DOI:
10.1039/D1SC02439F
(Edge Article)
Chem. Sci., 2021,
12, 11191-11196
Organocatalytic asymmetric synthesis of α-amino esters from sulfoxonium ylides†
Received
4th May 2021
, Accepted 28th June 2021
First published on 7th July 2021
Abstract
Described here is the first organocatalytic asymmetric N–H insertion reaction of α-carbonyl sulfoxonium ylides. Without a metal catalyst, this reaction represents an attractive complement to the well-established carbene insertion reactions. As a stable surrogate of diazocarbonyl compounds, sulfoxonium ylides reacted with a range of aryl amines to provide efficient access to α-aryl glycines with excellent enantiocontrol in the presence of a suitable chiral phosphoric acid catalyst. The high stability and weak basicity of sulfoxonium ylides not only enable this protocol to be user-friendly and practically useful, but also preclude catalyst decomposition, which is crucial to the excellent amenability to electron-poor amine nucleophiles. Detailed mechanistic studies indicated that the initial protonation is reversible and the C–N bond formation is rate-determining.
Introduction
Enantioenriched α-amino esters, particularly α-aryl glycines, are not only useful building blocks in organic synthesis, but also important subunits widely present in biologically active molecules including pharmaceutical agents.1,2 Consequently, their asymmetric synthesis has been a topic of intense investigations in the past few decades.2,3 Among the various strategies, metal-catalyzed asymmetric N–H insertion of α-diazo esters via a metal–carbene complex intermediate represents an attractive and straightforward approach, leading to high levels of enantioselectivity, despite the concerns on the stability and safety issues associated with diazocarbonyl compounds particularly in large scale synthesis.4 Recently, α-carbonyl sulfoxonium ylides have been demonstrated as safe, stable, and versatile surrogates for α-diazocarbonyl compounds.4 However, the exploitation of these species for catalytic asymmetric X–H bond insertion reactions remains scarce.5 During the preparation of this manuscript, Burtoloso and coworkers reported the use of a copper/squaramide co-catalyzed system to achieve the asymmetric N–H insertion of α-carbonyl sulfoxonium ylides, leading to various α-amino esters with moderate enantioselectivities (up to 84% ee).6 Mechanistically, this reaction shares the same copper carbene intermediate as those from diazo esters, i.e., the copper catalyst is essential for the observed reactivity (Scheme 1). In contrast, a metal-free asymmetric variant of this transformation without involving a carbene intermediate remains unknown but highly desirable.7,8 Herein we report such an organocatalytic complementary process with excellent enantioselectivity and high efficiency (up to 97% ee).
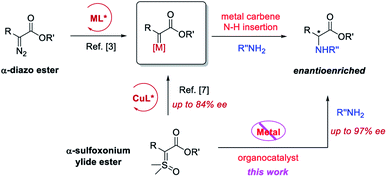 |
| Scheme 1 Introduction to asymmetric N–H insertion of α-carbonyl sulfoxonium ylides. | |
Recently, the laboratories of Burtoloso and us have demonstrated that sulfoxonium and sulfonium ylides can be activated by chiral Brønsted acids either by weak hydrogen bonding or strong protonation to form σ bonds, respectively, for asymmetric induction in the following bond-forming events.6,7b We wondered whether such interactions could be utilized for the successful amination of sulfoxonium ylides, which are much more stable and practically useful than their analogues, sulfonium ylides. However, sulfoxonium ylides are less basic due to the polarized S
O bond. The decreased basicity may weaken the interaction with the catalyst and thus lead to low reactivity and/or difficulty in enantiocontrol. Nevertheless, their weaker basicity might also be advantageous and more compatible with acid catalytic systems, e.g., obviation of catalyst decomposition (vide infra).
Results and discussion
Initially we tested the catalytic activity of chiral thiourea A for the reaction between α-carbonyl sulfoxonium ylide 1a and p-methoxyaniline 2a, since it has been the catalyst of choice for S–H insertion (Table 1, entry 1).5 Unfortunately, it resulted in completely no reactivity. Furthermore, squaramide B alone was also catalytically incompetent although it could serve as a co-catalyst for the Cu-catalyzed N–H insertion (entry 2),6 which corroborated the essential role of a metal catalyst as well as the carbene intermediate. We next resorted to stronger Brønsted acids, chiral phosphoric acids (CPAs),9 in hope of achieving sufficient reactivity. With BINOL-derived bulky chiral phosphoric acid C1, which contains two tricyclohexylphenyl substituents in the 3,3′-positions, the desired transformation proceeded cleanly to 50% conversion within 12 h at room temperature. Unfortunately, the desired product 3a was almost racemic (entry 3). Next, we screened a range of other CPAs (entries 4–7). Among them, we found that the one bearing the 9-anthryl substituent (C4) led to both high reactivity and promising enantioselectivity (>95% conversion within 15 min, 54% ee, entry 6). This result prompted us to modify the catalyst structure by introducing an additional phenyl group in the 9-anthryl substituent. To our delight, this catalyst C5 led to a dramatic increase in enantioselectivity while maintaining high chemical efficiency (79% ee). Notably, SPINOL-derived CPAs were inferior (see the ESI for details†). Further screening other solvents indicated that the reaction in polar Lewis basic solvents, such as CH3CN and THF, resulted in low reactivity and no improvement in enantioselectivity, presumably due to competing catalyst binding by these solvents. In contrast, halogenated solvents, DCM and DCE, resulted in an obvious improvement in enantioselectivity, with the former being the best (90% ee). Finally, decreasing the reaction temperature could further boost the enantioselectivity (entries 13 and 14). To compensate the low reaction rate at −10 °C, a higher concentration was employed, which proved to be optimal (95% ee, 93% isolated yield, entry 14).
Table 1 Evaluation of reaction conditionsa
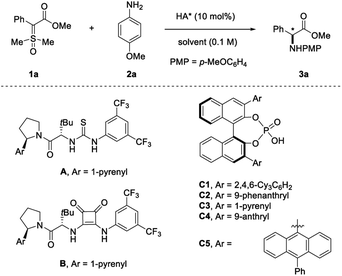
|
Entry |
HA* |
Solvent |
T/°C |
t
|
Conv.b (%) |
eeb (%) |
Reaction conditions: 1a (0.05 mmol), 2a (1.1 equiv.), catalyst (10 mol%), solvent (0.5 mL).
Conversion was determined by 1H NMR analysis of the crude reaction mixture. The reaction was clean, which means the yield was essentially the same as conversion. ee was determined by chiral HPLC.
Run with 0.2 mmol of 1a.
c = 0.2 M.
Yield in parentheses was the isolated yield.
|
1 |
A
|
DCM |
25 |
24 h |
0 |
— |
2 |
B
|
DCM |
25 |
24 h |
0 |
— |
3 |
(S)-C1 |
CHCl3 |
25 |
12 h |
50 |
3 |
4 |
(S)-C2 |
CHCl3 |
25 |
6 h |
>95 |
20 |
5 |
(S)-C3 |
CHCl3 |
25 |
6 h |
>95 |
21 |
6 |
(S)-C4 |
CHCl3 |
25 |
15 min |
>95 |
54 |
7 |
(S)-C5 |
CHCl3 |
25 |
15 min |
>95 |
79 |
8 |
(S)-C5 |
EtOAc |
25 |
14 h |
>95 |
79 |
9 |
(S)-C5 |
THF |
25 |
14 h |
82 |
80 |
10 |
(S)-C5 |
CH3CN |
25 |
14 h |
70 |
72 |
11 |
(S)-C5 |
DCM |
25 |
15 min |
>95 |
90 |
12 |
(S)-C5 |
DCE |
25 |
15 min |
>95 |
88 |
13c |
(S)-C5 |
DCM |
0 |
48 h |
>95 |
93 |
14c,d |
(S)-C5 |
DCM |
−10 |
48 h |
>95 (93)e |
95 |
We next investigated the substrate scope of this asymmetric N–H insertion reaction for the synthesis of α-aryl glycines (Scheme 2). First, various sulfoxonium ylide esters successfully participated in this process with high efficiency and enantioselectivity. Different ester groups, including methyl, ethyl, allyl, benzyl and phenyl esters, were all reactive to form the desired products with good to high ee (3a–f, Scheme 2). The absolute configuration of 3f was determined unambiguously by X-ray crystallography. The enantioselectivity slightly decreased if the size of this unit became larger. Substrates with various substituents at the α-phenyl group were also examined. Of note, the presence of a strong electron-withdrawing group, such as 3k–m, resulted in slightly lower reactivity, which required a relatively higher temperature (25 °C) to reach complete conversion. Furthermore, glycines bearing other α-aryl groups (3r–s), such as naphthyl and thiophenyl, could also be synthesized by this protocol with excellent enantioselectivity. The mild reaction conditions were compatible with a diverse array of functional groups, including alkene, aryl halide, ketone, nitrile, and nitro groups. Finally, late-stage functionalization of natural products was also possible with this process. For example, installation of a glycine unit in estrone and cholesterol could be achieved with high diastereocontrol.
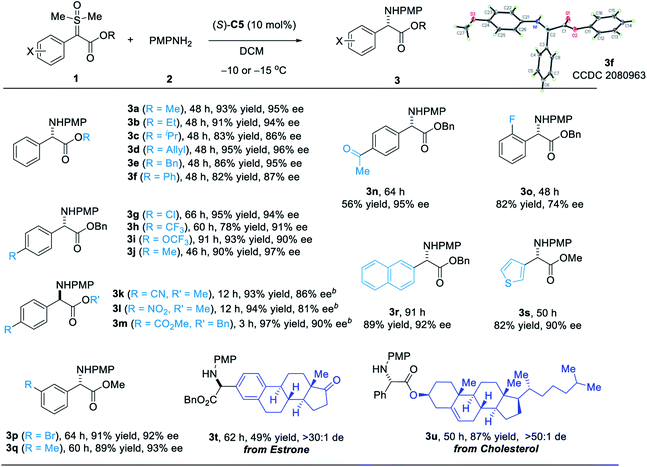 |
| Scheme 2 Scope of sulfoxonium ylides. a1 (0.2 mmol), 2 (0.22 mmol), catalyst (10 mol%) in DCM (1.0 mL). bRun with (R)-C5 at 25 °C. | |
As shown in Scheme 3, a broad range of aryl amines, including electron-rich and electron-poor ones, could all serve as good nucleophiles for this process. The reaction with electron-rich amines was generally faster than that with electron-poor ones. It is also worth mentioning that good chemoselectivity was observed. For example, for aryl amines with other nucleophilic positions, such as 8-aminonaphthalen-2-ol and 5-aminoindole, the reaction occurred only in the amine motif with excellent enantioselectivity. Aniline bearing a para-boronic ester group worked equally well with the boronate moiety untouched, which would allow further coupling or functionalization.
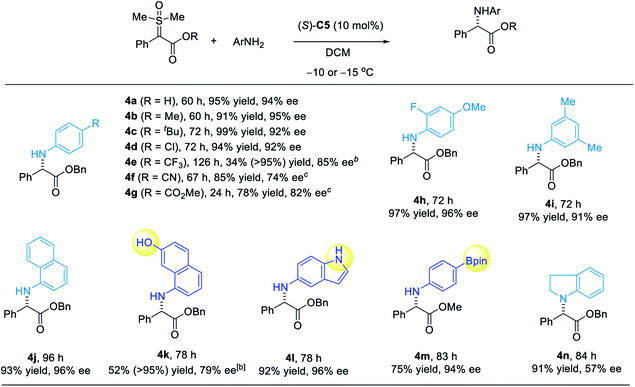 |
| Scheme 3 Scope of amines. a1 (0.2 mmol), 2 (0.22 mmol), catalyst (10 mol%) in DCM (1.0 mL). bYield in parentheses was based on the recovered starting material. cThe corresponding methyl esters were used as the substrates. | |
To demonstrate the robustness of our reaction, we also carried out a gram-scale reaction. Without modification, highly enantioenriched α-phenyl glycine 3a was obtained in 91% yield and 95% ee (Scheme 4). The catalyst was also recovered in 95% yield. It has been known that 3a is a useful precursor to free amine 4,10a which is a synthetic precursor to a wide range of useful molecules, including drugs and drug candidates with various biological activities as well as chiral catalysts.2i,10
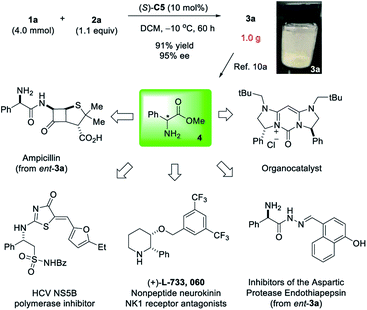 |
| Scheme 4 Gram-scale synthesis of 3a and its utility. | |
Some mechanistic studies were conducted to help understand the reaction pathway (Fig. 1). First of all, we monitored the reaction progress of the standard reaction of 1a and 2a, and the product ee remained constant during the reaction progress, suggesting that this is not simple kinetic resolution (Fig. 1a). Moreover, we employed a F-labeled amine to study the reaction kinetics using in situ19F NMR analysis (Fig. 1b). The time-dependence curves of both product formation and amine consumption were in agreement with first-order kinetics with respect to amine.11 All these results suggested that the C–N bond formation is likely the rate-determining step and the sulfoxonium protonation is reversible. We were also able to identify a linear correlation between log (e. r.) and the substituent electronic parameter (σp) of the α-phenyl group in the sulfoxonium substrate (Fig. 1c). The negative slope indicated that the electron-withdrawing substituent does not help in enantiocontrol. Combined with the lower reaction rates with the electron-withdrawing substituent at this position, these results are consistent with the C–N bond formation as the rate-determining step.
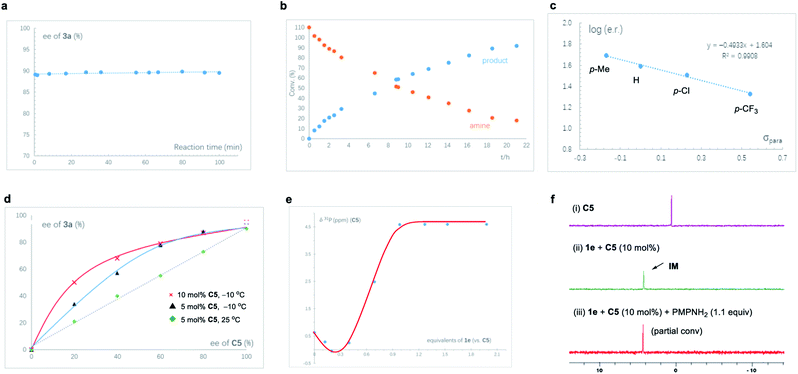 |
| Fig. 1 Mechanistic studies. (a) Time-dependence of product ee in the formation of 3a. (b) Reaction progress in the formation of 4e. (c) Correlation of enantioselectivity and the substituent electronic effect. (d) Non-linear effects. (e) Dependence of chemical shift in 31P NMR on the ratio of 1e and C5 in the NMR titration experiment. (f) Study of the catalyst resting state based on 31P NMR signals of C5 in different mixtures. | |
In addition, non-linear effect (NLE) experiments were performed (Fig. 1d). Under the standard conditions (10 mol% of C5, −10 °C), the reaction exhibited a strong positive NLE. Interestingly, reducing the catalyst loading to 5 mol% while maintaining other reaction parameters led to less pronounced deviation of this positive NLE. Furthermore, if the reaction was run at room temperature with 5 mol% of catalyst, a perfect linear relationship was observed. These observations prompted us to test the catalyst solubility under similar conditions. Indeed, upon cooling to −10 °C, a solution of racemic catalyst C5 in DCM at a concentration equivalent to that with 5 mol% and 10 mol% catalyst loadings resulted in immediate precipitation. In contrast, a clear solution (equivalent to 5 mol%) remains homogeneous at room temperature, even after standing for 24 h. Moreover, the solutions of enantiopure C5 of the same concentration (5 or 10 mol%) did not form any precipitate even at −10 °C. These observations indicated that the formation of less soluble heterochiral aggregates might be responsible for the observed NLE, and the reaction concentration and temperature may influence the outcome.12
On the basis of the above experiment, we proposed the following mechanism (with 1e as an example, Scheme 5). Despite the weak basicity of the sulfoxonium ylide, its interaction with CPA still favors the formation of an adduct (e.g., IM). The reversible nature of this step also provides a pathway for epimerization of the α-chiral center. Next, the rate-determining nucleophilic attack by amine delivers the product 3e. The chiral phosphate anion controls the stereochemistry in the C–N bond formation via dynamic kinetic resolution. Although efforts to obtain the pure intermediate for characterization were fruitless, it is important to note that the solution of this intermediate did not gradually decompose, for example, to form phosphate ester 5, even after an extended period of time. It could even be used to catalyze the reactions of other pairs of reactants with excellent yield and enantioselectivity. This is in sharp contrast to the situation using sulfonium ylides, which suffered from competitive and irreversible catalyst decomposition in this way and thus required high catalyst loading for electron-poor amines or no reactivity with many strong electron-poor amines.7b Indeed, absence of catalyst decomposition with sulfoxonium ylides led to a substantial improvement in the amenability to electron-poor amines of the present protocol.
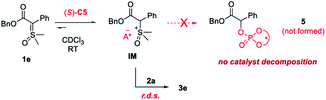 |
| Scheme 5 Proposed mechanism. | |
To further substantiate this mechanism, the interaction between the catalyst and sulfoxonium ylide was also investigated by titration experiments using 31P NMR analysis (Fig. 1e), which indicated that the protonation step is reversible favoring the intermediate.11b Furthermore, based on the in situ NMR experiment, the intermediate is the catalyst resting state (Fig. 1f). These results are all consistent with the proposed mechanism.
Finally, we have also assessed the sensitivity of this transformation to various reaction parameters to understand the robustness of this protocol according to the method developed by Glorius and coworkers.13 In this set of experiments, the influence of the reaction temperature, concentration, catalyst loading, water and oxygen content, as well as reaction scale on yield and enantioselectivity was systematically examined using the standard reaction between 1a and 2a as the reference. Only one parameter was varied in each experiment. The changes in product yield and enantioselectivity of 3a in comparison to the standard reaction conditions were illustrated using a radar chart (Fig. 2, see the ESI for details†). The results indicated that this organocatalytic asymmetric N–H insertion of sulfoxonium ylide is remarkably robust. Variation of the above mentioned parameters had little influence on the excellent outcome, indicating the good reproducibility of this protocol.
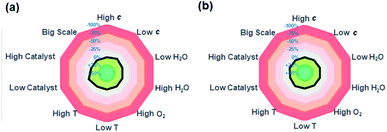 |
| Fig. 2 Sensitivity assessment of (a) reaction yield and (b) enantioselectivity. | |
Conclusions
We have developed the first organocatalytic asymmetric N–H insertion reaction of α-carbonyl sulfoxonium ylides, a safe and stable surrogate of diazocarbonyl compounds. In contrast to the well-established asymmetric insertion reactions of diazocarbonyl compounds, this protocol does not require a metal catalyst or involve a carbenoid intermediate. With the suitable chiral phosphoric acid catalyst, a range of sulfoxonium ylides could be sufficiently activated and achieve excellent enantiocontrol in the C–N bond formation. This protocol provided efficient access to a wide range of α-aryl glycines with high enantioselectivity. The mild conditions also tolerated various functional groups. More importantly, the high stability and weak basicity of sulfoxonium ylides not only enable this protocol to be more user-friendly and particularly useful, but also preclude catalyst decomposition, which is crucial to the excellent amenability to electron-poor amine nucleophiles. A series of detailed mechanistic studies provided important insights into understanding the reaction pathway, in which the initial protonation is reversible and the C–N bond formation is rate-determining.
Data availability
Details of experimental procedures, characterizations, and copy of NMR spectra as well as HPLC traces are provided in the ESI.
Author contributions
W. G., Z. H. and M. W. conceived and performed the experiments and wrote the paper. H. H. performed and directed the experiments. J. S. conceived and directed this work and wrote the paper. All the authors discussed the results and commented on the manuscript.
Conflicts of interest
There are no conflicts to declare.
Acknowledgements
Financial support was provided by the National Natural Science Foundation of China (91956114), Hong Kong Research Grants Council (16302318, 16303420), Innovation and Technology Commission (ITC-CNERC14SC01) and Jiangsu specially appointed professors program. We thank Herman H. Y. Sung for help in structural elucidation by X-ray crystallography and Shijia Li and Chang Liu for assistance in experiments.
Notes and references
-
(a) G. C. Hargaden and P. J. Guiry, Chem. Rev., 2009, 109, 2505–2550 CrossRef CAS PubMed;
(b) M. A. T. Blaskovich, J. Med. Chem., 2016, 59, 10807–10836 CrossRef CAS PubMed;
(c) D. Bardiot, M. Koukni, W. Smets, G. Carlens, M. McNaughton, S. Kaptein, K. Dallmeier, P. Chaltin, J. Neyts and A. Marchand, J. Med. Chem., 2018, 61, 8390–8401 CrossRef CAS PubMed;
(d) E. Lenci and A. Trabocchi, Chem. Soc. Rev., 2020, 49, 3262–3277 RSC;
(e) L. M. Lima, B. N. M. da Silva, G. Barbosa and E. J. Barreiro, Eur. J. Med. Chem., 2020, 208, 112829–112854 CrossRef CAS PubMed.
- For reviews (a−b) and selected recent examples of their synthesis:
(a) R. M. Williams and J. A. Hendrix, Chem. Rev., 1992, 92, 889–917 CrossRef CAS;
(b) C. Nájera and J. M. Sansano, Chem. Rev., 2007, 107, 4584–4671 CrossRef PubMed;
(c) G. Shang, Q. Yang and X. Zhang, Angew. Chem., Int. Ed., 2006, 45, 6360–6362 CrossRef CAS PubMed;
(d) M. A. Beenen, D. J. Weix and J. A. Ellman, J. Am. Chem. Soc., 2006, 128, 6304–6305 CrossRef CAS PubMed;
(e) G. Li, Y. Liang and J. C. Antilla, J. Am. Chem. Soc., 2007, 129, 5830–5831 CrossRef CAS PubMed;
(f) Q. Kang, Z.-A. Zhao and S.-L. You, Adv. Synth. Catal., 2007, 349, 1657–1660 CrossRef CAS;
(g) C. Zhu and T. Akiyama, Adv. Synth. Catal., 2010, 352, 1846–1850 CrossRef CAS;
(h) K.-J. Xiao, L. Chu and J.-Q. Yu, Angew. Chem., Int. Ed., 2016, 55, 2856–2860 CrossRef CAS PubMed;
(i) D. Liu, B. Li, J. Chen, I. D. Gridnev, D. Yan and W. Zhang, Nat. Commun., 2020, 11, 5935–5944 CrossRef CAS PubMed.
- For reviews and examples of the related synthesis of α-amino esters by metal-catalyzed N−H insertion of amines to α-diazoesters, see:
(a) S.-F. Zhu and Q.-L. Zhou, Acc. Chem. Res., 2012, 45, 1365–1377 CrossRef CAS PubMed;
(b) A. C. B. Burtoloso, J. V Santiago, B. Bernardim and A. G. Talero, Curr. Org. Synth., 2015, 12, 650–659 CrossRef CAS;
(c) Y.-Y. Ren, S.-F. Zhu and Q.-L. Zhou, Org. Biomol. Chem., 2018, 16, 3087–3094 RSC;
(d) E. C. Lee and G. C. Fu, J. Am. Chem. Soc., 2007, 129, 12066–12067 CrossRef CAS PubMed;
(e) Y. Zhu, X. Liu, S. Dong, Y. Zhou, W. Li, L. Lin and X. Feng, Angew. Chem., Int. Ed., 2014, 53, 1636–1640 CrossRef CAS PubMed;
(f) B. Liu, S.-F. Zhu, W. Zhang, C. Chen and Q.-L. Zhou, J. Am. Chem. Soc., 2007, 129, 5834–5835 CrossRef CAS PubMed;
(g) V. Arredondo, S. C. Hiew, E. S. Gutman, I. D. U. A. Premachandra and D. L. Van Vranken, Angew. Chem., Int. Ed., 2017, 56, 4156–4159 CrossRef CAS PubMed;
(h) M.-L. Li, J.-H. Yu, Y.-H. Li, S.-F. Zhu and Q.-L. Zhou, Science, 2019, 366, 990–994 CrossRef CAS PubMed.
- Reviews of sulfur ylides in organic synthesis:
(a) A. C. B. Burtoloso, R. M. P. Dias and I. A. Leonarczyk, Eur. J. Org. Chem., 2013, 2013, 5005–5016 CrossRef CAS;
(b) J. D. Neuhaus, R. Oost, J. Merad and N. Maulide, Top. Curr. Chem., 2018, 376, 15–62 CrossRef PubMed;
(c) D. Kaiser, I. Klose, R. Oost, J. Neuhaus and N. Maulide, Chem. Rev., 2019, 119, 8701–8780 CrossRef CAS PubMed. In the asymmetric domain, they have been mainly used in cycloaddition reactions. For selected examples, see:
(d) S. Klimczyk, A. Misale, X. Huang and N. Maulide, Angew. Chem., Int. Ed., 2015, 54, 10365–10369 CrossRef CAS;
(e) Q. Wang, T.-R. Li, L.-Q. Lu, M.-M. Li, K. Zhang and W.-J. Xiao, J. Am. Chem. Soc., 2016, 138, 8360–8363 CrossRef CAS.
- For the pioneering example of S–H insertion: P. B. Momo, A. N. Leveille, E. H. E. Farrar, M. N. Grayson, A. E. Mattson and A. C. B. Burtoloso, Angew. Chem., Int. Ed., 2020, 59, 15554–15559 CrossRef CAS PubMed.
- L. G. Furniel, R. Echemendía and A. C. B. Burtoloso, Chem. Sci., 2021, 12, 7453–7459 RSC.
- For a few examples using their analogues, sulfonium ylides, see:
(a) P. Müller, D. Fernandez, P. Nury and J.-C. Rossier, Helv. Chim. Acta, 1999, 82, 935–945 CrossRef; For our own effort:
(b) W. Guo, Y. Luo, H. H.-Y. Sung, I. D. Williams, P. Li and J. Sun, J. Am. Chem. Soc., 2020, 142, 14384–14390 CrossRef CAS PubMed.
- For racemic examples:
(a) H. He, K. Yan, J. Li, R. Lai, Y. Luo, M. Guan and Y. Wu, Synthesis, 2020, 52, 3065–3070 CAS;
(b) Z. Zhang, Y. Luo, H. Du, J. Xu and P. Li, Chem. Sci., 2019, 10, 5156–5161 RSC.
- Pioneering studies and recent reviews of CPA-related catalysis:
(a) T. Akiyama, J. Itoh, K. Yokota and K. Fuchibe, Angew. Chem., Int. Ed., 2004, 43, 1566–1568 CrossRef CAS PubMed;
(b) D. Uraguchi and M. Terada, J. Am. Chem. Soc., 2004, 126, 5356–5357 CrossRef CAS PubMed;
(c) D. Parmar, E. Sugiono, S. Raja and M. Rueping, Chem. Rev., 2014, 114, 9047–9153 CrossRef CAS PubMed;
(d) T. Akiyama and K. Mori, Chem. Rev., 2015, 115, 9277–9306 CrossRef CAS PubMed;
(e) T. James, M. Gemmeren and B. List, Chem. Rev., 2015, 115, 9388–9409 CrossRef CAS PubMed.
-
(a) X.-H. Hu and X.-P. Hu, Adv. Synth. Catal., 2019, 361, 5063–5068 CrossRef CAS;
(b) M. Mondal, N. Radeva, H. Köster, A. Park, C. Potamitis, M. Zervou, G. Klebe and A. K. H. Hirsch, Angew. Chem., Int. Ed., 2014, 53, 3259–3263 CrossRef CAS PubMed;
(c) A. E. Sheshenev, E. V. Boltukhina, A. J. P. White and K. K. Hii, Angew. Chem., Int. Ed., 2013, 52, 6988–6991 CrossRef CAS PubMed;
(d) A. L. Deaguero and A. S. Bommarius, Biotechnol. Bioeng., 2014, 111, 1054–1058 CrossRef CAS PubMed;
(e) G.-L. Li and G. Zhao, Org. Lett., 2006, 8, 633–636 CrossRef CAS PubMed.
-
(a) Deuterated anilines were employed to study the kinetic isotope effect. A one-pot competition experiment indicated kH/kD = 1.0 (see the ESI for details†), which suggested that protonation is not the rate-determining step; ;
(b) The initial high-field shift at high equivalents of CPA (vs. 1e) might be due to additional CPA interaction with the intermediate (e.g., H-bonding with ester or sulfoxide oxygens)..
- T. Satyanarayana, S. Abraham and H. B. Kagan, Angew. Chem., Int. Ed., 2009, 48, 456–494 CrossRef CAS PubMed.
-
(a) L. Pitzer, F. Schäfers and F. Glorius, Angew. Chem., Int. Ed., 2019, 58, 8572–8576 CrossRef CAS PubMed;
(b) D. Moock, T. Wagener, T. Hu, T. Gallagher and F. Glorius, Angew. Chem., Int. Ed., 2021, 60, 13677–13681 CrossRef CAS PubMed.
Footnote |
† Electronic supplementary information (ESI) available: 1H, 13C, and 19F NMR spectra, HPLC chromatograms, and X-ray crystallographic data for 3f. CCDC 2080963. For ESI and crystallographic data in CIF or other electronic format see DOI: 10.1039/d1sc02439f |
|
This journal is © The Royal Society of Chemistry 2021 |
Click here to see how this site uses Cookies. View our privacy policy here.