DOI:
10.1039/D0SC06133F
(Edge Article)
Chem. Sci., 2021,
12, 1240-1244
A protecting group strategy to access stable lacunary polyoxomolybdates for introducing multinuclear metal clusters†
Received
7th November 2020
, Accepted 9th December 2020
First published on 13th January 2021
Abstract
Although metal-containing polyoxomolybdates (molybdenum oxide clusters) exhibit outstanding catalytic properties, their precise synthetic method has not yet been developed. This is mainly because the very low stability of the multivacant lacunary polyoxomolybdates limited their use as synthetic precursors. Here, we present a “protecting group strategy” in polyoxometalate synthesis and successfully develop an efficient method for synthesising multinuclear metal-containing polyoxomolybdates using pyridine as a protecting group for unstable trivacant lacunary Keggin-type polyoxomolybdate [PMo9O34]9−. Specifically, tetranuclear cubane- and planar-type manganese clusters were selectively synthesised in the polyoxomolybdates using the present method. The importance of this work is that, in addition to being the first practical way of utilizing multivacant lacunary polyoxomolybdates as precursors, this new “protecting group strategy” will make it possible to produce polyoxometalates with unexplored structures and properties.
Introduction
Polyoxometalates (POMs) are attractive nanosized anionic metal oxide clusters that exhibit large structural diversity and have found applications in various fields including catalysis, analytical chemistry, medicine and optical materials.1,2 Their properties, such as redox potentials and acidities, can be finely controlled by selecting their structures, constituent elements and oxidation states. In addition, the introduction of transition metals into POMs allows control of these properties and/or achieve synergetic properties that cannot be realised by POM skeletons alone. Among POMs, “polyoxomolybdates” (molybdenum oxide clusters) showed outstanding catalytic and electrochemical properties.3 Typically, the catalytic activities of polyoxomolybdates in oxidation reactions are superior to those of tungsten-based analogues. For example, vanadium-containing polyoxomolybdates exhibited higher catalytic activity for oxidative transformations of various organic compounds than their corresponding polyoxotungstates.4 The partial doping of Mo atoms into Weakley-type cobalt-containing polyoxotungstates can greatly improve their catalytic properties in water oxidation reaction.5
The controlled synthesis of multinuclear transition metal-containing “polyoxotungstates” has been achieved using multivacant lacunary “polyoxotungstates” as inorganic ligands (or templates).6 For example, our group has developed a powerful method for the use of metastable multivacant lacunary polyoxotungstates in organic solutions. By introducing metal atoms into lacunary POMs in a controlled manner, we synthesised various metal-containing polyoxotungstates with unique catalytic, photocatalytic and magnetic properties.7 While considerable efforts have been made in POM synthesis, the precise synthesis of multinuclear transition metal-containing “polyoxomolybdates” has not yet been achieved. Typically, metal-containing polyoxomolybdates have been synthesised by the one-step reaction of Mo atoms, heteroatoms (e.g. P, Si, Ge) and target metals (e.g. V) in aqueous solution (Fig. 1a).8 However, using this method, several structural isomers were formed, and it is difficult to control the position and number of introduced metals in polyoxomolybdates. Because lacunary polyoxomolybdates are extremely unstable, the reaction of multivacant lacunary polyoxomolybdates in aqueous solution typically results in an unexpected transformation into monovacant structures or fully occupied Keggin and Wells–Dawson type structures (Fig. 1b).2e,6b,9 Therefore, the development of synthetic method for the controlled introduction of transition metals into lacunary polyoxomolybdates is undoubtedly both challenging and highly demanding.
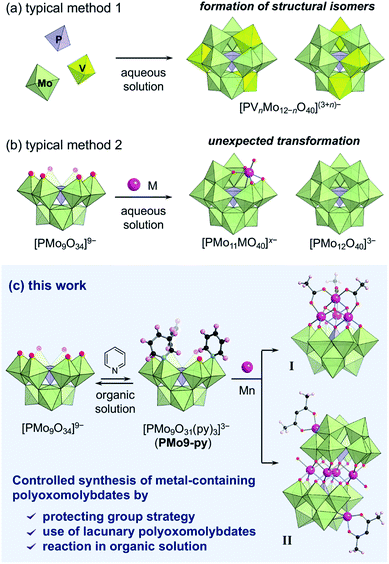 |
| Fig. 1 Schematic diagram of the synthetic method of metal-containing polyoxomolybdates: (a) conventional one-step synthesis of phosphovanadomolybdates; (b) reaction of trivacant lacunary polyoxomolybdates and metal cations in aqueous solution. (c) This work: protecting group strategy for the selective synthesis of metal-containing polyoxomolybdates using a pyridine-protected trivacant lacunary polyoxomolybdate in organic solution. | |
Recently, we determined that the metastable Keggin-type trivacant lacunary phosphomolybdate can be considerably stabilised by simply coordinating pyridine molecules at the vacant sites, and it can be isolated as [PMo9O31(py)3]3− (py = pyridyl, PMo9-py).10 The pyridyl groups can protect the highly reactive vacant sites and suppress the unexpected structure transformation and dimerisation of lacunary species. Notably, the introduced pyridyl groups are moderately labile; therefore, PMo9-py can be used as a precursor for the synthesis of POM–organic hybrid architectures. Based on these results, here we envisioned that PMo9-py is a promising precursor for the controlled synthesis of metal-containing polyoxomolybdates.
In this study, we present a “protecting group strategy” in POM synthesis and establish methods for the synthesis of multinuclear metal clusters using the pyridine-protected A-α-Keggin-type trivacant lacunary polyoxomolybdate PMo9-py as a precursor. By reacting PMo9-py with manganese species in organic solution, two types of multinuclear manganese clusters [i.e. tetranuclear cubane- (I) and a planar-type (II) manganese clusters] were selectively synthesised (Fig. 1c). This method fully utilised PMo9-py as a well-defined precursor and performed the synthesis in organic solution, which avoided the complex equilibria in aqueous media. Pyridine molecules acted as protecting groups of unstable lacunary polyoxomolybdates for the selective introduction of multinuclear metal clusters. To our knowledge, this is the first report of a successful synthesis of multinuclear transition metal clusters using multivacant lacunary polyoxomolybdates.
Results and discussion
Synthesis of a tetranuclear cubane-type manganese cluster
First, we investigated the reaction of trivacant lacunary polyoxomolybdate TPP3H6[PMo9O34] (TPP = tetraphenylphosphonium)10 and Mn(OAc)3 (OAc = acetate) in organic solution such as acetonitrile and acetone. However, owing to its low stability even in organic solution, cold-spray ionisation (CSI)-mass spectrometry revealed that the structure transformation of [PMo9O34]9− promptly proceeded to form a complex mixture including a fully occupied Keggin-type structure [PMo12O40]3−, a mononuclear manganese-containing structure [PMo11MnO39]4−, and other unidentified species (Fig S1a†). In contrast, when the pyridine-coordinating trivacant lacunary Keggin-type polyoxomolybdate [PMo9O31(py)3]3− (PMo9-py) and Mn(OAc)3 were reacted in a mixture of dichloromethane and acetonitrile (1/1, v/v) at 0 °C for 2 h, the CSI-mass spectrum showed a set of signals centred at m/z = 2902.9 that were assignable to [TPP3H1PMo9O37Mn4(CH3COO)3]− (theoretical m/z: 2902.7, Fig. S1b†), which supported the formation of the cubane-type structure I. Additionally, brown crystals of I suitable for crystallographic analysis were obtained from the solution (see ESI† for details). The X-ray crystallographic analysis revealed that the anion structure of I was a monomeric structure that consisted of four Mn atoms, where three Mn atoms occupied the vacant sites of a [B-α-PMo9O34]9− unit, and the other Mn atom was stranded on the top of three Mn atoms (Fig. 2a, S2 and Tables S1–S3†). These Mn atoms were bridged by three acetate ligands, which formed a {Mn4O3(OAc)3} cubane-like core.11 These results showed that pyridyl ligands successfully acted as protecting groups for the unstable lacunary polyoxomolybdate and they prevented the structure transformation of lacunary polyoxomolybdate frameworks. Although cubane-type structures in Dawson- and Keggin-type polyoxotungstates as well as polyoxovanadates have been reported,12 this is the first report of cubane-type structure in polyoxomolybdates.
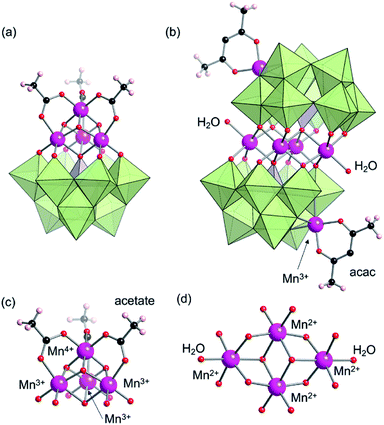 |
| Fig. 2 Crystal structures of the anion parts of (a) I and (b) II. The bottom figures are the selected views of manganese oxide clusters of (c) I and (d) II. Magenta, black, pink and red spheres represent Mn, C, H and O atoms, respectively; light green and light purple polyhedra represent {MoO6} and {PO4}, respectively. | |
The bond valence sum (BVS) values of the three Mn atoms on the vacant sites were in the range of 3.03–3.05, while that of the Mn atom on the top was 4.02, which showed the mixed-valence cubane-type structure {Mn3+3Mn4+O3(OAc)3} (Fig. 2c and Table S2†). The BVS values of Mo atoms (6.00–6.17) and P atom (4.95) revealed that their valences were +6 and +5, respectively. The Mn4+ atom was likely formed by aerobic oxidation during the reaction in air. The average distance between three Mn3+ atoms and Mn4+ atom at the top is 2.787 Å (Table S3†). Considering that the distances in previously reported cubane-type structures in polyoxotungstates were in the range of 2.801–2.837 Å, Mn4+ at the top of the core of I is slightly more closely positioned to the {PMo9} unit (Table S6†). The electrospray ionisation (ESI)-mass spectrum of I in acetonitrile showed signal sets centred at m/z = 1960.257, which were assignable to [TPP6HPMo9O37Mn4(OAc)3]2+ (theoretical m/z: 1960.262, Fig. 3a). The ESI-mass spectrum indicated that the anion structure of I was stable and remained in solution. By combining the X-ray crystallographic analysis, ESI mass spectrum, cyclic voltammogram, TG analysis (Fig. S4a†) and elemental analysis, the formula of I was revealed as TPP3H2[Mn3+3Mn4+O3(OAc)3(B-α-PMo9O34)]·(H2O)·(CH3CN).
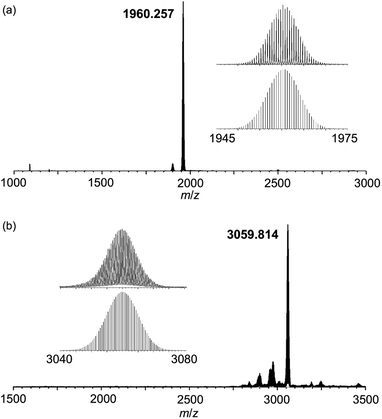 |
| Fig. 3 Positive-ion ESI-mass spectra of (a) I and (b) II in acetonitrile. Insets: (a) spectrum in the range of m/z 1945–1975, and simulated patterns for [TPP6HPMo9O37Mn4(OAc)3]2+ (m/z: 1960.262); (b) spectrum in the range of m/z 3040–3080 and simulated patterns for [TPP8{Mn(acac)}2Mn4(PMo9O34)2]2+ (m/z: 3059.837). | |
Synthesis of a tetranuclear planar-type manganese cluster
Besides the cubane-type structure, another important structure in multinuclear transition metal-containing POMs is the Weakley-type structure with planar-type tetranuclear cores.1,2,13 During the formation of the structure of I, bridging acetate ligands may have prevented the further dimerisation into a Weakley-type structure. Therefore, the type of ligands of manganese precursors may be essential in controlling the structures of products. As expected, we successfully synthesised a Weakley-type structure by reacting PMo9-py and Mn(acac)2 (acac = acetylacetonate) in 1,2-dichloroethane at 50 °C for 2 h (see ESI† for details). X-ray crystallographic analysis revealed that the anion structure of II consisted of six Mn atoms and two [B-α-PMo9O34]9− units (Fig. 2b, S3, Tables S1, S4 and S5†). Four Mn atoms in a planar configuration formed a {Mn4O14(H2O)2} core and the core was sandwiched between two [B-α-PMo9O34]9− units, which was identical to the Weakley-type structures.14,15 Two {Mn(acac)} units capped the edge of each [B-α-PMo9O34]9− unit. The BVS of Mo atoms (5.96–6.08) and P atoms (4.70) indicated the respective valences of +6 and +5 (Table S4†). The BVS values of sandwiched tetranuclear Mn atoms were in the range of 2.04–2.09, which indicated that the valences of sandwiched tetranuclear Mn atoms were +2, which was similar to the case in other Mn-containing Weakley-type structures. The Mn atoms in two {Mn(acac)} units showed BVS values of 3.08 (Table S4†). These results indicated that manganese atoms in the capping {Mn(acac)} units were oxidised from Mn2+ to Mn3+ during the reaction, possibly by O2 in air. This conclusion is supported by the fact that when the reaction was performed under an Ar atmosphere, the crystals with a Weakley-type structure were not obtained. The bond length of Mn2–O7 was 2.239 Å, which was slightly longer than that of other similar structures (Fig. 2c and Table S5†). According to BVS calculations, the two terminal O atoms on the sandwiched Mn atoms were aqua (H2O) ligands, judging by the BVS value of 0.28 (Fig. 2d and Table S4†). Although few Weakley-type polyoxomolybdates have been reported, all of them were synthesised by hydrothermal reactions of simple inorganic compounds;15a,b,c thus, the use of multivacant lacunary polyoxomolybdates as precursors is unprecedented.
The ESI-mass spectrum of II in acetonitrile showed signal sets centred at m/z = 3059.814, which were assignable to [TPP8{Mn(acac)}2Mn4(PMo9O34)2]2+ (theoretical m/z: 3059.837, Fig. 3b). Two aqua ligands on the edge of the sandwiched tetranuclear Mn core were dissociated from the POM framework under the ESI-mass measurement conditions. The ESI-mass spectrum revealed that the anion structure of II was stable and remained in solution. Therefore, we investigated the electrochemical properties of II. The cyclic voltammogram (0.5 mM II, 100 mM TBAClO4 in CH3CN, TBA = tetra-n-butylammonium, Fig. S5b†) showed three pairs of quasi-reversible peaks, with oxidation peaks observed at 0.25, 0.50 and 0.88 V versus Ag/Ag+, respectively. These redox pairs can be assigned to Mn2+/Mn3+, Mn2+/Mn3+, and Mn3+/Mn4+ ([M(acac)]2+), respectively. By combining the X-ray crystallographic analysis, ESI-mass spectrum, cyclic voltammogram, TG analysis (Fig. S4b†) and elemental analysis, it was revealed that the formula of II was TPP5.5H0.5[{Mn3+(acac)}2Mn2+4(H2O)2(B-α-PMo9O34)2]·(C2H4Cl2)·3(H2O).
Conclusions
In conclusion, we developed a new method of synthesising POMs by employing a “protecting group strategy” for unstable lacunary POMs. Specifically, using a pyridine-protected multivacant lacunary Keggin-type polyoxomolybdate as a precursor in organic solution, we overcame the low stability of lacunary polyoxomolybdates, which enabled the successful synthesis of two types of tetranuclear Mn clusters, i.e. cubane- (I) and planar-type (II) structures. These two Mn clusters possess structures that are similar to the previously reported structures on polyoxotungstates, which exhibited high catalytic activity in water oxidation or single-molecule magnetic properties.10,11 The synthetic method developed in this report represents the first practical way of utilising multivacant lacunary polyoxomolybdates as precursors. We believe that this new synthesis method will take POM synthesis to the next stage to produce POMs with unexplored structures and properties.
Conflicts of interest
There are no conflicts to declare.
Acknowledgements
We gratefully acknowledge financial support from JST PRESTO Grant Number JPMJPR18T7, JST CREST Grant Number JPMJCR17P4. JSPS KAKENHI Grant Numbers 20H02749, 20H04659 and the JSPS Core-to-Core program.
Notes and references
-
(a)
M. T. Pope, Heteropoly and Isopoly Oxometalates, Springer, Berlin, 1983 CrossRef
;
(b) C. L. Hill and C. M. Prosser-McCartha, Coord. Chem. Rev., 1995, 143, 407 CrossRef CAS
;
(c)
C. L. Hill in Comprehensive Coordination Chemistry II, ed. J. A. McCleverty and T. J. Meyer, Elsevier Pergamon, Amsterdam, 2004, vol. 4, p. 679 Search PubMed
;
(d) H. N. Miras, J. Yan, D.-L. Long and L. Cronin, Chem. Soc. Rev., 2012, 41, 7403 RSC
;
(e) O. Oms, A. Dolbecq and P. Mialane, Chem. Soc. Rev., 2012, 41, 7497 RSC
;
(f)
Advances in Inorganic Chemistry, ed. R. van Eldik and L. Cronin, Elsevier Academic Press, Amsterdam, 2017, vol. 69 Search PubMed
;
(g) N. I. Gumerova and A. Rompel, Nat. Rev. Chem., 2018, 2, 0112 CrossRef CAS
.
-
(a) N. Mizuno and M. Misono, Chem. Rev., 1998, 98, 199 CrossRef CAS
;
(b) M. Sadakane and E. Steckhan, Chem. Rev., 1998, 98, 219 CrossRef CAS
;
(c) U. Kortz, A. Müller, J. Van Slageren, J. Schnack, N. S. Dalal and M. Dressel, Coord. Chem. Rev., 2009, 253, 2315 CrossRef CAS
;
(d) C. Streb, Dalton Trans., 2012, 41, 1651 RSC
;
(e) H.-J. Lv, Y. V. Geletii, C.-C. Zhao, J. W. Vickers, G.-B. Zhu, Z. Luo, J. Song, T.-Q. Lian, D. G. Musaev and C. L. Hill, Chem. Soc. Rev., 2012, 41, 7572 RSC
;
(f) Y. Zhou, G.-J. Chen, Z.-Y. Long and J. Wang, RSC Adv., 2014, 4, 42092 RSC
;
(g) S.-S. Wang and G.-Y. Yang, Chem. Rev., 2015, 115, 4893 CrossRef CAS
;
(h) I. A. Weinstock, R. E. Schreiber and R. Neumann, Chem. Rev., 2018, 118, 2680 CrossRef CAS
;
(i) K. Suzuki, N. Mizuno and K. Yamaguchi, ACS Catal., 2018, 8, 10809 CrossRef CAS
.
-
(a) A. M. Khenkin, G. Leitus and R. Neumann, J. Am. Chem. Soc., 2010, 132, 11446 CrossRef CAS
;
(b) B. Nohra, H. E. Moll, L. M. R. Albelo, P. Mialane, J. Marrot, C. Mellot-Draznieks, M. O'Keeffe, R. N. Biboum, J. Lemaire, B. Keita, L. Nadjo and A. Dolbecq, J. Am. Chem. Soc., 2011, 133, 13363 CrossRef CAS
;
(c) J.-S. Qin, D.-Y. Du, W. Guan, X.-J. Bo, Y.-F. Li, L.-P. Guo, Z.-M. Su, Y.-Y. Wang, Y.-Q. Lan and H.-C. Zhou, J. Am. Chem. Soc., 2015, 137, 7169 CrossRef CAS
;
(d) J. Lehmann, A. Gaita-Arin
o, E. Coronado and D. Loss, Nat. Nanotechnol., 2007, 2, 312 CrossRef CAS
;
(e) V. Prabhakaran, B. L. Mehdi, J. J. Ditto, M. H. Engelhard, B.-B. Wang, K. D. D. Gunaratne, D. C. Johnson, N. D. Browning, G. E. Johnson and J. Laskin, Nat. Commun., 2016, 7, 11399 CrossRef CAS
;
(f) N. Kawasaki, H. Wang, R. Nakanishi, S. Hamanaka, R. Kitaura, H. Shinohara, T. Yokoyama, H. Yoshikawa and K. Awaga, Angew. Chem., Int. Ed., 2011, 50, 3471 CrossRef CAS
;
(g) H. Tanaka, M. Akai-Kasaya, A. TermehYousefi, L. Hong, L.-X. Fu, H. Tamukoh, D. Tanaka, T. Asai and T. Ogawa, Nat. Commun., 2018, 9, 2693 CrossRef
;
(h) Y.-R. Wang, Q. Huang, C.-T. He, Y.-F. Chen, J. Liu, F.-C. Shen and Y.-Q. Lan, Nat. Commun., 2018, 9, 4466 CrossRef
.
-
(a) K. Nomiya, K. Yagishita, Y. Nemoto and T. Kamataki, J. Mol. Catal. A: Chem., 1997, 126, 43 CrossRef CAS
;
(b) S. Shinachi, H. Yahiro, K. Yamaguchi and N. Mizuno, Chem.–Eur. J., 2004, 10, 6489 CrossRef CAS
;
(c) J. Das and K. M. Parida, J. Mol. Catal. A: Chem., 2007, 264, 248 CrossRef CAS
;
(d) K. Yajima, K. Yamaguchi and N. Mizuno, Chem. Commun., 2014, 50, 6748 RSC
;
(e) K. Yamaguchi, N. Xu, X. Jin, K. Suzuki and N. Mizuno, Chem. Commun., 2015, 51, 10034 RSC
;
(f) C. Li, K. Suzuki, K. Yamaguchi and N. Mizuno, New J. Chem., 2017, 41, 1417 RSC
.
- M. Martin-Sabi
, J. Soriano-López, R. S. Winter, J.-J. Chen, L. Vilà-Nadal, D.-L. Long
, J. R. Galán-Mascarós
and L. Cronin, Nat. Catal., 2018, 1, 208 CrossRef
.
-
(a) A. Proust, R. Thouvenot and P. Gouzerh, Chem. Commun., 2008, 16, 1837 RSC
;
(b) A. Dolbecq, E. Dumas, C. R. Mayer and P. Mialane, Chem. Rev., 2010, 110, 6009 CrossRef CAS
;
(c) A. Proust, B. Matt, R. Villanneau, G. Guillemot, P. Gouzerh and G. Izzet, Chem. Soc. Rev., 2012, 41, 7605 RSC
;
(d) K. Kastner, J. T. Margraf, T. Clark and C. Streb, Chem.–Eur. J., 2014, 20, 12269 CrossRef CAS
;
(e) K. Kastner, J. Forster, H. Ida, G. N. Newton, H. Oshio and C. Streb, Chem.–Eur. J., 2015, 21, 7686 CrossRef CAS
.
-
(a) T. Minato, K. Suzuki, K. Yamaguchi and N. Mizuno, Angew. Chem., Int. Ed., 2016, 55, 9630 CrossRef CAS
;
(b) K. Suzuki, R. Sato and N. Mizuno, Chem. Sci., 2013, 4, 596 RSC
;
(c) K. Suzuki, F. Tang, Y. Kikukawa, K. Yamaguchi and N. Mizuno, Angew. Chem., Int. Ed., 2014, 53, 5356 CrossRef CAS
;
(d) T. Minato, K. Suzuki, Y. Ohata, K. Yamaguchi and N. Mizuno, Chem. Commun., 2017, 53, 7533 RSC
;
(e) T. Minato, K. Suzuki, K. Yamaguchi and N. Mizuno, Chem.–Eur. J., 2017, 23, 14213 CrossRef CAS
;
(f) T. Minato, Y. Ohata, K. Ishii, K. Yamaguchi, N. Mizuno and K. Suzuki, J. Mater. Chem. C, 2019, 7, 12918 RSC
;
(g) K. Yonesato, H. Ito, H. Itakura, D. Yokogawa, T. Kikuchi, N. Mizuno, K. Yamaguchi and K. Suzuki, J. Am. Chem. Soc., 2019, 141, 19550 CrossRef CAS
;
(h) K. Yonesato, H. Ito, D. Yokogawa, K. Yamaguchi and K. Suzuki, Angew. Chem., Int. Ed., 2020, 59, 16361 CrossRef CAS
.
- V. F. Odyakov, E. G. Zhizhina, Y. A. Rodikova and L. L. Gogin, Eur. J. Inorg. Chem., 2015, 3618 CrossRef CAS
.
- Polyoxomolybdates typically show complex equilibria in aqueous media, where various species, such as [P2Mo5O23]6−, [PMo6O25]9−, [A-α-PMo9O34]9−, [PMo11O39]7−, [PMo12O40]3−, and [P2Mo18O62]6−, have been identified in an equilibrium mixture in aqueous solvents:
(a) J. A. Rob van Veen, O. Sudmeijer, C. A. Emeis and H. de Wit, J. Chem. Soc. Dalton Trans., 1986, 1825 RSC
;
(b) L. Pettersson, I. Andersson and L. Óhman, Inorg. Chem., 1986, 25, 4726 CrossRef CAS
;
(c) C. Marchal-Roch, E. Ayrault, L. Lisnard, J. Marrot, F.-X. Liu and F. Sécheresse, J. Cluster Sci., 2006, 17, 283 CrossRef CAS
;
(d) F. Li and L. Xu, Dalton Trans., 2011, 40, 4024 RSC
.
- C. Li, N. Mizuno, K. Yamaguchi and K. Suzuki, J. Am. Chem. Soc., 2019, 141, 7687 CrossRef CAS
.
-
(a) M. W. Wemple, H.-L. Tsai, K. Folting, D. N. Hendrickson and G. Christou, J. Am. Chem. Soc., 1995, 117, 7275 CrossRef CAS
;
(b) M. W. Wemple, D. M. Adams, K. S. Hagen, K. Folting, D. N. Hendrickson and G. Christou, J. Chem. Soc., Chem. Commun., 1995, 1591 RSC
;
(c) G. Aromí, M. W. Wemple, J. S. Aubin, K. Folting, D. N. Hendrickson and G. Christou, J. Am. Chem. Soc., 1998, 120, 5850 CrossRef
;
(d) Z.-J. Liu, X.-L. Wang, C. Qin, Z.-M. Zhang, Y.-G. Li, W.-L. Chen and E.-B. Wang, Coord. Chem. Rev., 2016, 313, 94 CrossRef CAS
.
-
(a) X. Fang, M. Speldrich, H. Schilder, R. Cao, K. P. O'Halloran, C. L. Hill and P. Kögerler, Chem. Commun., 2010, 46, 2760 RSC
;
(b) R. Al-Oweini, B. S. Bassil, J. Friedl, V. Kottisch, M. Ibrahim, M. Asano, B. Keita, G. Novitchi, Y. Lan, A. Powell, U. Stimming and U. Kortz, Inorg. Chem., 2014, 53, 5663 CrossRef CAS
;
(c) B. Schwarz, J. Forster, M. K. Goetz, D. Yîcel, C. Berger, T. Jacob and C. Streb, Angew. Chem., Int. Ed., 2016, 55, 6329 CrossRef CAS
.
- T. J. R. Weakley, H. T. Evans, J. S. Showell, G. F. Tourńe and C. M. J. Tourńe, J. Chem. Soc., Chem. Commun., 1973, 139 RSC
.
-
(a) C. J. Gomez-Garcia, E. Coronado, P. Gomez-Romero and N. Casa-Pastor, Inorg. Chem., 1993, 32, 3378 CrossRef CAS
;
(b) U. Kortz, S. Isber, M. H. Dickman and D. Ravot, Inorg. Chem., 2000, 39, 2915 CrossRef CAS
;
(c) U. Kortz, S. Nellutla, A. C. Stowe, N. S. Dalal, U. Rauwald, W. Danquah and D. Ravot, Inorg. Chem., 2004, 43, 2308 CrossRef CAS
;
(d) H. Lv, J. Song, H. Zhu, Y. V. Geletii, J. Bacsa, C. Zhao, T. Lian, D. G. Musaev and C. L. Hill, J. Catal., 2013, 307, 48 CrossRef CAS
;
(e) J. Wang, P. Ma, Y. Shen and J. Niu, Cryst. Growth Des., 2008, 8, 3130 CrossRef CAS
.
-
(a) S. Li, J. Zhao, P. Ma, J. Du, J. Niu and J. Wang, Inorg. Chem., 2009, 48, 9819 CrossRef CAS
;
(b) S. Zhou, B. Liu, T. Shi, X.-M. Li and Y.-G. Chen, Inorg. Chem., 2015, 54, 7165 CrossRef CAS
;
(c) H. Xu, X.-M. Liu, T. Su, W.-C. Chen and Z.-M. Su, Dalton Trans., 2020, 49, 977 RSC
.
Footnote |
† Electronic supplementary information (ESI) available: Experimental details, Tables S1–S7, and Fig. S1–S6. CCDC 2041768 and 2041769. For ESI and crystallographic data in CIF or other electronic format see DOI: 10.1039/d0sc06133f |
|
This journal is © The Royal Society of Chemistry 2021 |