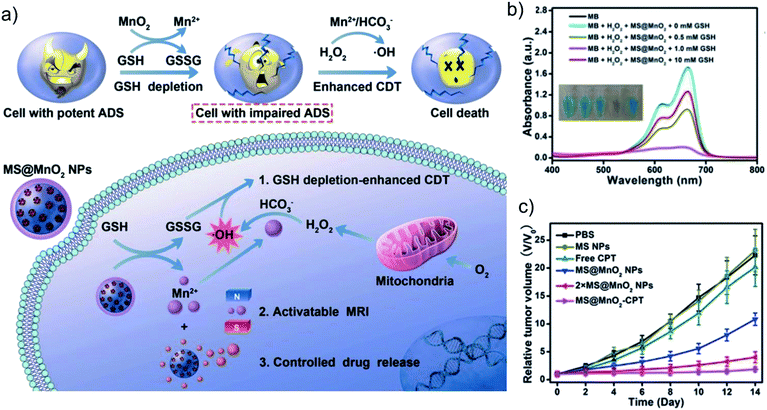 |
| Fig. 3 (a) Schematic illustration of the MnO2-coated mesoporous silica nanoparticles (MS@MnO2 NPs) for GSH-depletion enhanced CDT. (b) The Fenton-like properties of MS@MnO2 NPs under different concentrations of GSH. (c) Tumor volume curves of mice after different treatments. Reproduced with permission from ref. 30. Copyright 2018, John Wiley and Sons. | |
These metal ion-based nanomaterials that can generate ROS through biocatalysis to kill tumor cells provide a highly specific and efficient treatment strategy for cancer therapy, which greatly promotes the development of inorganic nanomedicine in the field of biomedicine. However, most metal ion-based nanomaterials are metal compounds and nanoagents loaded with metal ions. Most metal compounds are relatively stable, and their biocatalytic effects are not satisfactory due to their limited ion release and fewer catalytic sites on the surface. Moreover, the biological environment is complex and very different from the experimental environment, which leads to the use of in vivo biocatalysis may not be able to achieve the expected precise and efficient therapy. In addition, although various investigations have been conducted to reveal the long-term fate of these inorganic nanocatalysts, the partial biodegradation of transition metal ions may lead to potential metal homeostasis and related side effects. Therefore, how to suppress the unfavorable catalytic process to reduce the side effects remains to be extensively studied.
2.2 Endogenous ion biocatalysis
Iron is the most abundant transition metal in biological systems and plays a vital role in many cell metabolism processes.40 Especially, a small part of free or loosely bound intracellular Fe2+, called labile iron pool (LIP), has redox activity and can participate in Fenton-type reactions in cells to produce ROS.41,42 Since the LIP level of cancer cells is higher than that of normal cells, the intracellular LIP of tumor cells can be used as a source of Fe2+ for Fenton reaction. Based on this, Chen's group reported a new CDT method that generates free radicals mediated by LIP.6 By simulating the metabolic processes of intracellular peroxygen compounds (H2O2, peroxides (R′OOR′), hydroperoxides (R′OOH), and endoperoxides) (Fig. 4a), they found that the reaction of R′OOH with LIP can generate more free radicals than other molecules. Therefore, they prepared a pH-responsive polymer cascade reaction nanomedicine containing encapsulated linoleic acid methyl hydroperoxide and LIP enhancer to achieve efficient CDT (Fig. 4b–d). This work not only paved the way for the use of endogenous metal ions for cancer treatment, but also provided a model for exploring CDT agents activated by LIP. However, since LIP only accounts for a small part (<5%) of the total cell iron (20–100 μM),43 the biocatalysis induced by LIP may not be enough to cure tumors, and combination with other treatment strategies may be an effective way to improve the effect of ion therapy.
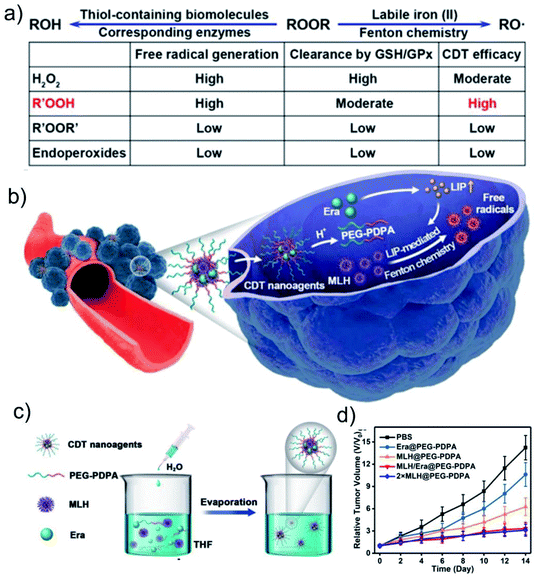 |
| Fig. 4 Schematic illumination of (a) factors influencing LIP-mediated CDT efficacy of ROOR and (b) methyl linoleate hydroperoxide (MLH) and erastin (Era)-loaded poly(ethyleneglycol)-block-poly(diisopropylaminoethyl methacrylate) (PEG-PDPA) nanoagents (MLH/Era-loaded PEG-PDPA NPs) for enhanced CDT. (c) Schematic illustration of the synthesis of MLH/Era-loaded PEG-PDPA NPs nanoagents. (d) Tumor volume curves of mice after different treatments. Reproduced with permission from ref. 6. Copyright 2020, American Chemical Society. | |
3. Metal ion therapeutic strategy based on osmolarity
The asymmetric ion gradients inside and outside mammalian cells are critical to cell function and drive basic cellular processes.44 If the concentration of extracellular Na+ or K+ is reduced, it will cause damage to the cytoskeleton, cell cycle arrest, and cell lysis. Elevating intracellular osmotic pressure can also cause similar effects.45,46 Therefore, changing the intracellular and extracellular ion concentration of tumors is an effective strategy to kill tumors. However, owning to self-regulation mechanisms of tumors, this seemingly simple idea is difficult to achieve. The emergence of nanotechnology has brought great hopes for this. Unlike ions and small molecules, nanomaterials can enter the cell through special methods such as endocytosis and large pinocytosis without being detected through the relevant ion pumps/channels.5 Based on this, Xie's group successfully prepared relatively stable NaCl nanoparticles (SCNP), which can directly enter tumor cells through endocytosis.47 Then, SCNP will dissolve in tumor cells to release a large amount of Na+, and these ions will be restricted inside the cell owing to the reverse osmotic gradient across the plasma membrane, ultimately resulting in effective suppression of cancer cells. In addition, this treatment can also trigger immunogenic cell death (ICD) and activate the anti-tumor immune response. Similarly, our group reported phospholipid-coated Na2S2O8 nanoparticles (PNSO NPs) as a new type of Na+ delivery agent and ROS generation agent (Fig. 5a–d).48 PNSO NPs could be degraded into Na+ and S2O82− in cancer cells, and then S2O82− will be further converted into toxic ˙SO4− and ˙OH. Meanwhile, PNSO NPs can send a large amount of Na+ into cells through endocytosis, causing a surge of osmotic pressure and rapid cell rupture. Moreover, PNSO NPs also can activate the systemic anti-tumor immune response, effectively inhibiting tumor metastasis and recurrence. Therefore, transporting a large amount of Na+ and K+ through nanomaterials is an effective strategy to break the ion balance of cells. Interference with ion balance can effectively kill cancer cells without causing systemic side effects and drug accumulation. However, due to the relatively short half-life of NaCl and PNSO NPs in aqueous solutions, the current form of these nanoagents are not suitable for systemic cancer treatment. Therefore, improving the stability and specificity of this type of nanomaterials is the main challenge before its clinical application.
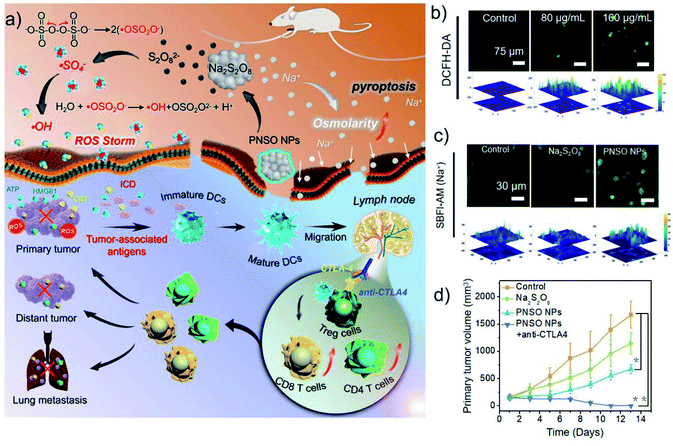 |
| Fig. 5 (a) Illustration of therapeutic mechanism of PNSO NPs. (b) The ability of PNSO NPs to generate ROS at the cellular level. (c) Increased intracellular Na+ concentration caused by PNSO NPs. (d) Tumor volume curves of mice after different treatments. Reproduced with permission from ref. 48. Copyright 2020, American Chemical Society. | |
In addition to transporting metal ions into cells through nanocarriers to cause cell osmotic pressure change, interference with ion channels by drugs can also affect the cell osmotic homeostasis. Ion channels are pathways for passive transport of various inorganic ions across membranes, and they are also involved in the malignant phenotype of cancer cells.49 In many tumor types, K+ channels exhibit high variability and frequent expression changes, so K+ channels can be used as potential targets for cancer treatment.50 It is reported that barium ions (Ba2+) can prevent the outflow of K+ by strongly binding to K+ channels, thereby affecting cell membrane potential and osmotic balance, and ultimately inducing cell proliferation and death.51 Therefore, Ba2+-based nanoagents have great potential in metal ion-based anti-tumor therapy. However, its acute and chronic toxicity caused by its common channel targeting in normal tissues should be concerned. Based on this, Bu's group designed chelator-modified barium peroxide nanoparticles (GL-BaO2NPs), which can achieve controllable cancer cell killing ability with the assistance of X-ray (Fig. 6a–c).52 The modification of the N,N-bis(carboxymethyl)-L-glutamic acid tetrasodium salt (GLDA) chelator can strongly bind to the leaked free Ba2+, thereby alleviate its side effects in vivo. However, ˙OH produced by BaO2 NPs under X-ray irradiation can destroy the structure of chelators and achieve effective release of Ba2+. The released Ba2+ will bind to K+ channels to further affect the cell membrane potential and osmotic homeostasis, and forming a good synergistic promotion effect with radiotherapy. This work achieved precise and effective control of the biological properties of exogenous metal ions through external stimulation, and provided a safe and referential strategy for the anti-tumor application of exogenous ions.
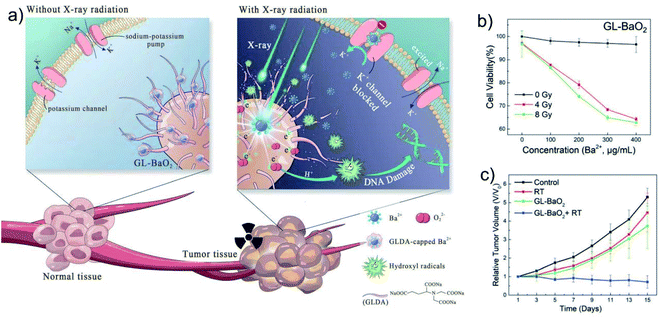 |
| Fig. 6 (a) Schematic representation of the radiation-assisted metal ion therapy by GL-BaO2 NPs. (b) Cell viability detection under different concentrations of GL-BaO2 NPs and different radiation doses. (c) Tumor volume curves of mice after different treatments. Reprinted with permission from ref. 52. Copyright 2019, The Royal Society of Chemistry. | |
4. Metal ion therapeutic strategy based on metabolism
Copper is a transition metal with redox activity, which is essential for most organisms.53 As a catalytic cofactor of enzymes, copper plays a vital role in antioxidant defense, iron homeostasis, cell respiration, and various biochemical processes.54 Many human tissue abnormalities and diseases are related to the decrease or increase of copper levels. Therefore, all life forms can maintain copper homeostasis through a conserved and exquisite copper metabolic systems.55 In many tumor tissues and serum, the concentration of copper is higher than that of normal tissues. Especially in breast cancer patients, the mitochondrial copper chaperone and cochaperone proteins COX17 and SCO2 were up-regulated, showing that breast cancer cells have higher requirements for copper transport to mitochondria.56,57 The mitochondrial copper enzyme cytochrome c oxidase (COX) is a complex IV of the electron transport chain,58,59 which mainly matures in the mitochondrial membrane space, and its activity is affected by the supply of mitochondrial copper.60 Therefore, limiting the supply of copper can effectively affect the metabolism of cancer cells that require a large amount of copper. Rao's group developed a copper-depleting nanoparticle (CDN) to consume the copper in tumors with minimal side effects on healthy tissues.61 CDN consists of two parts: a copper depletion part (CDM) and a semiconducting polymer nanoparticle (SPN). The positive charge on the surface of CDN facilitates its accumulation in mitochondria and further depletes mitochondrial copper. After treatment with CDN, the metabolism of triple negative breast cancer (TNBC) cells changes from mitochondrial oxidative phosphorylation (OXPHOS) to glycolysis, which in turn reduces the production of adenosine triphosphate (ATP). Under the combined action of metabolic remodeling, damaged mitochondrial membrane potential, and increased oxidative stress, TNBC cells will be effectively killed. Although most tumor cells obtain energy through glycolytic metabolism, it is increasingly recognized that certain cancer cell types depend on mitochondrial OXPHOS, including breast cancer, metastatic ovarian cancer, prostate cancer, and glioblastoma.62–66 Fatty acids ingested from adjacent adipocytes are their main source of energy. Mitochondrial copper consumption can reshape cell metabolism and reduce energy production, which is effective for cancer types that rely on OXPHOS. At present, most of the metal ion therapy strategies that regulate metabolism by interfering with endogenous ions use ion chelators. However, most of these ion chelators have poor selectivity and will inevitably interfere with the metabolism of normal cells when treating tumor cells, resulting in unavoidable biological toxicity. Therefore, the targeted transport and specific release of ion chelators should receive further attention.
5. Metal ion therapeutic strategy based on signal transduction
Cells are the basic structure and functional unit of a living body, which receive various signals from inside and outside the cell at all times. Cell signal transduction is a basic and important way of life activities. Ca2+ is an important intracellular messenger that exists widely in cells.67–70 Almost all the physiological activities of cells are regulated by Ca2+, such as the contraction of muscle cells, the release of neurotransmitters from neurons and astrocytes, the activation of fertilized eggs, the healing and metabolic processes of liver and pancreas wounds, and cellular maturity, differentiation, death, etc.71–74 In addition, Ca2+ also has significant regulatory effects on tumor growth, development, invasion, and metastasis.75 Under normal circumstances, cells have an extremely strict regulation mechanism on the concentration of Ca2+. However, under oxidative stress, due to the abnormal function of cellular calcium channels, it is difficult for cells to regulate Ca2+ homeostasis, resulting in calcium-overload-induced cell death.76–78 Therefore, destruction of tumor cellular Ca2+ homeostasis through calcium overload is expected to be one of the potential means for anti-tumor therapy.
Similar to Ca2+, zinc element also can act as a signal molecule in the cell in the form of free ions and plays an important regulatory role in cell signal transduction through the influence of multiple key links, such as extracellular signal recognition, second messenger metabolism, protein kinase, phosphatase activity, and transcription factor activity.79–82 It plays an important role in a variety of physiological and pathological events, including cellular immunity, proliferation, apoptosis, differentiation, and invasion. Zn2+ has the function of regulating many ion channels, such as L-type Ca2+ channel and K+ channels, and Zn2+ as a second messenger also can cross-communicate with Ca2+ signaling pathways.83–86 Pan's group found that Zn2+ can selectively prevent the growth of esophageal cancer cells without affecting normal esophageal epithelial cells.87 They revealed for the first time the anti-cancer mechanism of Zn2+: Zn2+ can inhibit the over-activated Ca2+ signal in tumor cells, while normal cells do not show over-activated Ca2+ signals. In addition, destroying intracellular Zn2+ homeostasis is also an effective way to kill tumor cells.88 A variety of Zn-based nanomaterials that can release Zn2+ at tumor sites have been developed for tumor therapy, such as ZnO, ZnO2, and etc.89,90 The release of excessive Zn2+ can induce the depolarization of mitochondrial membrane potential, activate caspase-3, and lead to cell apoptosis and lactic dehydrogenase release. At the same time, Zn2+ can also enhance the generation of endogenous ROS by inhibiting the mitochondrial electron transport chain.88,90
Therefore, the design and preparation of degradable nanoparticles containing Ca2+ or Zn2+ are of great significance for Ca2+ or Zn2+-based metal ion anti-tumor therapy. These nanomaterials can transport ions into cells through endocytosis, and further release large amounts of free ions through degradation. The excessive amount of messenger ions in the cell will immediately activate the intracellular storage mechanism, significantly enhance mitochondrial respiration, increase the production of free radicals, cause oxidative damage to DNA and functional proteins, and ultimately lead to irreversible death of cancer cells. Bu's group cleverly used ultra-small calcium peroxide (CaO2) to activate tumor cells to produce a unique “calcium overload” biological effect, and for the first time proposed a new type of tumor treatment strategy based on the “calcium death” of tumor cells: ion interference therapy (IIT) (Fig. 7a–c).91 CaO2 can quickly decompose to produce H2O2 and free Ca2+ in the acidic TME. Due to the down-regulation of catalase expression in tumor cells, the H2O2 produced by the decomposition of CaO2 accumulates in tumor cells in large quantities, which induces tumor cells to produce oxidative stress. Oxidative stress further leads to the dysfunction of Ca-related ion channels, thereby causing Ca2+ overload in tumor cells. Ca2+ overload further induces metabolic and functional disorders of tumor cells, thereby inducing cell death. In addition, Dong's group reported a nanoscale covalent organic framework (COF)-based nanoagent, namely CaCO3@COF-BODIPY-2I@GAG (boron dipyrromethene-2I (BODIPY-2I) as photosensitizer and glycosaminoglycan (GAG) as targeting agent).92 This nanomedicine shows a good therapeutic effect on colon tumors through the synergistic treatment of photodynamic therapy (PDT) and 1O2-triggered Ca2+ overload (Fig. 8a–e). Although it can effectively inhibit the growth of cancer cells by interfering with the intracellular Ca2+ or Zn2+ homeostasis, the poor stability of Ca/Zn-based nanomedicines such as CaO2, CaCO3, ZnO in the physiological environment leads to the premature release of Ca2+ or Zn2+, which makes the therapeutic effect unsatisfactory. Moreover, these nanomaterials lack specificity and targeting in the regulation of ions homeostasis, and their impact on normal cells need to be further investigated.
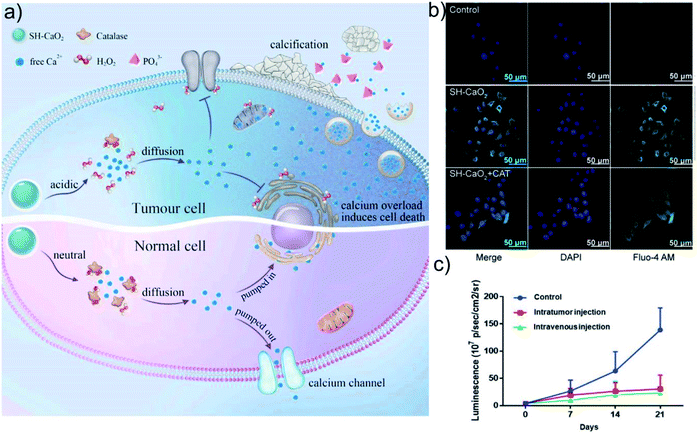 |
| Fig. 7 (a) Schematic illustration of SH-CaO2 NPs for Ca2+ overload therapy. (b) Increased intracellular Ca2+ concentration caused by SH-CaO2. (c) Tumor volume curves of mice after different treatments. Reprinted with permission from ref. 91. Copyright 2019, Elsevier. | |
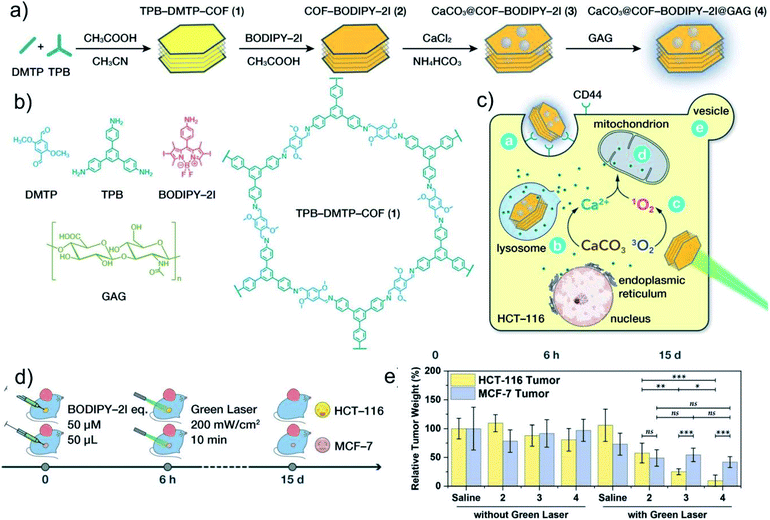 |
| Fig. 8 (a) Synthesis process of CaCO3@COF-BODIPY-2I@GAG. (b) Molecular structure of 2,5-dimethoxyterephthalaldehyde (DMTP), 1,3,5-tris(4-aminophenyl)benzene (TPB), BODIPY-2I, GAG, and TPB-DMTPCOF. (c) Schematic illustration of CaCO3@COF-BODIPY-2I@GAG for PDT and intracellular Ca2+ overload therapy. (d) Schematic diagram of in vivo treatment strategy. (e) Tumor weight of mice after various treatments. Reprinted with permission from ref. 92. Copyright 2020, John Wiley and Sons. | |
6. Metal ion therapeutic strategy based on DNA damage
Metal drugs show excellent performance in the diagnosis and treatment of diseases and play an indispensable role in the stage of drug development. Among them, as the most widely used metal anti-tumor chemotherapy drugs, platinum (Pt)-containing drugs represent one of the major achievements in the field of medicinal inorganic chemistry.93 Pt-containing drugs are cell cycle non-specific drugs, including cisplatin, carboplatin, oxaliplatin, etc., which are currently widely used in diseases, such as gynecological and digestive system tumors.94–96 Such drugs can enter the cell nucleus and act on DNA molecules to form Pt–DNA compounds, which can deform the DNA structure and hinder its replication and transcription.97 However, Pt-containing anti-tumor drugs have disadvantages, such as high system toxicity, easy induction of cell resistance, low selectivity to tumor tissues, and low bioavailability.98 For example, the combination of Pt drugs and proteins in the blood will cause most drugs to be inactivated before reaching the lesion, which not only causes some toxic side effects, but also reduces the bioavailability.99 In addition, traditional Pt drugs mainly target DNA, but tumor cells have the ability to repair DNA damage, so cancer cells are prone to developing resistance to Pt drugs.100 Designing Pt complexes as Pt(IV) prodrugs is an effective way to overcome the side effects of cisplatin drugs. Compared with Pt(II) anti-tumor drugs, Pt(IV) complexes are more stable due to their kinetic inertia.101 Moreover, the two additional ligands introduced by the octahedral metal center provide many possibilities for improving pharmacokinetic parameters, including enhancing molecular targeting.102 Since Pt(IV) must be reduced to Pt(II) in the cell to exhibit cytotoxicity, the Pt(IV)-based prodrug provides a better method for tumor-targeted delivery of cisplatin or its analogs. Based on this, Lin's group developed a multifunctional nanoplatform based on Pt(IV) prodrug loaded NaYF4:Yb,Tm upconversion nanoparticles (UCNPs), followed by coating a layer of dimethyl-maleic acid (DMMA) and mPEG-COOH modified poly-(allylamine) (PEG–PAH–DMMA) polymer (UCNs-Pt(IV)@PEG–PAH–DMMA).103 The ultraviolet light emitted by UCNPs under near-infrared (NIR) light irradiation and the GSH in TME can effectively activate Pt(IV) prodrugs into highly cytotoxic Pt(II), realizing efficient chemotherapy (Fig. 9a–e). In addition, disguising the cytotoxic Pt(II) ions into inert Pt(0) atoms is also an effective strategy to improve the selectivity and biocompatibility of Pt-containing drugs. Helma Wennemers's group developed peptide-stabilized platinum nanoparticles (PtNPs), which showed significantly greater cytotoxicity to liver cancer cells (HepG2) than to other cancer cells and normal cells.104 This is mainly because the high oxidation environment of HepG2 cells can oxidize inert Pt(0) into cytotoxic Pt(II), which in turn triggers the effective treatment of cancer cells by Pt nanoagent. In short, Pt prodrugs that can specifically produce toxicity at the tumor site not only effectively reduce the biological toxicity of Pt drugs, but the discovery of some new targets also improves their drug resistance. However, some Pt drugs still have shortcomings such as narrow anti-tumor spectrum, short duration, cross-resistance, and side effects. Therefore, the design and synthesis of high-efficiency, broad-spectrum, low-toxicity, and long-lasting Pt drugs is still the direction of current efforts.
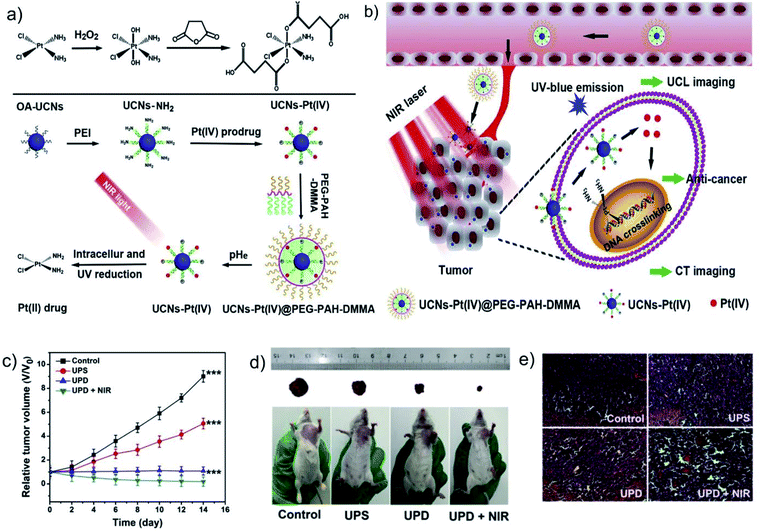 |
| Fig. 9 (a) Schematic illustration of the preparation of UCNs-Pt(IV)@PEG–PAH–DMMA. (b) Schematic illustration of UCNs-Pt(IV)@PEG–PAH–DMMA for upconversion luminescence (UCL) and computed tomography (CT) imaging dual-modality imaging and near-infrared triggered cancer treatment. (c) Tumor volume curves and (d) photographs of mice after different treatments. (e) Hematoxylin and eosin (H&E) stained images of tumor tissues after different treatments. Reprinted with permission from ref. 103. Copyright 2017, Elsevier. | |
Due to the antibacterial properties of silver ions (Ag+), silver nanoparticles (AgNPs) have become one of the most attractive commercial nanomaterials in the field of biomedicine.105 Recently, the therapeutic application of AgNPs has attracted widespread attention, especially in cancer therapy. Ag+ can kill cancer cells by inducing DNA damage, oxidative stress, and autophagy.106–108 Huang's group reported a nanomedicine (AgNC–GOD) combining glucose oxidase (GOD) and silver nanocubes (AgNC), which can achieve a synergistic treatment of starvation therapy and metal ion therapy.109 Intratumoral glucose can be oxidized by GOD into gluconic acid and H2O2, which can further promote the dissolution of AgNC into Ag+:
|  | (1) |
| 2Ag + H2O2 + 2H+ → 2Ag+ + 2H2O | (2) |
However, since the released Ag+ has certain skin, liver, and lung toxicity, etc., improving the targeting and specificity of Ag-based nanoagents is a concern for the future development of Ag-based nanoagents.
7. Conclusions and perspectives
Cancer therapeutic strategies based on metal ions are mainly based on the rational regulation of functional ions/ion clusters in cells by nanomaterials, which are used to interfere with or hinder the physiological processes of tumor cells, such as metabolism and proliferation, etc. In a broad sense, regulating the interaction between multiple types of biologically active ions and the physiological processes of tumor cells can induce tumor cell metabolism disorders, thereby inhibiting tumor growth and metastasis. We summarized and discussed the anti-tumor mechanism of nanomedicine through exogenous ion stimulation and endogenous ion interference as follows: (a) activating biocatalysis at the tumor site; (b) changing cell osmolarity; (c) affecting cell metabolism; (d) interfering with cell signal transduction; and (e) active targeting intracellular DNA. Although many anti-tumor agents based on metal ions have been synthesized and optimized in the past few decades, exception of some Pt-containing anti-tumor agents, other agents are still in the early stages. Before using such nanomaterials in actual clinical applications, many problems need to be analyzed and resolved.
(1) For metal ion therapy based on biocatalysis, improving the efficiency of biocatalysis is crucial. The unsaturated coordinating atoms on the surface of the nanocatalyst are the active sites of catalysis, so controlling the size, morphology, and crystal face of the nanocrystals to adjust the distribution and structure of the atoms on the catalyst surface can improve the catalytic performance. In addition, it is also a reasonable way to prepare heterogeneous metal-based catalysts with multiple active sites by introducing defects and single atoms. The existence of defects will cause the redistribution of the surface charge of the catalyst, which can not only promote the decomposition efficiency of the substrate, but also accelerate the circulation of metal ions between their respective high and low valence states.110 Due to the exposure of a large number of atomic active sites on the surface, single-atom catalysts have excellent catalytic properties.111 Therefore, the development of degradable nanocatalysts, defect-rich nanocatalysts, and single-atom nanocatalysts is of great significance for metal ion therapy based on biocatalysis.
(2) For metal ion therapy based on osmotic pressure, it is of great significance to improve the stability and specificity of this type of nanoagents. Through effective regulation of nanomaterial composition, degradation properties, and targeting ability, a variety of ion delivery nanoagents that can disrupt the balance of Na+ and K+ in cells can be derived. In addition, it is also of great significance to destroy the balance of Na+ and K+ in cells by other methods, such as selective ion absorption, responsive ion release, and cell autoregulation inhibition, etc.
(3) For metal ion therapy based on metabolism and signal transduction, due to the poor selectivity of most ion chelators and messenger ions, they will inevitably interfere with the metabolism and signal transduction of normal cells while treating tumor cells, resulting in unavoidable biological toxicity. Therefore, the targeted transport and specific release of ion chelators and messenger ions should receive further attention. The unique TME provides an effective trigger mechanism for the specific release of drugs. The design and synthesis of TME-responsive nanocarriers can effectively solve the non-specific problems of drugs. In addition, drawing on the design ideas of prodrugs can also effectively hide the toxicity of drugs. Using specific stimulation or bio-orthogonal approaches to “on-demand” activate prodrugs can achieve safe and specific treatments.
(4) For metal ion therapy based on DNA damage. Metal-based anti-tumor agents represented by Pt-containing agents play a very important role in cancer therapy because of their chemical structure diversity and anti-tumor activity. However, Metal-based anti-tumor agents that target DNA are prone to cause drug resistance and systemic toxicity. Therefore, to reduce the systemic toxicity of drugs and overcome the drug resistance of tumor cells, it is necessary to develop a new generation of Pt-containing agents and discover new targets or pathways. The discovery of new proteins or signal pathways related to the mechanism of Pt drugs through molecular biology can provide a theoretical basis for the development of new Pt agents. In addition, finding some drug adjuvants that can activate or inhibit related signal pathways to sensitize tumor cells is also an effective way.
(5) It is important to understand the potential response mechanism of metal ion-based agents at the specific tissue, single cell, and molecular level. Therefore, it is necessary to develop more advanced analytical techniques or sensitive probes to reveal the specific working mechanism of these metal ion-based agents in cells and even subcellular organelles intuitively or quantitatively. In recent years, multifunctional optical probes have been extensively developed and can be used to detect the concentration and distribution of specific biochemical substances in the biological environment. It is expected that these diagnostic agents may help to accurately characterize the biocatalytic process and osmotic pressure/metabolic changes induced by metal ions in vivo.
Author contributions
H. J. Zhang and Y. H. Wang conceived the topic and structure of the article. Y. Liu and Y. H. Wang conducted the literature research, drafted the manuscript, and designed the figures. H. J. Zhang and S. Y. Song reviewed and refined the manuscript.
Conflicts of interest
The authors declare no conflicts of interest.
Acknowledgements
This work was supported by the financial aid from the National Natural Science Foundation of China (Grants 52022094, 22020102003, 21834007, 51502284, and 21673220), the National Key R&D Program of China (2020YFA0712102), and the Youth Innovation Promotion Association of Chinese Academy of Sciences (Grant 2019232).
References
- C. Andreini, I. Bertin, G. Cavallaro, G. L. Holliday and J. M. Thornton, J. Biol. Inorg Chem., 2008, 13, 1205 CrossRef CAS PubMed
.
- S. P. Yu, L. M. Canzoniero and D. W. Choi, Curr. Opin. Cell Biol., 2001, 13, 405 CrossRef CAS PubMed
.
- Y. Liu, M. Zhang and W. Bu, VIEW, 2020, 1, e18 Search PubMed
.
- S. Li, L. Shao, T. Xu, X. Jiang, G. Yang and L. Dong, Biomed. Pharmacother., 2021, 137, 111401 CrossRef CAS PubMed
.
- N. Oh and J. H. Park, Int. J. Nanomed., 2014, 9, 51 Search PubMed
.
- L. Lin, S. Wang, H. Deng, W. Yang, L. Rao, R. Tian, Y. Liu, G. Yu, Z. Zhou, J. Song, H. H. Yang, Z. Y. Chen and X. Chen, J. Am. Chem. Soc., 2020, 142, 15320–15330 CrossRef CAS PubMed
.
- S. Ishidaa, P. Andreuxb, C. Poitry-Yamatec, J. Auwerxb and D. Hanahan, Proc. Natl. Acad. Sci. U. S. A., 2013, 110, 19507–19512 CrossRef PubMed
.
- J. Wang, C. Luo, C. Shan, Q. You, J. Lu, S. Elf, Y. Zhou, Y. Wen, J. L. Vinkenborg, J. Fan, H. Kang, R. Lin, D. Han, Y. Xie, J. Karpus, S. Chen, S. Ouyang, C. Luan, N. Zhang, H. Ding, M. Merkx, H. Liu, J. Chen, H. Jiang and C. He, Nat. Chem., 2015, 7, 968–979 CrossRef CAS PubMed
.
- B. Yang, Y. Chen and J. Shi, Adv. Mater., 2019, 31, 1901778 CrossRef PubMed
.
- H. Lin, Y. Chen and J. Shi, Chem. Soc. Rev., 2018, 47, 1938 RSC
.
- S. M. Hirst, A. S. Karakoti, R. D. Tyler, N. Sriranganathan, S. Seal and C. M. Reilly, Small, 2009, 5, 2848 CrossRef CAS PubMed
.
- D. Furtado, M. Bjornmalm, S. Ayton, A. I. Bush, K. Kempe and F. Caruso, Adv. Mater., 2018, 30, 1801362 CrossRef PubMed
.
- T. Hao, J. Li, F. Yao, D. Dong, Y. Wang, B. Yang and C. Wang, ACS Nano, 2017, 11, 5474 CrossRef CAS PubMed
.
- P. Zhao, Z. Tang, X. Chen, Z. He, X. He, M. Zhang, Y. Liu, D. Ren, K. Zhao and W. Bu, Mater. Horiz., 2019, 6, 369 RSC
.
- W. Zhen, W. Hu, L. Dong, S. An and X. Jiang, Nanoscale Adv., 2020, 2, 1395–1409 RSC
.
- P. Hua, T. Wu, W. Fan, L. Chen, Y. Liu, D. Ni, W. Bu and J. Shi, Biomaterials, 2017, 141, 86 CrossRef PubMed
.
- W. Li, C. Su, Y. Chang, Y. Lin and C. Yeh, ACS Nano, 2016, 10, 2017 CrossRef CAS PubMed
.
- W. Feng, X. Han, R. Wang, X. Gao, P. Hu, W. Yue, Y. Chen and J. Shi, Adv. Mater., 2019, 31, 1805919 Search PubMed
.
- R. Liang, Y. Chen, M. Huo, J. Zhang and Y. Li, Nanoscale Horiz., 2019, 4, 890 RSC
.
- H. Ranji-Burachaloo, P. A. Gurr, D. E. Dunstan and G. G. Qiao, ACS Nano, 2018, 12, 11819 CrossRef CAS PubMed
.
- Z. Tang, Y. Liu, M. He and W. Bu, Angew. Chem., Int. Ed., 2019, 58, 946 CrossRef CAS PubMed
.
- Y. Liu, Y. Jiang, M. Zhang, Z. Tang, M. He and W. Bu, Acc. Chem. Res., 2018, 51, 2502 CrossRef CAS PubMed
.
- C. Zhang, W. Bu, D. Ni, S. Zhang, Q. Li, Z. Yao, J. Zhang, H. Yao, Z. Wang and J. Shi, Angew. Chem., Int. Ed., 2016, 55, 2101–2106 CrossRef CAS PubMed
.
- M. Huo, L. Wang, Y. Chen and J. Shi, Nat. Commun., 2017, 8, 357 CrossRef PubMed
.
- Z. Tang, H. Zhang, Y. Liu, D. Ni, H. Zhang, J. Zhang, Z. Yao, M. He, J. Shi and W. Bu, Adv. Mater., 2017, 29, 1701683 CrossRef PubMed
.
- S. Zhang, L. Jin, J. Liu, Y. Liu, T. Zhang, Y. Zhao, N. Yin, R. Niu, X. Li, D. Xue, S. Song, Y. Wang and H. Zhang, Nano-Micro Lett., 2020, 12, 180 CrossRef CAS PubMed
.
- Y. Liu, W. Zhen, L. Jin, S. Zhang, G. Sun, T. Zhang, X. Xu, S. Song, Y. Wang, J. Liu and H. Zhang, ACS Nano, 2018, 12, 4886–4893 CrossRef CAS PubMed
.
- L. Lin, T. Huang, J. Song, X. Ou, Z. Wang, H. Deng, R. Tian, Y. Liu, J. Wang, Y. Liu, G. Yu, Z. Zhou, S. Wang, G. Niu, H. Yang and X. Chen, J. Am. Chem. Soc., 2019, 141, 9937–9945 CrossRef CAS PubMed
.
- B. Ma, S. Wang, F. Liu, S. Zhang, J. Duan, Z. Li, Y. Kong, Y. Sang, H. Liu, W. Bu and L. Li, J. Am. Chem. Soc., 2019, 141, 849–857 CrossRef CAS PubMed
.
- L. Lin, J. Song, L. Song, K. Ke, Y. Liu, Z. Zhou, Z. Shen, J. Li, Z. Yang, W. Tang, G. Niu, H. Yang and X. Chen, Angew. Chem., Int. Ed., 2018, 57, 4902–4906 CrossRef CAS PubMed
.
- Y. Liu, W. Zhen, Y. Wang, J. Liu, L. Jin, T. Zhang, S. Zhang, Y. Zhao, S. Song, C. Li, J. Zhu, Y. Yang and H. Zhang, Angew. Chem., Int. Ed., 2019, 58, 2407 CrossRef CAS PubMed
.
- C. Liu, Y. Chen, J. Zhao, Y. Wang, Y. Shao, Z. Gu, L. Li and Y. Zhao, Angew. Chem., Int. Ed., 2021, 60, 14324–14328 CrossRef CAS PubMed
.
- A. Tarangelo and S. J. Dixon, Nat. Nanotechnol., 2016, 11, 921–922 CrossRef CAS PubMed
.
- S. E. Kim, L. Zhang, K. Ma, M. Riegman, F. Chen, I. Ingold, M. Conrad, M. Z. Turker, M. Gao, X. Jiang, S. Monette, M. Pauliah, M. Gonen, P. Zanzonico, T. Quinn, U. Wiesner, M. S. Bradbury and M. Overholtzer, Nat. Nanotechnol., 2016, 11, 977–985 CrossRef CAS PubMed
.
- S. Xu, H. Zheng, R. Ma, D. Wu, Y. Pan, C. Yin, M. Gao, W. Wang, W. Li, S. Liu, Z. Chai and R. Li, Nat. Commun., 2020, 11, 3484 CrossRef CAS PubMed
.
- S. Zanganeh, G. Hutter, R. Spitler, O. Lenkov, M. Mahmoudi, A. Shaw, J. S. Pajarinen, H. Nejadnik, S. Goodman, M. Moseley, L. M. Coussens and H. E. Daldrup-Link, Nat. Nanotechnol., 2016, 11, 986–994 CrossRef CAS PubMed
.
- Y. Liu, J. Wu, Y. Jin, W. Zhen, Y. Wang, J. Liu, L. Jin, S. Zhang, Y. Zhao, S. Song, Y. Yang and H. Zhang, Adv. Funct. Mater., 2019, 29, 1904678 CrossRef CAS
.
- M. Li, C. Wang, Z. Di, H. Li, J. Zhang, W. Xue, M. Zhao, K. Zhang, Y. Zhao and L. Li, Angew. Chem., Int. Ed., 2019, 58, 1350–1354 CrossRef CAS PubMed
.
- C. Liu, Y. Chen, J. Zhao, Y. Wang, Y. Shao, Z. Gu, L. Li and Y. Zhao, Angew. Chem., Int. Ed., 2021, 60, 14324–14328 CrossRef CAS PubMed
.
- S. V. Torti and F. M. Torti, Nat. Rev. Cancer, 2013, 13, 342–355 CrossRef CAS PubMed
.
- O. Kakhlon and Z. Cabantchik, Free Radical Biol. Med., 2002, 33, 1037–1046 CrossRef CAS PubMed
.
- S. Kuang, X. Liao, X. Zhang, T. W. Rees, R. Guan, K. Xiong, Y. Chen, L. Ji and H. Chao, Angew. Chem., Int. Ed., 2020, 59, 3315–3321 CrossRef CAS PubMed
.
- B. P. Espósito, S. Epsztejn, W. Breuer and Z. I. Cabantchik, Anal. Biochem., 2002, 304, 1–18 CrossRef PubMed
.
- Y. Okada, Cell Biochem. Biophys., 2004, 41, 233 CrossRef PubMed
.
- F. Lang, M. Ritter, N. Gamper, S. Huber, S. Fillon, V. Tanneur, A. LeppleWienhues, I. Szabo and E. Gulbins, Cell. Physiol. Biochem., 2000, 10, 417 CrossRef CAS PubMed
.
- E. K. Hoffmann and S. F. Pedersen, Acta Physiol., 2011, 202, 465 CrossRef CAS PubMed
.
- W. Jiang, L. Yin, H. Chen, A. V. Paschall, L. Zhang, W. Fu, W. Zhang, T. Todd, K. S. Yu, S. Zhou, Z. Zhen, M. Butler, L. Yao, F. Zhang, Y. Shen, Z. Li, A. Yin, H. Yin, X. Wang, F. Y. Avci, X. Yu and J. Xie, Adv. Mater., 2019, 31, 1904058 CrossRef CAS PubMed
.
- Y. Liu, W. Zhen, Y. Wang, S. Song and H. Zhang, J. Am. Chem. Soc., 2020, 142, 21751–21757 CrossRef CAS PubMed
.
- C. D. Bortner and J. A. Cidlowski, Philos. Trans. R. Soc., B, 2014, 369, 20130104 CrossRef PubMed
.
- L. A. Pardo and W. Stuhmer, Nat. Rev. Cancer, 2014, 14, 39 CrossRef CAS PubMed
.
- J. M. Quayle, N. B. Standen and P. R. Stanfield, J. Physiol., 1988, 405, 677–697 CrossRef PubMed
.
- M. Zhang, B. Shen, R. Song, H. Wang, B. Lv, X. Meng, Y. Liu, Y. Liu, X. Zheng, W. Su, C. Zuo and W. Bu, Mater. Horiz., 2019, 6, 1034–1040 RSC
.
- B. E. Kim, T. Nevitt and D. J. Thiele, Nat. Chem. Biol., 2008, 4, 176–185 CrossRef CAS PubMed
.
- P. A. Cobinea, S. A. Mooreb and S. C. Leary, Biochim. Biophys. Acta, Mol. Cell Res., 2021, 1868, 118867 CrossRef PubMed
.
- H. Shi, Y. Jiang, Y. Yang, Y. Peng and C. Li, BioMetals, 2021, 34, 3–14 CrossRef CAS PubMed
.
- G. M. Nagaraja, M. Othman, B. P. Fox, R. Alsaber, C. M. Pellegrino, Y. Zeng, R. Khanna, P. Tamburini, A. Swaroop and R. P. Kandpal, Oncogene, 2006, 25, 2328–2338 CrossRef CAS PubMed
.
- S. Blockhuys, E. Celauro, C. Hildesjo, A. Feizi, O. Stål, J. C. Fierro-González and P. Wittung-Stafshede, Metallomics, 2017, 9, 112–123 CrossRef CAS PubMed
.
- I. P. Hargreaves, A. J. Duncan, L. Wu, A. Agrawal, J. M. Land and S. J. R. Heales, Mitochondrion, 2007, 7, 284–287 CrossRef CAS PubMed
.
- Y. Li, M. D'Aurelio, J. Deng, J. Park, G. Manfredi, P. Hu, J. Lu and Y. Bai, J. Biol. Chem., 2007, 282, 17557–17562 CrossRef CAS PubMed
.
- H. S. Carr and D. R. Winge, Acc. Chem. Res., 2003, 36, 309–316 CrossRef CAS PubMed
.
- L. Cui, A. M. Gouw, E. L. LaGory, S. Guo, N. Attarwala, Y. Tang, J. Qi, Y. Chen, Z. Gao, K. M. Casey, A. A. Bazhin, M. Chen, L. Hu, J. Xie, M. Fang, C. Zhang, Q. Zhu, Z. Wang, A. J. Giaccia, S. S. Gambhir, W. Zhu, D. W. Felsher, M. D. Pegram, E. A. Goun, A. Le and J. Rao, Nat. Biotechnol., 2021, 39, 357–367 CrossRef CAS PubMed
.
- A. J. Hoy, S. Balaban and D. N. Saunders, Trends Mol. Med., 2017, 23, 381–392 CrossRef CAS PubMed
.
- Y. Liu, Prostate Cancer Prostatic Dis., 2006, 9, 230–234 CrossRef CAS PubMed
.
- K. M. Nieman, H. A. Kenny, C. V. Penicka, A. Ladanyi, R. Buell-Gutbrod, M. R. Zillhardt, I. L. Romero, M. S. Carey, G. B. Mills, G. S. Hotamisligil, S. Diane Yamada, M. E. Peter, K. Gwin and E. Lengyel, Nat. Med., 2011, 17, 1498–1503 CrossRef CAS PubMed
.
- T. Schild, V. Low, J. Blenis and A. P. Gomes, Cancer Cell, 2018, 33, 347–354 CrossRef CAS PubMed
.
- C. Duman, K. Yaqubi, A. Hoffmann, A. A. Acikgoz, A. Korshunov, M. Bendszus, C. Herold-Mende, H. Liu and J. Alfonso, Cell Metab., 2019, 30, 274–289 CrossRef CAS PubMed
.
- N. Prevarskaya, H. Ouadid-Ahidouch, R. Skryma and Y. Shuba, Philos. Trans. R. Soc. London, Ser. B, 2014, 369, 20130097 CrossRef PubMed
.
- S. Orrenius, B. Zhivotovsky and P. Nicotera, Nat. Rev. Mol. Cell Biol., 2003, 4, 552 CrossRef CAS PubMed
.
- S. Panda, S. Behera, M. F. Alam and G. H. Syed, Mitochondrion, 2021, 58, 227–242 CrossRef CAS PubMed
.
- K. Nowicka-Bauer and M. Szymczak-Cendlak, Int. J. Mol. Sci., 2021, 22, 3259 CrossRef CAS PubMed
.
- Y. Wu, P. Huang and X. Dong, Cancers, 2021, 13, 1299 CrossRef CAS PubMed
.
- C. Zhao, Y. Tang, J. Wang, Y. Zeng, H. Sun, Z. Zheng, R. Su, K. Schneeberger, J. E. Parker and H. Cui, New Phytol., 2021, 230, 1078–1094 CrossRef CAS PubMed
.
- R. Agarwal, Kritika and S. D. Purohit, Chaos, Solitons Fractals, 2021, 143, 110610 CrossRef
.
- R. Rahban and S. Nef, Mol. Cell. Endocrinol., 2020, 518, 110951 CrossRef CAS PubMed
.
- L. Xu, G. Tong, Q. Song, C. Zhu, H. Zhang, J. Shi and Z. Zhang, ACS Nano, 2018, 12, 6806–6818 CrossRef CAS PubMed
.
- C. M. Doskey, V. Buranasudja, B. A. Wagner, J. G. Wilkes, J. Du, J. J. Cullen and G. R. Buettner, Redox Biol., 2016, 10, 274–284 CrossRef CAS PubMed
.
- G. Ermak and K. J. Davies, Mol. Immunol., 2002, 38, 713–721 CrossRef CAS PubMed
.
- P. Pinton, C. Giorgi, R. Siviero, E. Zecchini and R. Rizzuto, Oncogene, 2008, 27, 6407–6418 CrossRef CAS PubMed
.
- C. A. D. Benedictis, C. Haffke, S. Hagmeyer, A. K. Sauer and A. M. Grabrucker, Int. J. Mol. Sci., 2021, 22, 4511 CrossRef PubMed
.
- R. Magerand, P. Rey, L. Blanchard and A. de Groot, Sci. Rep., 2021, 11, 4528 CrossRef CAS PubMed
.
- W. Alker and H. Haase, Biol. Trace Elem. Res., 2021, 199, 2414–2419 CrossRef CAS PubMed
.
- K. J. Anderson, R. T. Cormier and P. M. Scott, World J. Gastroenterol., 2019, 25, 5732–5772 CrossRef CAS PubMed
.
- J. Olliga, V. Klouberta, K. M. Taylorb and L. Rink, J. Nutr. Biochem., 2019, 64, 72–79 CrossRef PubMed
.
- N. Levaot and M. Hershfinkel, Cell Calcium, 2018, 75, 53–63 CrossRef CAS PubMed
.
- W. Maret, Int. J. Mol. Sci., 2017, 18, 2285 CrossRef PubMed
.
- D. Guo, Y. Du, Q. Wu, W. Jiang and H. Bi, Arch. Biochem. Biophys., 2014, 560, 44–51 CrossRef CAS PubMed
.
- S. Choi, C. Cui, Y. Luo, S. H. Kim, J. K. Ko, X. Huo, J. Ma, L. Fu, R. F. Souza, I. Korichneva and Z. Pan, FASEB J., 2018, 32, 404–416 CrossRef CAS PubMed
.
- V. Kolenko, E. Teper, A. Kutikov and R. Uzzo, Nat. Rev. Urol., 2013, 10, 219–226 CrossRef CAS PubMed
.
- Y. Zhang, H. Wang, H. Jiang and X. Wang, Nanoscale, 2012, 4, 3530 RSC
.
- L. Lin, J. Wang, J. Song, Y. Liu, G. Zhu, Y. Dai, Z. Shen, R. Tian, J. Song, Z. Wang, W. Tang, G. Yu, Z. Zhou, Z. Yang, T. Huang, G. Niu, H. Yang, Z. Chen and X. Chen, Theranostics, 2019, 9, 7200–7209 CrossRef CAS PubMed
.
- M. Zhang, R. Song, Y. Liu, Z. Yi, X. Meng, J. Zhang, Z. Tang, Z. Yao, Y. Liu, X. Liu and W. Bu, Chem, 2019, 5, 2171–2182 CAS
.
- Q. Guan, L. Zhou, F. Lv, W. Li, Y. Li and Y. Dong, Angew. Chem., Int. Ed., 2020, 59, 18042–18047 CrossRef CAS PubMed
.
- K. Cao, X. Ding, Y. Sheng, Y. Wang and Y. Liu, Chem. Commun., 2020, 56, 4599–4602 RSC
.
- T. Fang, W. Chen, Y. Sheng, S. Yuan, Q. Tang, G. Li, G. Huang, J. Su, X. Zhang, J. Zang and Y. Liu, Nat. Commun., 2019, 10, 186 CrossRef PubMed
.
- N. P. Farrell, Chem. Soc. Rev., 2015, 44, 8773–8785 RSC
.
- A. R. Kapdi and I. J. S. FairlambL, Chem. Soc. Rev., 2014, 43, 4751–4777 RSC
.
- T. C. Johnstone, K. Suntharalingam and S. J. Lippard, Philos. Trans. R. Soc., A, 2015, 373, 20140185 CrossRef PubMed
.
- H. Song, W. Li, R. Qi, L. Yan, X. Jing, M. Zheng and H. Xiao, Chem. Commun., 2015, 51, 11493–11495 RSC
.
- C. Sanchezcano and M. J. Hannon, Dalton Trans., 2009, 48, 10702–10711 RSC
.
- L. Galluzzi, L. Senovilla, I. Vitale, J. Michels, I. Martins, O. Kepp, M. Castedo and G. Kroemer, Oncogene, 2011, 3, 1869–1883 Search PubMed
.
- Q. Cao, D. Zhou, Z. Pan, G. Yang, H. Zhang, L. Ji and Z. Mao, Angew. Chem., Int. Ed., 2020, 59, 18556–18562 CrossRef CAS PubMed
.
- S. Jin, Z. Guo and X. Wang, Prog. Pharm. Sci., 2020, 44, 280–293 Search PubMed
.
- J. Xu, Y. Kuang, R. Lv, P. Yang, C. Li, H. Bi, B. Liu, D. Yang, Y. Dai, S. Gai, F. He, B. Xing and J. Lin, Biomaterials, 2017, 130, 42–55 CrossRef CAS PubMed
.
- M. S. Shoshan, T. Vonderach, B. Hattendorf and H. Wennemers, Angew. Chem., Int. Ed., 2019, 58, 4901 CrossRef CAS PubMed
.
- B. Calderon-Jimenez, M. E. Johnson, A. R. Montoro Bustos, K. E. Murphy, M. R. Winchester and J. R. Vega Baudrit, Front. Chem., 2017, 5, 6 Search PubMed
.
- S. J. Soenen, W. J. Parak, J. Rejman and B. Manshian, Chem. Rev., 2015, 115, 2109–2135 CrossRef CAS PubMed
.
- M. I. Sriram, S. B. Kanth, K. Kalishwaralal and S. Gurunathan, Int. J. Nanomed., 2010, 5, 753–762 CAS
.
- L. Wei, J. Lu, H. Xu, A. Patel, Z. Chen and G. Chen, Drug Discovery Today, 2015, 20, 595 CrossRef CAS PubMed
.
- Y. Zhang, Y. Yang, S. Jiang, F. Li, J. Lin, T. Wang and P. Huang, Mater. Horiz., 2019, 6, 169 RSC
.
- K. Pan, C. Yang, J. Hu, W. Yang, B. Liu, J. Yang, S. Liang, K. Xiao and H. Hou, J. Hazard. Mater., 2020, 389, 122072 CrossRef CAS PubMed
.
- Y. Zhang, B. Xia, J. Ran, K. Davey and S. Z. Qiao, Adv. Energy Mater., 2020, 10, 1903879 CrossRef CAS
.
|
This journal is © The Royal Society of Chemistry 2021 |
Click here to see how this site uses Cookies. View our privacy policy here.