DOI:
10.1039/D1SC01889B
(Edge Article)
Chem. Sci., 2021,
12, 9088-9095
A visible-light mediated ring opening reaction of alkylidenecyclopropanes for the generation of homopropargyl radicals†
Received
6th April 2021
, Accepted 27th May 2021
First published on 28th May 2021
Abstract
Classical cyclopropylcarbinyl radical clock reactions have been widely applied to conduct mechanistic studies for probing radical processes for a long time; however, alkylidenecyclopropanes, which have a similar molecular structure to methylcyclopropanes, surprisingly have not yet attracted researcher's attention for similar ring opening radical clock processes. In recent years, photocatalytic NHPI ester activation chemistry has witnessed significant blooming developments and provided new synthetic routes for cross-coupling reactions. Herein, we wish to report a non-classical ring opening radical clock reaction using innovative NHPI esters bearing alkylidenecyclopropanes upon photoredox catalysis, providing a brand-new synthetic approach for the direct preparation of a variety of alkynyl derivatives. The potential synthetic utility of this protocol is demonstrated in the diverse transformations and facile synthesis of bioactive molecules or their derivatives and medicinal substances.
Introduction
Over the past few decades, the ring-opening reactions of alkylidenecyclopropanes (ACPs) have been vigorously explored, due to their high reactivity endowed by ring strain.1 In general, the reaction modes of alkylidenecyclopropanes can be mainly classified into the following: transition metal-catalyzed reactions,2 Lewis or Brønsted acid-catalyzed/mediated reactions,3 thermal induced cyclizations4 and free radical additions (Scheme 1A).5 Since the 1970s, the research on the chemistry of ACPs upon transition metal catalysis has been in progress, and four different reaction patterns have been disclosed thus far (Scheme 1A, a). The distal bond (C3–C4) and proximal bond (C2–C3 or C2–C4) of ACPs can be inserted by transition metal M to undergo the subsequent reactions. On the other hand, the organo-transition metal reagent R–MLn can be added into alkylidenecyclopropanes via anti-Markovnikov or Markovnikov addition, leading to the ring opening of cyclopropane or other transformations. In the reaction of ACPs with Lewis or Brønsted acids, the cyclopropyl ring can be activated to undergo ring opening to form two different zwitterionic intermediates, which can undergo further transformations (Scheme 1A, b). As for thermally induced cyclizations, heating would facilitate the double bond of ACPs to react with a dienophile or dipole to afford spirocyclic derivatives (Scheme 1A, c).
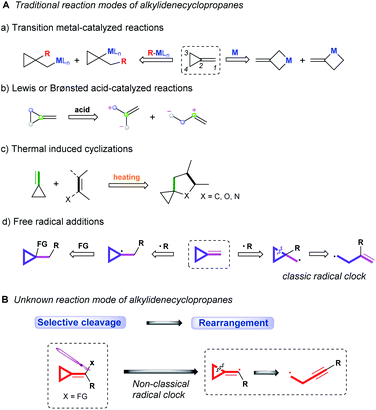 |
| Scheme 1 Traditional reaction modes of alkylidenecyclopropanes and unknown reaction mode of alkylidenecyclopropanes. | |
Furthermore, the application of alkylidenecyclopropanes in radical C–C bond forming reactions has also received much attention. The addition of radicals into alkylidenecyclopropanes could generate cyclopropyl radicals or cyclopropylcarbinyl radicals. The former can be further transformed to achieve the difunctionalization of alkenes, and the latter can undergo β-scission in the cyclopropyl ring to afford the homoallyl radical known as a classical radical clock (Scheme 1A, d). These synthetic methods provide an efficient access to the rapid generation of molecular complexity. However, with increasing exploration on research means and technologies, nearly no breakthrough on the chemical transformation of alkylidenecyclopropanes has been made for a long time. More efforts to develop new reaction modes of alkylidenecyclopropanes are highly desired in current research.
The ring opening of the cyclopropylcarbinyl (CPC) radical to the 3-butenyl radical is a fast radical rearrangement that holds a position of distinction in mechanistic studies involving “radical clocks” and “mechanistic probes”. Numerous research studies have adopted potential precursors of this radical or its analogues in attempts to implicate radical intermediates in a reaction pathway by the detection of the products from the radical ring opening process.6 At the same time, it is found that this methodology and the final detected products bearing alkenyl groups can be applied to diverse organic synthesis.7 Since alkylidenecyclopropanes (ACPs) have a similar molecular structure to methylcyclopropane and the release of ring strain can provide a potent thermodynamic driving force, we designed an unknown radical reaction mode, in which the C(sp2)–X bond of alkylidenecyclopropane can be selectively cleaved to afford the alkylidenecyclopropane radical (Scheme 1B). This radical can further undergo the same ring opening rearrangement process to generate the corresponding radical species containing an alkynyl moiety and subsequently play a vital role in mechanistic studies and diverse organic synthesis. Thus, we conducted preliminary density functional theory (DFT) calculations to predict whether the envisaged process is kinetically feasible (Scheme 2A) (see the ESI† for the details). The calculation results showed that the energy barrier for the ring-opening process of the alkylidenecyclopropane radical
is lower than that for the ring-opening process of the CPC radical
indicating that the ring-opening process of the alkylidenecyclopropane radical is kinetically favorable. In addition, the ring-opening process of the alkylidenecyclopropane radical is an exergonic process (ΔG1 = −13.4 kcal mol−1), suggesting that this is a thermodynamically favorable process. Based on these calculation results, developing reactions involving the ring opening process of the alkylidenecyclopropane radical is feasible.
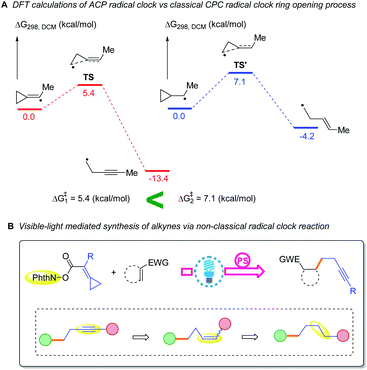 |
| Scheme 2 DFT calculations of the ACP radical clock vs. classical CPC ring opening radical clock process. Alkylidenecyclopropanes and this work. | |
Recently, NHPI (N-hydroxyphthalimide) esters, as stable and readily accessible compounds, have emerged as a novel and efficient synthon to undergo a number of interesting and practical transformations.8 Due to their redox reactivity, NHPI esters can serve as a radical precursor to accept an electron to trigger a consecutive domino of events that release phthalimidyl anions, CO2 and the corresponding radicals applied for a variety of reactions.9 Photoredox catalysis induced by visible light, owing to the advantages of ease of handling, application safety and natural abundance, is utilized as one of the accesses fulfilling the described process.10 Visible-light induced carbon–carbon bond-forming reactions involving NHPI esters were identified as an ideal strategy to construct various interesting compounds. Such reactions can be categorized as follows: (i) C(sp3)–C(sp3) bond formation; (ii) C(sp3)–C(sp2) bond formation; (iii) C(sp3)–C(sp) bond formation; (iv) C(sp3)–B or Se/S bond formation.8 However, in these reactions, NHPI esters directly release the C(sp3) radicals applied for the bond formation. No examples of releasing the highly reactive vinyl radicals as C(sp2) radicals have been reported to date. With regard to this aspect, we skillfully take advantage of the high reactivity of C(sp2) radicals to fulfill our design. Building on the widely investigated field of classical ring opening radical clock reactions and the successes of photocatalytic NHPI esters in decarboxylative activity chemistry, we are inspired by the possibility of an innovative non-classical ring opening radical clock reaction to provide both terminal and internal alkynes (Scheme 2B). Notably, it is convinced that different levels of saturation of small molecules can cause a broad spectrum of transformations, especially applicable in the areas of pharmaceutical and agrochemical production. Therefore, the transformations of alkynes into alkenes and alkanes fulfilled by our envisaged method would provide a practical synthetic route (Scheme 2B).
Results and discussion
Experimental investigations
We began our investigation with easily available NHPI ester 1b (0.075 mmol, 1.5 equiv.) and phenyl vinyl ketone 2a (0.05 mmol, 1.0 equiv.) as the model substrates, Ru(bpy)3(PF6)2 as the photosensitizer, and Hantzsch ester and iPr2NEt as additives in dichloromethane (0.75 mL) at room temperature upon the irradiation of a 15 W blue LED for 3 h, giving the desired alkyne 3ba in 87% yield (Table 1, entry 1). The optimization of reaction conditions revealed that the photosensitizer, light and additive were indispensable in this reaction (Table 1, entries 2–4). Other metal-polypyridyl complex photosensitizers were also screened, and the results showed that Ru(bpz)3(PF6)2 was less effective as compared with Ru(bpy)3(PF6)2, but the use of fac-Ir(ppy)3 did not afford the desired product (Table 1, entries 5-6). Instead of iPr2NEt, other additives such as DBU and DABCO were tested, which resulted in decreased yields (Table 1, entries 7-8). We further investigated other solvents, such as THF and DCE, providing 3ba in 71% and 57% yields (Table 1, entries 9-10), respectively, identifying DCM as the most suitable solvent. Using 1.0 equiv. of 1b or prolonging the reaction time to 12 h could not effectively improve the yield of 3ba (Table 1, entries 11-12).
Table 1 Optimization of the reaction conditionsa

|
Entry |
Condition |
Yieldb (%) |
Reaction conditions: 1b (0.075 mmol, 1.5 equiv.), 2a (0.05 mmol, 1.0 equiv.), Hantzsch ester (HEH) (0.075 mmol, 1.5 equiv.), iPr2NEt (0.11 mmol, 2.2 equiv.), Ru(bpy)3(PF6)2 (2 mol%), DCM (0.75 mL), rt, 3 h, Ar, and 15 W blue LED. All the reactions were carried out on a 0.05 mmol scale in solvent (0.75 mL) at rt for 3 h unless otherwise specified.
Isolated yield.
|
1 |
Standard condition |
87 |
2 |
Without Ru(bpy)3(PF6)2 |
NR |
3 |
Without blue LED |
NR |
4 |
Without iPr2NEt |
15 |
5 |
Ru(bpz)3(PF6)2 instead of Ru(bpy)3(PF6)2 |
34 |
6 |
fac-lr(ppy)3 instead of Ru(bpy)3(PF6)2 |
NR |
7 |
DBU instead of iPr2NEt |
21 |
8 |
DABCO instead of iPr2NEt |
13 |
9 |
THF instead of DCM |
71 |
10 |
DCE instead of DCM |
57 |
11 |
1.0 equiv. 1b |
66 |
12 |
12 h instead of 3 h |
84 |
With the optimal reaction conditions in hand, we attempted to investigate the substrate scope of various NHPI esters bearing alkylidenecyclopropanes and the results are shown in Table 2. When the substituent R was a hydrogen atom, the reaction could proceed smoothly to provide the terminal alkyne 3aa in 75% yield. For substrates 1c and 1d, ethyl group and propyl group substituted NHPI esters were compatible in this reaction, generating the corresponding internal alkynes 3ca and 3da in 89% and 92% yields, respectively. When the R group was changed into the benzyl group bearing different substituents, such as 4-H, 4-OMe, 4-Cl and 3,5-Br (1e–1h), the desired products 3ea–3ha were obtained in 89–93% yields. Moreover, we attempted to introduce featured substituents, such as allyl, propargyl and cinnamyl groups, into the substrates, and found that the reactions also proceeded smoothly to afford the corresponding internal alkynes 3ia, 3ja and 3ka in good yields ranging from 80–94%. Notably, the substrate 1la having an aromatic heterocycle was also suitable for this reaction, affording the desired product 3la in 85% yield.
Table 2 Substrate scope of NHPI esters bearing alkylidenecyclopropanesa
Reaction conditions: 1 (0.3 mmol, 1.5 equiv.), 2a (0.2 mmol, 1.0 equiv.), Hantzsch ester (HEH) (0.3 mmol, 1.5 equiv.), iPr2NEt (0.44 mmol, 2.2 equiv.), Ru(bpy)3(PF6)2 (2 mol%), DCM (3 mL), rt, 3 h, Ar, and 15 W blue LED. Yields were determined from isolated products.
|
|
Next, we focused on the generality of this protocol regarding α,β-unsaturated carbonyl compounds. As outlined in Table 3, most of the reactions proceeded smoothly under well-established conditions to generate the expected products in moderate to good yields. First, we examined aryl substituted α,β-unsaturated ketones (2b–2i). The mild conditions enabled the tolerance to important functional groups, such as halide, cyano, ester, alkoxy and so on, affording the corresponding alkynes 3bb–3bi, in the yields ranging from 75% to 92%. Moreover, alkyl substituted α,β-unsaturated ketones (2j–2n) were also compatible in this reaction, affording the desired alkynes 3bj–3bn, albeit with moderate yields (36–43%). For the α,β-unsaturated amide 2o, the reaction could also proceed smoothly to produce the desired product 3bo in 33% yield. When the α position of α,β-unsaturated ketones was substituted by ester and phenyl groups, the reaction occurred smoothly to give the expected products 3bp and 3bq in 87% and 81% yields, respectively. For conjugated diene substrate 2r, in which the δ position was substituted by a methyl group, the corresponding product 3br was obtained in 92% yield, probably due to the radical addition taking place at the δ position and the relative stability of key radical intermediates. It is well known that aromatic heterocycles play an important role in medicinal chemistry; therefore, α,β-unsaturated ketones bearing furyl, thienyl, pyrrole, indolyl, benzofuryl and imidazolyl groups were applied to this reaction to generate alkynes having aromatic heterocycles. To our delight, these reactions also proceeded very well to provide the corresponding alkynes 3bs–3bx in moderate to good yields. For cyclic exo-methylene ketones 2y and 2z, the desired products 3by and 3bz were obtained in both 78% yields. Notably, when the alkenyl sulfone 2aa was utilized as the substrate, the desulfonylative cross-coupling reaction occurred, resulting in the formation of product 3baa in 97% yield. Finally, we illustrated the utility of this novel non-classical radical clock reaction using the core structures of several bioactive molecules, such as geraniol (2ab), estrone (2ad) and vanillin (2ae), and a mesogenic compound (2ac). All the desired alkynylated products were obtained in good yields (78–88%), irrespective of existing complex molecular structures. In addition, acrylesters, acrylamides, exo-cyclic lactone and simple crotonyl or cinnamoyl substrates were also attempted to use as the radical acceptors to proceed this reaction; however, no desired products were obtained (for unsuccessful examples, see Page S3 in the ESI†). Unfortunately, we did not succeed in obtaining an aryl-substituted substrate after several attempts.
Table 3 Substrate scope of α,β-unsaturated ketonesa
Reaction conditions: 1b (0.3 mmol, 1.5 equiv.), 2 (0.2 mmol, 1.0 equiv.), Hantzsch ester (HEH) (0.3 mmol, 1.5 equiv.), iPr2NEt (0.44 mmol, 2.2 equiv.), Ru(bpy)3(PF6)2 (2 mol%), DCM (3 mL), rt, 3 h, Ar, and 15 W blue LED. Yields were determined from isolated products.
|
|
Synthetic applications
To demonstrate the preparative utility of our methodology, product transformations were conducted (Scheme 3A). The resulting alkynes can act as versatile forging blocks for diversity-oriented synthesis. A rhodium-catalyzed hydrosilylation reaction and gold-catalyzed cyclization of 3baf were conducted to afford the corresponding products, silylated compound 4 and cyclized product 5 in 87% and 90% yields, respectively. The Sonogashira cross-coupling reaction of 3baf with vinyl bromide also proceeded smoothly, giving the enyne 6 in 99% yield, which could be utilized for the construction of valuable building blocks, such as pyrroles. The click reaction of 3baf with tosyl azide via copper catalysis afforded the [3 + 2] cycloaddition product 7 in 95% yield, demonstrating the potential practicability of the resulting alkynes in medicinal chemistry. Hydrogenation or partial hydrogenation of the obtained alkyne 3baf effectively generated the corresponding alkane 8 and alkene 9. As for the immediate potential in biochemistry and medicinal chemistry, we have easily synthesized five different synthetic precursors 10, 11, 12, 15 and 16 on a gram scale through this methodology (Scheme 3B). Compound 10 was synthesized from 3ba through a two-step reduction procedure in 65% yield (0.91 g), which was regarded as the key intermediate for the synthesis of Fingolimod, mainly available for the treatment of Multiple Sclerosis (MS).11 Starting from 3aa, the two vital precursors 11 and 12 were synthesized in 46% (0.56 g) and 43% (0.49 g) yields, respectively. Precursors 11 and 12 could be further transformed into substituted imidazo [1,5-b] pyridazine 13 and its analogue 14, which are proved to be exceptionally active against the reverse transcriptase of HIV-1, in 64% (0.68 g) yield and 62% (0.64 g) yield, respectively12 (for details on the formal synthesis or total synthesis of bioactive molecules, see Pages S13 and S14 in the ESI†). Since 1977, Tramadol has been used as a painkiller by serving as a weak μ-opioid receptor agonist;13 therefore, we synthesized the vital precursor 15 through a one-pot selective hydroboration and oxidation of alkyne 3baf in 59% yield (0.69 g). Furthermore, on the basis of Razdan's protocol,14 we prepared the building block 16 in 79% yield (0.63 g) through the reduction and demethylation of alkyne 3baf, which could be applied to assemble the novel tetrahydrocannabinol/anandamide (THC/AEA) hybrid ligand. This modified ligand could significantly improve its binding affinity to the CB1 receptor in biological research. In summary, these diverse transformations exhibit the strong capability of our non-classical radical clock reaction coupled with various subsequent manipulations for generating terminal or internal alkynes and their derivatives, which would be synthetically very useful along with excellent functional group tolerance.
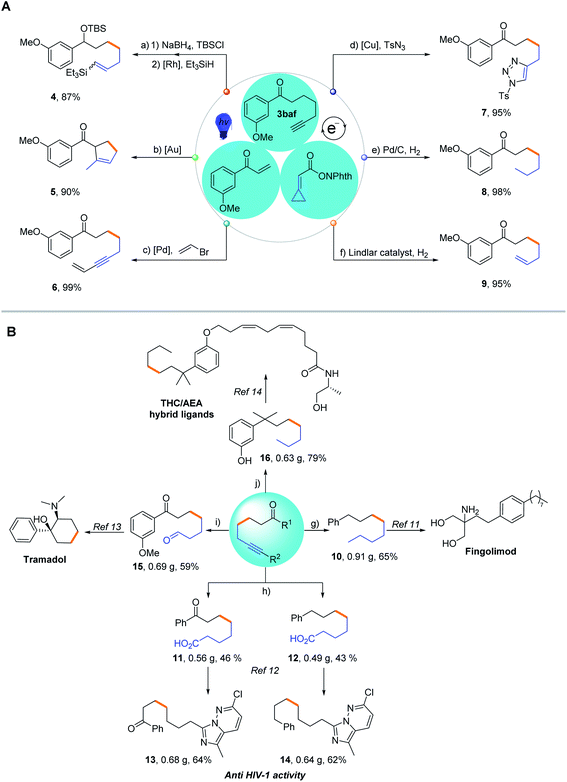 |
| Scheme 3 Synthetic applications of the non-classical ring opening radical clock reaction. (A) Product transformations of 3baf. (a) NaBH4, MeOH, 0 °C – rt; DMAP, imidazole, TBSCl, DCM, 0 °C – rt, and 12 h; Rh(COD)2BF4, PPh3, Et3SiH, acetone, rt, and 20 h. (b) AuCl3, AgOTf, toluene, rt, and 8 h. (c) Pd(PPh3)2Cl2, CuI, Et3N, THF, rt, 12 h. (d) CuTc, toluene, rt, and 8 h. (e) Pd/C, MeOH, H2, rt, and 4 h. (f) Lindlar catalyst, MeOH, H2, rt, and 8 h. (B) Synthesis of drugs applying our methodology. (g) Pd/C, MeOH, H2, rt, and 4 h; NaBH4, AlCl3, THF, and 0 °C - 65 °C. (h) ethylene glycol, p-TsOH, toluene, and 110 °C; n-BuLi, propyl chloroformate, THF, and −78 °C; Pd/C, MeOH, H2, rt, and overnight; LiOH, THF, H2O, and rt; DMAP, DCC, Et3N, DCM, DMF, and 0 °C – rt; 6-(1-aminoethyl)pyridazin-3(2H)-one S6, POCl3, DCE, reflux, and 3 h. (i) BH3·THF, cyclohexene, THF, 0 °C, and 2 h; NaBO3·4H2O, H2O, and 2 h. (j) Pd/C, MeOH, H2, rt, and 4 h; TiCl4, (CH3)2Zn, DCM, −50 °C, and 2 h; BBr3, DCM, 0 °C, and 12 h. | |
Mechanistic studies
To gain more insights into the reaction mechanism, the fluorescence quenching experiments of Ru(bpy)3(PF6)2 with 1b were performed and its Stern–Volmer analysis is depicted in Fig. 1, indicating that 1b could not be photo-excited by the photocatalyst effectively. In addition, we carried out several control experiments (Scheme 4). First, when TEMPO as a radical scavenger was subjected to our model reaction, the non-classical radical clock reaction was inhibited completely and a new product 17 generated from the resultant alkyl radical trapped by TEMPO was obtained in 49% yield (Scheme 4, a). Subsequently, a reaction using deuterium-labeled Hantzsch ester d3-2af was conducted, and the deuterium atom was incorporated exclusively at the α position of alkyne d1-3ba, showing that SET and HAT processes were both involved in the last step15 (Scheme 4, b). Substrate 1a (E1/2 = −1.18 V vs. SCE, see Page S18 in the ESI†) and radical clock substrate 1m (E1/2 = −1.24 V vs. SCE, see Page S18 in the ESI†) were mixed together to undergo the reaction, affording the corresponding products 3aa and 18 in 48% and 16% yield, respectively (Scheme 4, c). This reaction outcome basically agrees with the prediction of DFT calculations, which indicate that the ring-opening process of the alkylidenecyclopropane radical is kinetically more favorable.
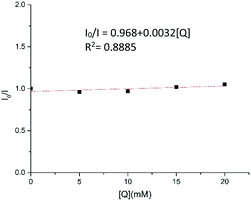 |
| Fig. 1 Stern–Volmer quenching experiment. | |
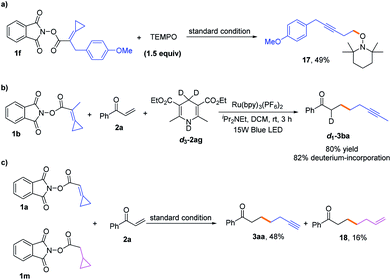 |
| Scheme 4 Mechanistic investigations. | |
On the basis of generally accepted mechanisms for NHPI ester's decarboxylative activity,8,15 a plausible catalytic cycle is proposed in Scheme 5. The initial excitation of Ru(bpy)3(PF6)2 produces the excited state
which undergoes SET with iPr2NEt or Hantzsch ester to generate the Ru(I), amine radical cation or Hantzsch ester radical cation. The N-(acyloxy)phthalimide 1 receives an electron from Ru(I) (E1/2 = −1.33 V vs. SCE), to transiently form radical anionic intermediate A. Next, an entropically favored decarboxylation can proceed via two plausible pathways to generate the desired radical intermediate D: (i) rapid homolytic fragmentation and decarboxylation from A to release the phthalimide anion, CO2 and the radical intermediate B. As a central design element, we postulated that B would undergo the ring-opening process to produce the nucleophilic carbon-centered radical bearing alkynyl D, or (ii) homolytic fragmentation of the N–O bond to furnish intermediate C, which can further undergo decarboxylation and radical rearrangement to D. The addition of this nucleophilic radical to a conjugate acceptor 2 generates a stabilized radical E, which then undergoes SET and HAT processes to successfully form the functionalized alkyne 3. Notably, the radical intermediate E was not engaged in a fast 5-exo-dig-cyclization to afford the cyclized product; therefore, we assumed that the cyclization is reversible and reduction only occurred on the ring-opened isomer.
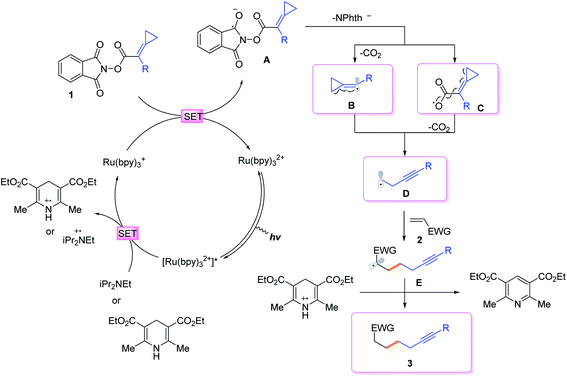 |
| Scheme 5 Proposed reaction mechanisms. | |
Conclusions
In conclusion, we have successfully established a robust methodology to access alkynes via the versatile and practical non-classical ring-opening radical clock reactions of NHPI esters bearing ACPs inspired by conventional photocatalytic NHPI ester's activation and classical radical clock reactions. Compared with the traditional reaction modes of alkylidenecyclopropanes, this method is totally new and its prospect will be prosperous for the rapid generation of molecular complexity in organic synthesis. Most importantly, since the carbon–carbon triple bond can serve as a versatile functional group for diverse organic synthesis, the resulting alkynes can be transformed into a variety of bioactive molecules and natural products. Further studies for discovering detailed mechanisms and novel reaction types to synthesize other useful compounds are underway.
Author contributions
X.-Y. Z., C.N. and B. M. contributed to the experimental work; Y. W. contributed to the computational work. X.-Y. Z., Y. W. and M. S. contributed to ideation and writing of the paper.
Conflicts of interest
There are no conflicts to declare.
Acknowledgements
We are grateful for the financial support from the Strategic Priority Research Program of the Chinese Academy of Sciences (Grant No. XDB20000000), the National Natural Science Foundation of China (21372250, 21121062, 21302203, 20732008, 21772037, 21772226, 21861132014 and 91956115), the Project supported by Shanghai Municipal Science and Technology Major Project (Grddant No. 2018SHZDZX03) and the Fundamental Research Funds for the Central Universities 222201717003.
References
-
(a) A. Brandi and A. Goti, Chem. Rev., 1998, 98, 589–636 Search PubMed
;
(b) A. Brandi, S. Cicchi, F. M. Cordero and A. Goti, Chem. Rev., 2003, 103, 1213–1270 Search PubMed
;
(c) G. Audran and H. Pellissier, Adv. Synth. Catal., 2010, 352, 575–608 Search PubMed
;
(d) M. Shi, J.-M. Lu, Y. Wei and L.-X. Shao, Acc. Chem. Res., 2012, 45, 641–652 Search PubMed
;
(e) D.-H. Zhang, X.-Y. Tang and M. Shi, Acc. Chem. Res., 2014, 47, 913–924 Search PubMed
;
(f) L.-Z. Yu, K. Chen, Z.-Z. Zhu and M. Shi, Chem. Commun., 2017, 53, 5935–5945 Search PubMed
;
(g) X.-Y. Zhang, P.-H. Li and M. Shi, Asian J. Org. Chem., 2018, 7, 1924–1933 Search PubMed
.
-
(a) N. Tsukada, A. Shibuya, I. Nakamura and Y. Yamamoto, J. Am. Chem. Soc., 1997, 119, 8123–8124 Search PubMed
;
(b) M. Shi, L.-P. Liu and J. Tang, J. Am. Chem. Soc., 2006, 128, 7430–7431 Search PubMed
;
(c) C. Aïssa and A. Fürstner, J. Am. Chem. Soc., 2007, 129, 14836–14837 Search PubMed
;
(d) S. Cui, Y. Zhang and Q. Wu, Chem. Sci., 2013, 4, 3421–3426 Search PubMed
;
(e) J. C. Timmerman, B. D. Robertson and R. A. Widenhoefer, Angew. Chem., Int. Ed., 2015, 54, 2251–2254 Search PubMed
;
(f) W. Fang, Y. Wei and M. Shi, Org. Lett., 2018, 20, 7141–7144 Search PubMed
;
(g) W. Fang and M. Shi, Chem. –Eur. J., 2018, 24, 9998–10005 Search PubMed
;
(h) B. Cao, Y. Wei and M. Shi, Chem. Commun., 2018, 54, 14085–14088 Search PubMed
.
-
(a) M. Shi and B. Xu, Org. Lett., 2002, 4, 2145–2148 Search PubMed
;
(b) M. Shi, B. Xu and J.-W. Huang, Org. Lett., 2004, 6, 1175–1178 Search PubMed
;
(c) L.-X. Shao, B. Xu, J.-W. Huang and M. Shi, Chem. –Eur. J., 2006, 12, 510–517 Search PubMed
;
(d) M. E. Scott, Y. Bethuel and M. Lautens, J. Am. Chem. Soc., 2007, 129, 1482–1483 Search PubMed
;
(e) J.-W. Huang and M. Shi, Synlett, 2004, 2004, 2343–2346 Search PubMed
.
-
(a) I. Nakamura, T. Nemoto, Y. Yamamoto and A. de Meijere, Angew. Chem., Int. Ed., 2006, 45, 5176–5179 Search PubMed
;
(b) X.-Y. Tang and M. Shi, J. Org. Chem., 2009, 74, 5983–5986 Search PubMed
;
(c) K. Chen, R. Sun, Q. Xu, Y. Wei and M. Shi, Org. Biomol. Chem., 2013, 11, 3949–3953 Search PubMed
;
(d) X.-Y. Zhang, S. Yang, Y. Wei and M. Shi, Org. Biomol. Chem., 2018, 16, 6399–6404 Search PubMed
.
-
(a) B. Xu, Y. Chen and M. Shi, Tetrahedron Lett., 2002, 43, 2781–2784 Search PubMed
;
(b) J.-W. Huang and M. Shi, J. Org. Chem., 2005, 70, 3859–3863 Search PubMed
;
(c) T. Kippo, K. Hamaoka and I. Ryu, J. Am. Chem. Soc., 2013, 135, 632–635 Search PubMed
;
(d) L. Yu, Y. Wu, T. Chen, Y. Pan and Q. Xu, Org. Lett., 2013, 15, 144–147 Search PubMed
;
(e) Z.-Z. Zhu, K. Chen, L.-Z. Yu, X.-Y. Tang and M. Shi, Org. Lett., 2015, 17, 5994–5997 Search PubMed
;
(f) Y.-C. Yuan, H.-L. Liu, X.-B. Hu, Y. Wei and M. Shi, Chem. –Eur. J., 2016, 22, 13059–13063 Search PubMed
;
(g) X. Fan, L.-Z. Yu, Y. Wei and M. Shi, Org. Lett., 2017, 19, 4476–4479 Search PubMed
;
(h) X.-Y. Zhang, C. Ning, Y.-J. Long, Y. Wei and M. Shi, Org. Lett., 2020, 22, 5212–5216 Search PubMed
.
-
(a) L. Huang, A. M. Olivares and D. J. Weix, Angew. Chem., Int. Ed., 2017, 56, 11901–11905 Search PubMed
;
(b) W. Zhang, F. Wang, S. D. McCann, D. Wang, P. Chen, S. S. Stahl and G. Liu, Science, 2016, 353, 1014–1018 Search PubMed
;
(c) W. Zhao, R. P. Wurz, J. C. Peters and G. C. Fu, J. Am. Chem. Soc., 2017, 139, 12153–12156 Search PubMed
;
(d) Q. Fu, Z.-Y. Bo, J.-H. Ye, T. Ju, H. Huang, L.-L. Liao and D.-G. Yu, Nat. Commun., 2019, 10, 3592–3595 Search PubMed
;
(e) J. H. Blackwell, R. Kumar and M. J. Gaunt, J. Am. Chem. Soc., 2021, 143, 1598–1609 Search PubMed
.
-
(a) S. Handa and G. Pattenden, Chem. Commun., 1998, 311–312 Search PubMed
;
(b) G. Rüedi and H.-J. Hansen, Tetrahedron Lett., 2004, 45, 5143–5145 Search PubMed
;
(c) Y. Takekawa and K. Shishido, J. Org. Chem., 2001, 66, 8490–8503 Search PubMed
;
(d) T. Fukuyama, T. Kippo, K. Hamaoka and I. Ryu, Sci. China Chem., 2019, 62, 1525–1528 Search PubMed
.
-
(a) S. Murarka, Adv. Synth. Catal., 2018, 360, 1735–1753 Search PubMed
;
(b) K. Okada, K. Okamoto, N. Morita, K. Okubo and M. Oda, J. Am. Chem. Soc., 1991, 113, 9401–9402 Search PubMed
.
-
(a)
H. Subramanian, Y. Landais and M. P. Sibi, Radical Addition Reactions, in Comprehensive Organic Synthesis II, ed. P. Knoche, Elsevier, Amsterdam, 2nd edn, 2014, pp. 699–741 Search PubMed
;
(b) M. P. Sibi, S. Manyem and J. Zimmerman, Chem. Rev., 2003, 103, 3263–3296 Search PubMed
.
-
(a) J. Xuan, Z.-G. Zhang and W.-J. Xiao, Angew. Chem., Int. Ed., 2015, 54, 15632–15641 Search PubMed
;
(b) M. H. Shaw, J. Twilton and D. W. C. MacMillan, J. Org. Chem., 2016, 81, 6898–6926 Search PubMed
;
(c) N. Vallavoju, S. Selvakumar, B. C. Pemberton, S. Jockusch, M. P. Sibi and J. Sivaguru, Angew. Chem., Int. Ed., 2016, 55, 5446–5451 Search PubMed
;
(d) L. Candish, M. Teders and F. Glorius, J. Am. Chem. Soc., 2017, 139, 7440–7443 Search PubMed
;
(e) T. Fukuyama, T. Nishikawa, K. Yamada, D. Ravelli, M. Fagnoni and I. Ryu, Org. Lett., 2017, 19, 6436–6439 Search PubMed
;
(f) Y. Zhao, J.-R. Chen and W.-J. Xiao, Org. Lett., 2018, 20, 224–227 Search PubMed
;
(g) M.-C. Fu, R. Shang, B. Zhao, B. Wang and Y. Fu, Science, 2019, 363, 1429–1434 Search PubMed
.
- R. U. Maruthi, R. B. Venkateswara and V. Siddaiah, Der Pharma Chemica, 2017, 9, 36–40 Search PubMed
.
- D. G. H. Livermore, R. C. Bethell, N. Cammack, A. P. Hancock, M. M. Hann, D. V. S. Green, R. B. Lamont, S. A. Noble and D. C. Orr, J. Med. Chem., 1993, 36, 3784–3794 Search PubMed
.
- F. Lecerf-Schmidt, R. Haudecoeur, B. Peres, M. M. Ferreira Queiroz, L. Marcourt, S. Challal, E. Ferreira Queiroz, G. Sotoing Taiwe, T. Lomberget, M. Le Borgne, J. L. Wolfender, M. De Waard, R. J. Robins and A. Boumendjel, Chem. Commun., 2015, 51, 14451–14453 Search PubMed
.
- C. Bourne, S. Roy, J. L. Wiley, B. R. Martin, B. F. Thomas, A. Mahadevan and R. K. Razdan, Bioorg. Med. Chem., 2007, 15, 7850–7864 Search PubMed
.
- G. Pratsch, G. L. Lackner and L. E. Overman, J. Org. Chem., 2015, 80, 6025–6036 Search PubMed
.
Footnote |
† Electronic supplementary information (ESI) available: Experimental procedures, characterization data, NMR spectra and computational details. See DOI: 10.1039/d1sc01889b |
|
This journal is © The Royal Society of Chemistry 2021 |