DOI:
10.1039/D1SC00127B
(Edge Article)
Chem. Sci., 2021,
12, 4981-4984
Light-induced assembly and disassembly of polymers with PdnL2n-type network junctions†
Received
8th January 2021
, Accepted 19th February 2021
First published on 5th March 2021
Abstract
Polymers containing PdnL2n complexes as network junctions were obtained by reaction of poly(ethylene glycol)-linked N-donor ligands with Pd2+. The addition of a metastable state photoacid renders the networks light sensitive, and gel–sol transitions can be achieved by irradiation with light. The inverse process, a light-induced sol–gel transition, was realized by using a molecularly defined Pd complex as an acid-sensitive reservoir for Pd2+. Upon irradiation, Pd2+ ions are released, allowing the formation of an acid-resistant polymer network. Both the gel–sol and the sol–gel transitions are reversed in the dark.
Introduction
Polymer networks can be obtained by covalent interconnection of discrete metal–organic assemblies (macrocycles or cages) with organic linkers.1,2 Two main synthetic strategies have been explored in this context: (a) the post-synthetic cross-linking of assemblies featuring reactive sites/groups in their periphery,3–7 and (b) the utilization of polymer-linked ligands during the self-assembly process.8–11 The incorporation of metal-based assemblies into polymer networks can lead to materials with interesting properties. For example, a hydrogel containing tetranuclear Fe cages was found to display sorption properties, which reflect the host–guest chemistry of the Fe cage.8 Similarly, by cross-linking of porous Rh cages, it was possible to obtain materials with permanent porosity.3 The utilization of cages as nodes in polymer networks is also interesting in terms of network topology, because cage junctions can act as highly connected nodes.11 Another noteworthy feature of metal-based junctions is the fact that they can be altered or cleaved with appropriate stimuli.8–10 Light is a particularly interesting stimulus, because it allows remote manipulation with high spatial and temporal control.12 First studies about light-induced modifications of cage-containing polymer networks were recently published by Johnson and co-workers. They have shown that networks containing Cu24L24-type cages can be cleaved by photochemical reduction of the Cu(II) centers to Cu(I), resulting in a gel–sol transition.9 The inverse process could be induced by re-oxidation with O2. Furthermore, they have reported a system, where irradiation allows converting macrocyclic Pd3L6 junctions reversibly into Pd24L48 cage junctions.10 The light sensitivity was achieved by using polymeric ligands containing photochromic dithienylethene groups. Below, we describe a new approach for rendering Pd-based polymer networks photosensitive. Importantly, our approach allows both the light-induced destruction of polymer networks and the light-induced formation of polymer networks without accumulation of ‘chemical waste’.13
Results and discussion
The study described herein was inspired by our recent observation14 that the assembly of certain metal–organic cages can be controlled with light if the merocyanine-based photoacid PAH15 (Scheme 1) is added to the mixture.
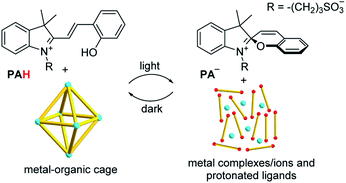 |
| Scheme 1 The photoacid PAH allows controlling the assembly of metal–organic cages. | |
Upon irradiation with blue light, PAH undergoes a ring-closure reaction, liberating a proton.16 The increased acidity can lead to the disassembly of metal–organic cages if they contain acid-sensitive metal–ligand bonds. Importantly, cages containing more basic ligands display an increased susceptibility for an acid-induced cleavage.17 In the dark, the process is reversed, and metal–ligand interactions are re-established.
In order to investigate if a photoacid can be used to control the assembly and disassembly of cage- and macrocycle-containing polymer networks, we have prepared the tetratopic N-donor ligands L1–L4 (Scheme 2). All ligands feature a poly(ethylene glycol) spacer with an average molecular weight of Mn = 4600. The design of these ligands was inspired by work of Johnson and co-workers, who have used structural analogues of L2 and L3 for the assembly of networks with Pd2L4 and Pd12L24 cage junctions.11b,c Ligand L1 features additional alkynyl spacers, which renders the terminal 3-pyridyl group less basic than the pyridyl groups of L2 and L3. Ligand L4, on the other hand, shows more basic imidazolyl donor groups (for details, see the ESI†).
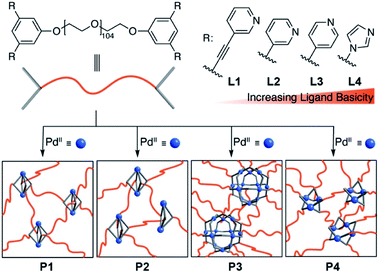 |
| Scheme 2 Polymers P1–P4 with PdnL2n-type network junctions are formed upon mixture of the N-donor ligands L1–L4 with [Pd(CH3CN)4](BF4)2 in CH3CN. | |
The geometry of the terminal pyridyl groups of L1 favors the formation of Pd2L4-type metal–organic cages.18 Upon mixing of [Pd(CH3CN)4](BF4)2 (1 equiv.) with L1 (1 equiv.) in acetonitrile, an opaque gel was immediately obtained. The mixture was then tempered at 70 °C for 4 h to ensure the formation of a polymer network (P1) with intact Pd2L4 junctions. The gels P2–P4 were prepared in an analogous fashion. Based on literature reports19–21 and our own control experiments (see the ESI†), we expected the presence of Pd2L4 links with some Pd2L3X2 defects22,23 for P2, Pd12L24 cage junctions for P3, and a mixture of macrocyclic Pd3L6 and Pd4L8 junctions for P4.
The polymers P1–P4 were characterized by NMR spectroscopy, scanning electron microscopy (SEM), and rheology measurements (for details, see ESI†). Selected data are depicted in Fig. 1. The 1H magic angle spinning (MAS) NMR spectrum of P1 shows six broad signals in the aromatic region, which are shifted downfield with respect to those of L1 (Fig. 1a). It was possible to obtain a standard solution-based 1H NMR spectrum of P1 when the reaction between L1 and [Pd(CH3CN)4](BF4)2 was performed under dilute conditions. The resulting spectrum was similar to what was obtained for the gel by MAS NMR. The SEM image of dried P1 shows a three-dimensional cross-linked structure (Fig. 1b). Rheology measurements were used to assess the mechanical properties of P1–P4. The storage modulus (G′) increases in the order P2 < P1 < P3 < P4. Two factors are expected to contribute to the observed differences: the cross-link density,10,11 and the strength of the metal–ligand bond. The latter is related to the basicity of the ligands. An alternative measure for the donor strengths of a ligand is the Huynh Electronic Parameter (HEP).24 We have determined the HEP parameter for close analogues of L1–L4 (lacking the polymer linker), and the data confirmed that the relative donor capability of the ligands is in line with the ligand basicity (see the ESI†). The high G′ value observed for P4 is probably a reflection of the strong Pd–N bonds in the Pd3L6 and Pd4L8 assemblies. On the other hand, the donor strength of the ligand can be compensated by other factors (e.g. anion effects),23 as evidenced by the higher G′ value of P1 when compared to what is found for P2.
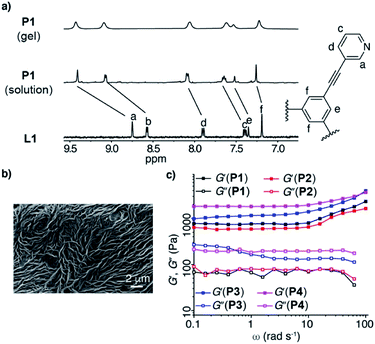 |
| Fig. 1 (a) Aromatic region of the 1H NMR spectra (400 MHz, CD3CN) of L1 (bottom), P1 in dilute solution (middle), and 1H MAS NMR spectrum of gel P1 (top); (b) SEM image of P1; (c) rheology data for P1–P4. | |
The acid sensitivity of P1–P4 was examined with trifluoroacetic acid (TFA). After addition of TFA (4 equiv. per N-donor group) and heating at 70 °C for 1 h, a gel–sol transition was observed for P2–P4, but not for P1. The higher acid resistance of P1 is likely related to the low basicity of L1. Having established that acid-induced gel–sol transitions are possible, we subsequently performed test experiments with photoacid PAH. Unfortunately, PAH was not sufficiently soluble in CH3CN to perform photoswitching experiments. Therefore, we have prepared the new photoacid PAH′(BF4) featuring a neutral –(CH2)2–OH side chain instead of the anionic –(CH2)3–SO3− side chain of PAH (Fig. 2a). PAH′(BF4) turned out to be soluble in CH3CN.
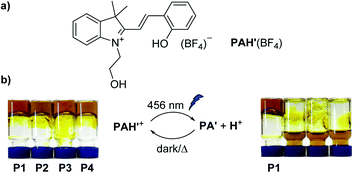 |
| Fig. 2 (a) Structure of the photoacid PAH′(BF4), and (b) reversible gel–sol transition of the polymer networks P2–P4. Polymer P1 contains acid-resistant network junctions, and is therefore not affected by light. | |
An excess of PAH′(BF4) was added to the respective gel (≥2 equiv. per donor group), and the mixture was homogenized by agitation with a vortex mixer. When the samples were irradiated with blue light (456 nm) for 1 h, we observed a similar behavior as for TFA additions: all gels were transformed to solutions except P1. The gel–sol transition could be reversed by heating the solutions in the dark for 2 h. The cycle was repeated another 4 times without noticeable difference in behavior.
The results obtained with PAH′(BF4) showed that it is possible to use a photoacid for the light-triggered disassembly of polymers with PdnL2n network junctions. Next, we set out to explore a more challenging task: the acid-induced assembly of Pd-based networks. In view of the good acid resistance of P1, we hypothesized that it might be possible to achieve the assembly of P1 from L1 and Pd2+ under acidic conditions. The prerequisite would be a source of Pd, which would liberate Pd2+ only under acidic conditions.
Previously, we had shown that metalloligand L5 (Fig. 3) forms hexanuclear Pd complexes of the formula [Pd6(L5)12]12+.25 The two pyridyl groups of L5 are highly basic, rendering the Pd assembly sensitive to acid.14 Consequently, [Pd6(L5)12]12+ represents a reservoir for Pd2+, with metal ions being released under acidic conditions.
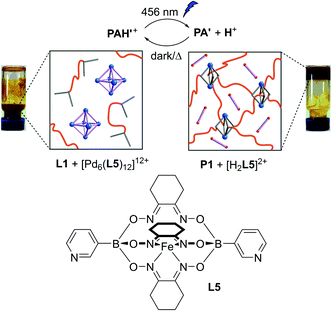 |
| Fig. 3 Light-induced assembly of the Pd-based polymer network P1 is achieved by irradiation of a mixture of L1, [Pd6(L5)12]12+, and PAH′(BF4). The hexanuclear complex [Pd6(L5)12]12+ acts as an acid-sensitive reservoir for Pd2+, enabling the formation of P1 under acidic conditions. The sol–gel transition can be reversed by heating the sample in the dark. | |
When a mixture of L1 (1 equiv.), L5 (2 equiv.) and [Pd(CH3CN)4](BF4)2 (1 equiv.) in CD3CN was equilibrated at 70 °C for 3 h, a dark red solution was obtained. Inspection of this solution by NMR spectroscopy revealed the formation [Pd6(L5)12]12+ along with ‘free’ polymer ligand L1. The preferential complexation of L5 over L1 can be explained by the higher donor strength of the former. When TFA (2 equiv. per total N-donor groups) was added, the mixture gradually converted to a gel when annealed at 70 °C for 2 h.
Next, we have performed a similar experiment using photoacid PAH′(BF4) instead of TFA. After irradiation of the sample with blue light at 50 °C for 11 h, we observed the formation of a gel (Fig. 3). The network could be cleaved by keeping the sample in the dark at 70 °C for 7 h. A light induced sol–gel transition followed by a heat-induced gel–sol transition cycle could be repeated 4 times without noticeable difference.
Conclusions
To conclude, we have demonstrated that the photoacid PAH′(BF4) can be used for the light-controlled assembly and disassembly of Pd-based polymers networks. Light-induced gel–sol transitions were achieved by using acid-sensitive PdnL2n network junctions. For realizing the inverse process, the light-induced formation of a metallogel, we have employed a hexanuclear Pd cage as an acid-sensitive reservoir for Pd2+. The relative basicity of the polymeric N-donor ligand is a key parameter for both processes, because the ligand basicity controls the acid-sensitivity of the polymer network. Another important factor is the pH range, which can be accessed with the photoacid. The metastable state acidity of PAH′(BF4) is suited to cleave Pd–N bonds. In principle, it should be possible to use photoacids for the light-controlled cleavage of other types of metal–ligand assemblies. However, it might be necessary to use – or develop – photoacids with different properties in order to achieve a reversible cleavage of the respective metal–ligand bond. Investigations in this direction are ongoing in our laboratory.
Conflicts of interest
There are no conflicts to declare.
Acknowledgements
The work was supported by the Swiss National Science Foundation (SNSF “Ambizione” PZ00P2_180008), and by the École Polytechnique Fédérale de Lausanne (EPFL). We thank Dr Daniel Ortiz for measuring mass spectra and Dr Claudia Avalos for help with MAS NMR spectroscopy measurements.
References
-
(a) P. Sutar and T. K. Maji, Dalton Trans., 2020, 49, 7658–7672 RSC;
(b) V. J. Pastore and T. R. Cook, Chem. Mater., 2020, 32, 3680–3700 CrossRef CAS;
(c) Y. Gu, J. Zhao and J. A. Johnson, Angew. Chem., Int. Ed., 2020, 59, 5022–5049 CrossRef CAS PubMed;
(d) K. C. Bentz and S. M. Cohen, Angew. Chem., Int. Ed., 2018, 57, 14992–15001 CrossRef CAS.
- The interconnection of metal-based macrocycles and cages via non-covalent interactions has also been explored. For reviews, see:
(a) B. Li, T. He, Y. Fan, X. Yuan, H. Qiu and S. Yin, Chem. Commun., 2019, 55, 8036–8059 RSC;
(b) Y. Sun, C. Chen and P. J. Stang, Acc. Chem. Res., 2019, 52, 802–817 CrossRef CAS PubMed;
(c) L. J. Chen and H. B. Yang, Acc. Chem. Res., 2018, 51, 2699–2710 CrossRef CAS PubMed;
(d) S. Datta, M. L. Saha and P. J. Stang, Acc. Chem. Res., 2018, 51, 2047–2063 CrossRef CAS PubMed.
-
(a) A. Legrand, G. A. Craig, M. Bonneau, S. Minami, K. Urayama and S. Furukawa, Chem. Sci., 2019, 10, 10833–10842 RSC;
(b) A. Carné-Sánchez, G. A. Craig, P. Larpent, V. Guillerm, K. Urayama, D. Maspoch and S. Furukawa, Angew. Chem., Int. Ed., 2019, 58, 6347–6350 CrossRef PubMed;
(c) A. Carné-Sánchez, G. A. Craig, P. Larpent, T. Hirose, M. Higuchi, S. Kitagawa, K. Matsuda, K. Urayama and S. Furukawa, Nat. Commun., 2018, 9, 1–8 CrossRef PubMed.
- G. Lal, M. Derakhshandeh, F. Akhtar, D. M. Spasyuk, J.-B. Lin, M. Trifkovic and G. K. H. Shimizu, J. Am. Chem. Soc., 2019, 141, 1045–1053 CrossRef CAS PubMed.
- J. Liu, W. Duan, J. Song, X. Guo, Z. Wang, X. Shi, J. Liang, J. Wang, P. Cheng, Y. Chen, M. J. Zawarotko and Z. Zhang, J. Am. Chem. Soc., 2019, 141, 12064–12070 CrossRef CAS PubMed.
- X.-Y. Xie, F. Wu, X. Liu, W.-Q. Tao, Y. Jiang, X.-Q. Liu and L.-B. Sun, Chem. Commun., 2019, 55, 6177–6180 RSC.
- S.-Q. Deng, D.-M. Li, X.-J. Mo, Y.-L. Miao, S.-L. Cai, J. Fan, W.-G. Zhang and S.-R. Zheng, ChemPlusChem, 2020 DOI:10.1002/cplu.202000570.
- J. A. Foster, R. M. Parker, A. M. Belenguer, N. Kishi, S. Sutton, C. Abell and J. R. Nitschke, J. Am. Chem. Soc., 2015, 137, 9722–9729 CrossRef CAS PubMed.
- N. J. Oldenhuis, K. P. Qin, S. Wang, H. Z. Ye, E. A. Alt, A. P. Willard, T. Van Voorhis, S. L. Craig and J. A. Johnson, Angew. Chem., Int. Ed., 2020, 59, 2784–2792 CrossRef CAS PubMed.
- Y. Gu, E. A. Alt, H. Wang, X. Li, A. P. Willard and J. A. Johnson, Nature, 2018, 560, 65–69 CrossRef CAS PubMed.
-
(a) Y. Wang, Y. Gu, E. G. Keeler, J. V. Park, R. G. Griffin and J. A. Johnson, Angew. Chem., Int. Ed., 2017, 56, 188–192 CrossRef CAS PubMed;
(b) A. V. Zhukhovitskiy, M. Zhong, E. G. Keeler, V. K. Michaelis, J. E. P. Sun, M. J. A. Hore, D. J. Pochan, R. G. Griffin, A. P. Willard and J. A. Johnson, Nat. Chem., 2016, 8, 33–41 CrossRef CAS PubMed;
(c) A. V. Zhukhovitskiy, J. Zhao, M. Zhong, E. G. Keeler, E. A. Alt, P. Teichen, R. G. Griffin, M. J. A. Hore, A. P. Willard and J. A. Johnson, Macromolecules, 2016, 49, 6896–6902 CrossRef CAS.
-
(a) L. Li, J. M. Scheiger and P. A. Levkin, Adv. Mater., 2019, 31, 1807333 CrossRef PubMed;
(b) Q. Zhang, D.-H. Qu and H. Tian, Adv. Opt. Mater., 2019, 7, 1–18 Search PubMed;
(c) E. R. Draper and D. J. Adams, Chem. Commun., 2016, 52, 8196–8206 RSC;
(d) G. L. Fiore, S. J. Rowan and C. Weder, Chem. Soc. Rev., 2013, 42, 7278–7288 RSC.
- M. Weißenfels, J. Gemena and R. Klajn, Chem, 2021, 7, 23–27 Search PubMed.
- S. M. Jansze, G. Cecot and K. Severin, Chem. Sci., 2018, 9, 4253–4257 RSC.
-
(a) Y. Liao, Acc. Chem. Res., 2017, 50, 1956–1964 CrossRef CAS PubMed;
(b) Z. Shi, P. Peng, D. Strohecker and Y. Liao, J. Am. Chem. Soc., 2011, 133, 14699–14703 CrossRef CAS PubMed.
- For a detailed mechanistic investigation of this process, see: C. Berton, D. M. Busiello, S. Zamuner, E. Solari, R. Scopelliti, F. Fadaei-Tirani, K. Severin and C. Pezzato, Chem. Sci., 2020, 11, 8547–8468 RSC.
- S. M. Jansze and K. Severin, J. Am. Chem. Soc., 2019, 141, 815–819 CrossRef CAS PubMed.
- D. P. August, G. S. Nichol and P. J. Lusby, Angew. Chem. Int. Ed., 2016, 55, 15022–15026 CrossRef CAS PubMed.
- Y. Wang, M. Zhong, J. V. Park, A. V. Zhukhovitskiy, W. Shi and J. A. Johnson, J. Am. Chem. Soc., 2016, 138, 10708–10715 CrossRef CAS PubMed.
- M. Tominaga, K. Suzuki, M. Kawano, T. Kusukawa, T. Ozeki, S. Sakamoto, K. Yamaguchi and M. Fujita, Angew. Chem., Int. Ed., 2004, 43, 5621–5625 CrossRef CAS PubMed.
- D. Samanta and P. S. Mukherjee, Chem. Eur. J., 2014, 20, 12483–12492 CrossRef CAS PubMed.
- B. Chen, J. J. Holstein, S. Horiuchi, W. G. Hiller and G. H. Clever, J. Am. Chem. Soc., 2019, 141, 8907–8913 CrossRef CAS PubMed.
- Apparently, the formation of Pd2L4 links is compromised when using [Pd(CH3CN)4](BF)2 instead of Pd(NO3)2 (see ref. 11b). For anion effects during the formation of Pd2L4 complexes, see: T. Tateishi, S. Takahashi, A. Okazawa, V. Martí-Centelles, J. Wang, T. Kojima, P. J. Lusby, H. Sato and S. Hiraoka, J. Am. Chem. Soc., 2019, 141, 19669–19676 CrossRef CAS PubMed.
- Q. Teng and H. V. Huynh, Dalton Trans., 2017, 46, 614–627 RSC.
- M. D. Wise, J. J. Holstein, P. Pattison, C. Besnard, E. Solari, R. Scopelliti, G. Bricogne and K. Severin, Chem. Sci., 2015, 6, 1004–1010 RSC.
Footnote |
† Electronic supplementary information (ESI) available: Containing synthetic procedures and experimental details. See DOI: 10.1039/d1sc00127b |
|
This journal is © The Royal Society of Chemistry 2021 |