DOI:
10.1039/D1RA07159A
(Paper)
RSC Adv., 2021,
11, 38172-38188
Rapid antibacterial activity of anodized aluminum-based materials impregnated with quaternary ammonium compounds for high-touch surfaces to limit transmission of pathogenic bacteria†
Received
24th September 2021
, Accepted 22nd November 2021
First published on 26th November 2021
Abstract
Infections caused by multidrug-resistant bacteria are a major public health problem. Their transmission is strongly linked to cross contamination via inert surfaces, which can serve as reservoirs for pathogenic microorganisms. To address this problem, antibacterial materials applied to high-touch surfaces have been developed. However, reaching a rapid and lasting effectiveness under real life conditions of use remains challenging. In the present paper, hard-anodized aluminum (AA) materials impregnated with antibacterial agents (quaternary ammonium compounds (QACs) and/or nitrate silver (AgNO3)) were prepared and characterized. The thickness of the anodized layer was about 50 μm with pore diameter of 70 nm. AA with QACs and/or AgNO3 had a water contact angle varying between 45 and 70°. The antibacterial activity of the materials was determined under different experimental settings to better mimic their use, and included liquid, humid, and dry conditions. AA–QAC surfaces demonstrated excellent efficiency, killing >99.9% of bacteria in 5 min on a wide range of Gram-positive (Staphylococcus aureus, Clostridioides difficile, vancomycin-resistant Enterococcus faecium) and Gram-negative (streptomycin-resistant Salmonella typhimurium and encapsulated Klebsiella pneumoniae) pathogens. AA–QACs showed a faster antibacterial activity (from 0.25 to 5 min) compared with antibacterial copper used as a reference (from 15 min to more than 1 h). We show that to maintain their high performance, AA–QACs should be used in low humidity environments and should be cleaned with solutions composed of QACs. Altogether, AA–QAC materials constitute promising candidates to prevent the transmission of pathogenic bacteria on high-touch surfaces.
1. Introduction
Bacterial nosocomial infections represent an extremely serious global threat to human health.1–3 Drug-resistant infections are currently responsible for 700
000 deaths per year worldwide and if the current situation does not improve, this human toll might reach 10 million deaths per year by 2050.3 The direct (healthcare) and indirect (international trade, animal production) impacts of these infections also place a heavy financial burden on global systems, estimated between 1.0 and 3.4 $ billion per year.4,5 Finding new drugs to fight bacterial pathogens is urgent but developing new strategies to break the transmission chain and prevent propagation of these bacteria is also crucial.
The transmission of bacterial pathogens that cause infections is mainly linked to cross-contamination through inert surfaces and the environment.1,6–8 An interesting way to limit the spread of pathogenic bacteria, without the use of antibiotics, is to develop antibacterial materials for use in high contact areas, such as door handles, stair railings, components of public transport and other surfaces that can serve as reservoirs for microorganisms.8–10
Several strategies can be used to design antibacterial materials. One of them is to select a type of material with inherent antibacterial activity such as metal alloys, mainly based on copper, silver, or zinc.10–13 Copper-based alloys are currently the most used with over 500 materials registered as antibacterial products by the U.S. Environmental Protection Agency (EPA). They are capable of killing 99.9% of pathogenic bacteria within 2 h.14–16 However, several issues have been raised with copper-based alloys, such as durability, corrosion susceptibility, bacterial resistance selection and cost-effectiveness.17 In addition, their effectiveness to prevent cross-contamination or to reduce the incidence of healthcare-associated infections (HAI) was not based on blinded, randomized and unbiased clinical studies.18–21
Another strategy consists in applying specific coatings to acquire antibacterial surface properties (superhydrophobicity, nanostructuring) or to incorporate into the material a wide variety of known antibacterial compounds (copper, silver, quaternary ammoniums compounds (QACs), etc.).10,12,22–28 QACs are antimicrobial agents effective against a broad spectrum of Gram-positive and Gram-negative bacteria as well as several enveloped viruses, and they are widely used in cleaning products.29,30 The persistence of their antibacterial property over time and over a wide pH range, their odorless and amphiphilic properties, as well as the low cost of commercial grade QACs make them very good candidates for manufacturing biocidal surfaces.31–33 The silver ions, due to their antibacterial effect mediated by interactions and alterations of proteins and cell walls, are also used in various applications such as medical devices (central venous catheters and topical antiseptics: silver nitrate and silver sulfadiazine), textiles (clothing and bedsheets) and self-disinfecting surfaces (Surfacine™).9,12,34,35
Proper testing of antibacterial surfaces is critical to evaluate their effectiveness. Several international standards are currently used to assess the antibacterial properties of materials such as the Japanese standard (JIS Z2801: 2010), the European standard (ISO 22196: 2011), the American interim standard (US EPA: 2020) and its older standard more specific to copper surfaces (US EPA: 2015). These standards recommend testing antibacterial activity on model pathogens like Staphylococcus aureus and Escherichia coli (JIS Z2801 and ISO 22196) or Pseudomonas aeruginosa (US EPA). However, the antibacterial properties of materials are usually evaluated after long contact times such as 1–2 h to several days (e.g., 99.9% of bacteria killed within 1–2 h: EPA) under liquid conditions, not representative of the practical conditions of high touch surfaces.
In the present study, we report antibacterial activity testing of anodized aluminum-based surfaces that aim to limit the spread of pathogenic bacteria.36 The low cost of aluminum (4 times cheaper than copper) and the anodizing process, as well as its wide range of applications (industrial, commercial and consumer goods) make anodized aluminum-based materials very interesting candidates for use in high-touch surfaces.37
The antibacterial materials consist of a nanoporous surface layer impregnated with antibacterial solutions. First, the aluminum-based materials were characterized after the anodization and impregnation steps by scanning electron microscopy (SEM) and contact angle measurement. The release kinetics of the impregnated compounds were also evaluated by inductively coupled plasma optical emission spectrometry (ICP-OES) and UV spectrophotometry. The antibacterial properties of the solutions selected for impregnation of the anodized aluminum (AgNO3 and/or QACs) were verified on Gram-positive and Gram-negative bacterial pathogens posing significant clinical problems in hospitals: Staphylococcus aureus, Clostridioides difficile, vancomycin-resistant enterococci, Escherichia coli, streptomycin-resistant Salmonella and Klebsiella pneumoniae. Then, the antibacterial properties of these materials were determined in a time dependent manner using experimental approaches that we developed to better mimic real life conditions of use of these materials. Copper was used as an antibacterial effectiveness control. Finally, we evaluated the antibacterial properties and tested the durability of the materials after treatment with different cleaning products and after repeated immersions into water.
2. Experimental section
2.1. Materials
2.1.1. Surface materials. The materials used are hard-anodized aluminum surfaces (AA6061 and AA5052 alloys) impregnated with different antibacterial solutions: (i) AgNO3 (1% w/v); (ii) QACs-based (10.9% w/v) solution containing alkyldimethylbenzylammonium chloride (ADBAC), octyldecyldimethylammonium chloride, dioctyldimethylammonium chloride and didecyldimethylammonium chloride (DDAC); (iii) a combination of both AgNO3 (1% w/v) and QACs-based (10.9% w/v) solutions (Fig. S1†). This technology is patented by the company A3S (A3Surfaces, Chicoutimi, Quebec, Canada),38 who produced all anodized aluminum-based materials used in the present study. In all assays, aluminum without anodization (Al) and anodized aluminum without antibacterial solution impregnation (AA) were used as negative controls. Copper alloy C70600 (common name: CuNi10Fe1Mn) was also used as an antibacterial material control.39
2.1.2. Bacterial strains and culture media. The selected bacterial strains which represent the vast majority of problematic nosocomial and community infections nowadays and their characteristics are listed in Table S1.†3,4 Staphylococcus aureus (ATCC® 29213™) and Escherichia coli (ATCC® 29532™) were purchased from ATCC® (Manassas, VA, USA). Clostridioides difficile epidemic strain R20291 and streptomycin-resistant Salmonella enterica serovar Typhimurium SL1344 were kindly provided by Dr Trevor Lawley (Sanger Institute, United Kingdom) and the laboratory of Pr. Alfredo Menendez (Université de Sherbrooke, QC, Canada),40 respectively. Klebsiella pneumoniae, vancomycin-resistant Enterococcus faecalis (Van B) and vancomycin-resistant Enterococcus faecium (Van A) were clinical isolates obtained from the Centre Hospitalier Universitaire de Sherbrooke (CHUS), thanks to Dr Simon Lévesque (Sherbrooke, QC, Canada). Brain Heart Infusion (BHI), Tryptose–Yeast extract (TY: 3% tryptose and 2% yeast extract, pH 7.4), Luria–Bertani (LB), and Mueller–Hinton (MH) broths were purchased from BD Biosciences (Mississauga, ON, Canada). For solid media, 1% (w/v) agar was added to the above media.
2.2. Methods
2.2.1. Surface wettability. The sessile drop method was performed to measure static contact angle on aluminum and copper materials using a goniometer coupled to a camera system (First Ten Angstroms, FTA 200). Drops of distilled water controlled at 1 μL were deposited on the surfaces using a microliter syringe. All steps, from the material placement to wettability measurements using the ellipse–tangent fit method, were automated and controlled by computer via FTA32Video software. Four measurements were taken at different locations on both sides of each sample at room temperature allowing consistent assessment of the materials.
2.2.2. Characterization of the anodization layer. A cross section was made on the materials using a cutter with diamond blades from Buehler (Esslingen, Germany). Then, the materials were held vertically in a resin (EpoxyCure™2, Buehler, IL, USA) to be able to observe their cross sections. A polishing (from 1 μm to 0.05 μm) and a metallization step with a gold/palladium mixture were then carried out. Subsequently, the slices of materials were observed by scanning electron microscopy at a voltage of 5.0 kV (Hitachi SU8000, Japan).
2.2.3. QACs release kinetics from anodized aluminum materials with or without impregnation. Material samples (disks of 1 cm in diameter) consisting of AA, AA–AgNO3, AA–QACs and AA–AgNO3–QACs were immersed in 1 mL of nanopure water for a predetermined duration ranging from 30 s to 144 h. At each time point, the solution was collected, and the absorbance was measured at a wavelength of 215 nm using a UV-VIS spectrophotometer (Ultrospec 2100 pro UV-VIS Spectrophotometer, Amersham Biosciences, UK). Calibration curves obtained by measuring the absorbance at 215 nm of several dilutions of the QACs stock solution showed linear behavior and were used to calculate the concentrations of QACs released from the materials.
2.2.4. Bacteria culture. Frozen stocks of bacterial strains stored at - 80 °C in glycerol were spread out on agar plates and grown overnight under the appropriate conditions (see Table S1†). For C. difficile experiments, bacteria were manipulated and incubated under anaerobic conditions (10% hydrogen, 5% CO2 and 85% nitrogen) using an anaerobic chamber (Coy Laboratories, Grass Lake, MI, USA), and all media were pre-reduced overnight (O/N) before use. Prior to each experiment, bacterial pre-cultures inoculated from a single isolated colony were prepared in 5 mL of broth and incubated O/N at 37 °C. Then, at their logarithmic stage of growth, bacterial cells were diluted in fresh broth and the optical density at 600 nm (OD600) was determined using a portable spectrophotometer (Fisherbrand™ Cell Density Meter 40, ThermoFisher Scientific, USA) to adjust the working bacterial density.
2.2.5. Minimal inhibitory concentration (MIC) and minimal bactericidal concentration (MBC) assay. The minimum inhibitory concentration (MIC) and minimum bactericidal concentration (MBC) of each solution used for the impregnation of materials (AgNO3, QACs and AgNO3 + QACs solution) were evaluated on each bacterial strain under study. To determine the MIC, doubling dilutions of the solutions to be tested were prepared in 96-well microtiter plates. An inoculum of 100 μL of bacterial suspension (106 colony forming units (CFU) per mL) prepared as mentioned above, was added to each well containing 100 μL of antibacterial solution. Negative controls without bacterial culture and positive controls without antibacterial solution were used in parallel. The plates were incubated for 18 h at 37 °C and the OD600 was measured using a UV-VIS spectrophotometer (Synergy™ HTX Multi-Mode Microplate Reader, BioTek, USA). The lowest concentration of each impregnation solution at which no turbidity could be observed was defined as the MIC. To determine the MBC, 100 μL from each well of MIC plates showing no bacteria growth were transferred in 96-well microtiter plates to make decimal dilutions using fresh sterile broth. Subsequently, 20 μL aliquots were spotted on agar plates (spot plating assay41,42) and colonies were counted after 24 h of incubation at 37 °C. The lowest concentration of antibacterial solution for which no colony could be observed was defined to be MBC.
2.2.6. Antibacterial activity of materials using a swab liquid-inoculation assay. A swab liquid-inoculation assay was carried out to evaluate the antibacterial activity of materials (Fig. 1A). A sterile nylon swab (FLOQSwabs™, Copan, Italy) was immersed in a bacterial suspension (108 CFU mL−1) prepared as mentioned above and the excess liquid was drained. The contaminated swab was rubbed on the material sample for 5 s, allowing for standardized inoculation of 106 CFU per surface. Following a contact kinetics of 0.25, 1, 5, 15 and 60 min, the contaminated materials were immersed into 1 mL of QACs neutralization solution Casein peptone Lecithin Polysorbate Broth43,44 (Sigma-Aldrich®, MO, USA) containing 0.04% of Tween20® (Bioshop®, ON, Canada), and vortexed for 10 s. This neutralization solution did not affect the viability of bacteria. Aliquots of bacteria released from the contaminated materials in the neutralization solution were immediately transferred to a 96-well plate, serially diluted in sterile broth, and spotted on agar plates using a spot plating assay as described above. The number of CFU were counted after O/N incubation at 37 °C to determine the bacterial load present on the materials.
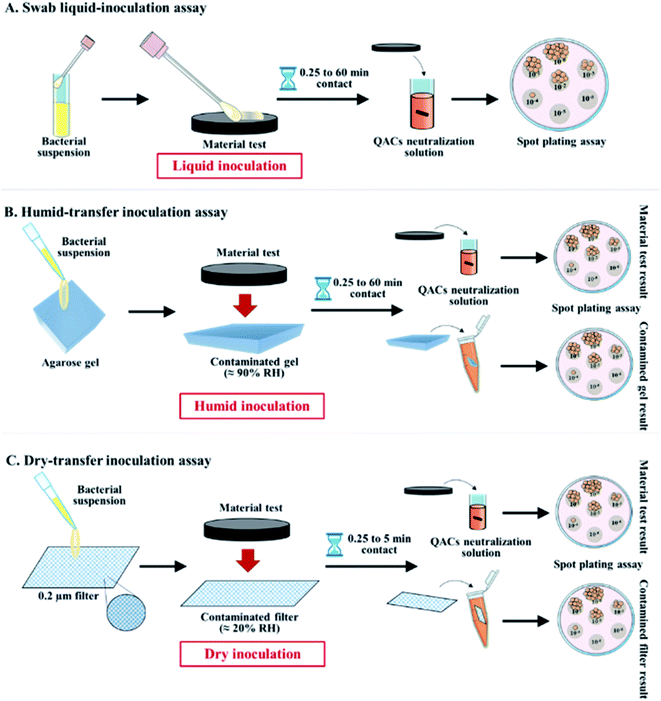 |
| Fig. 1 Schematic representation of (A) the swab liquid-inoculation assay, (B) the humid-transfer inoculation assay and (C) the dry-transfer inoculation assay. | |
2.2.7. Antibacterial activity of materials using a humid-transfer inoculation assay. A humid-transfer inoculation assay was carried out to evaluate the effectiveness of materials to prevent bacterial cross-contamination under limited water content (Fig. 1B). A 10 μL inoculum of a bacterial suspension (108 CFU mL−1) was deposited on a sterile piece of 2% (w/v) agarose gel (1 cm × 1 cm) and left for 1 h under the biological hood to obtain a final relative humidity (RH) of 89.4 ± 0.9%. The RH of the gel was measured by the drying technique in an oven maintained at 160 °C, by means of weighing the gel before and after drying (considered dry when its weight was constant in the oven). To evaluate the level of contamination of the materials resulting from the transfer of bacteria from the gel, the different materials were deposited on the contaminated gels for 0.25, 1, 5, 15 and 60 min and then the number of surviving bacteria on the materials was determined as described above. In parallel, to determine the effect of a contact with antibacterial materials on the bacteria present in the contaminated gel, the pieces of gel were transferred in 2 mL microtubes containing 1 mL of neutralization solution and four sterile 3 mm glass beads. The pieces of gel were then disrupted and homogenized for 10 min at 30 s−1 frequency using a Mixer Mill MM 400 (Retsch®, France) to release bacteria trapped in the gel, which were then quantified by spot plating assay as described above. CFUs were counted to determine the number of surviving bacteria remaining in the contaminated gel and after transfer onto the materials.
2.2.8. Antibacterial activity of materials using a dry-transfer inoculation assay. A dry-transfer inoculation assay was performed to evaluate the effectiveness of materials to prevent bacterial cross-contamination under low humidity conditions representative of the surface of the skin (Fig. 1C). Aliquots of 10 μL from a bacterial suspension (108 CFU mL−1) were deposited on 0.2 μm sterile nylon filters (Nylon membrane disk diameter 13 mm – filter 0.22 μm, GVS North America, USA). The inoculated filters were dried for 7 min under the biological hood in order to obtain a RH of 16.8 ± 2.6%, representative of the RH found in Stratum Corneum of human skin epidermis.45 The RH of the filter was measured by the oven drying technique (160 °C) as mentioned above. The different materials were deposited on the inoculated filters for 0.25, 1, 5, 15 and 60 min. Then, the number of surviving bacteria on the contaminated filter and after transfer on the materials were quantified as described above.
2.2.9. Effectiveness of bactericidal materials after washing with cleaning products. The impact of serial washes on the antibacterial properties of the materials copper, Al, AA and AA–AgNO3QACs was evaluated. The materials underwent series of 0, 5 or 10 washes using different solutions, including cleaning products commonly used in hospitals: (a) sterile nanopure water, (b) ethanol (70% v/v), (c) Virox™5 containing ≃7% v/v of hydrogen peroxide (utilization: diluted 1/16; v/v), (d) QACs-based impregnation solution (diluted 8 mL in 1 L). When applicable, each wash was performed following the procedure called “disinfection of medical devices” recommended by the cleaning companies. The washes carried out with Virox™5 (The Butcher Company, WI, USA) and the QACs solution were applied to the materials for 5 and 10 min, respectively. The product was dried in air and a water wash was carried out to eliminate the residues of cleaning products on the materials. The washes with sterile nanopure water and ethanol were performed directly, without rinsing. Following these series of washes, the antibacterial efficiency of the materials was evaluated following the swab test methodology, using S. aureus and a constant inoculum-material contact time of 1 h. In parallel, copper, Al, AA and AA–AgNO3–QACs without wash with cleaning solution were used as controls.
2.2.10. Efficiency of A3S materials following immersions in water. AA–AgNO3–QACs underwent series of 1 to 10 successive 12 h immersions in 1 mL of sterile nanopure water. Between each successive immersion, the used deionized water was replaced with 1 mL of fresh sterile nanopure water. Following these series of immersions, the antibacterial efficiency of the AA–AgNO3–QACs was evaluated with the swab liquid-inoculation methodology, using S. aureus and a constant inoculum-material contact time of 1 h. In parallel, the same experiment was repeated using AA control samples and AA–AgNO3–QACs treated samples which had not been immersed in sterile nanopure water.
2.2.11. Statistical analyses. Analysis of variance (ANOVA) and subsequent statistical tests (Tukey–Kramer studentized range post-hoc tests) were performed using Excel (Excel 2013®) and GraphPad (GraphPad Software 2020 Inc., Prism 8, San Diego, CA, USA). Only difference with a p < 0.05 were considered significant. In the current paper, the number of independent experiments is defined as “n” and the total number of technical replicates per condition is defined as “N”.
3. Results and discussion
3.1. Characterization of anodized aluminum materials
All the anodized aluminum-based materials (with or without impregnation) used in this study were prepared and provided by the company A3S (Fig S1†).
3.1.1. Surface characterization.
Hydrophobicity by contact angle measurement. Since wettability of surfaces can influence the first stage of pathogen adhesion on materials,46,47 static contact angle measurements were carried out using a goniometer to assess the surface wettability of Al, AA, AA–AgNO3, AA–QACs, AA–AgNO3–QACs and copper. As shown in Table 1, the reference materials copper and Al exhibited a slight hydrophobicity of their surface (around 78–85°), in accordance with previously published data.48–51
Table 1 Surface wettability of the materials using contact angle measurement by goniometer
Material |
Contact angle measurement in static conditiona (°) |
Significant effectb compared to |
Al |
AA |
Results are means ± SD (n = 2; N = 24). Significant effect *p < 0.01 and **p < 0.001. |
Copper |
78.05 ± 3.77 |
— |
** |
Al |
85.53 ± 2.83 |
— |
** |
AA |
48.99 ± 6.76 |
** |
— |
AA–AgNO3 |
45.96 ± 5.53 |
** |
— |
AA–QACs |
67.25 ± 4.00 |
* |
* |
AA–AgNO3–QACs |
69.37 ± 4.49 |
* |
* |
In contrast, the anodization process on Al significantly decreased the contact angle (around 49°), the AA being more hydrophilic than Al (p < 0.001). These results are in contradiction with the hydrophobic/superhydrophobic properties normally induced by the hard anodization process of aluminum materials.52–54
However, this difference was probably induced during the final stage of manufacturing AA materials by the company A3S, which consists of pore sealing process by hydration of alumina molecules on the surfaces leading to dilatation and gradual closure of the alumina oxide layer.55 Furthermore, the impregnation step with QACs modified the wettability of AA. Indeed, unlike AA–AgNO3, which showed similar contact angle in comparison with non-impregnated AA, AA–QACs partially restored the hydrophobicity lost following anodization (p < 0.001). QACs cations are composed of a positively charged nitrogen atom with four long non-polar carbon chains, which, used as a coating, can influence the surface hydrophobicity of the material.56–59 Thus, the type of solution used during the material impregnation step influences their surface wettability property. Generally, super-hydrophobic materials (θ > 150°) are recommended for antibacterial applications, since they inhibit cellular adhesion.60
Observation of the anodization layer by scanning electron microscopy. Cross sections were carried out to characterize by scanning electron microscopy the anodization layer of AA with or without AgNO3–QACs impregnation (Fig. 2). As expected, untreated aluminum Al did not show any layer at its surface. However, hard anodized AA and AA–AgNO3–QACs possessed over all of their surface a homogeneous layer of anodization with a thickness of about 50 μm. This layer was composed of organized arrays of pores with an overall diameter of 65–70 nm (the narrowing of the diameter of pores on anodized aluminum materials, due to the clogging stage, is not considered in these analyses) (Fig. 2). These results agree well with those of other studies showing the formation of an anodized layer thickness ranging from 25 to 100 μm with pore diameters between 58 and 134 nm depending on the operating parameters (acid solution, temperature, current density).53,61,62
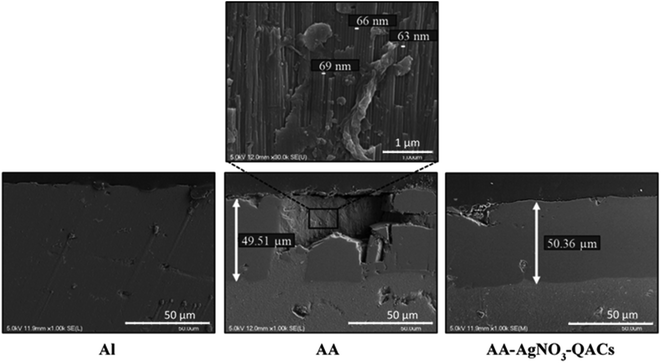 |
| Fig. 2 Characterization of the anodized layer of reference materials using cross sectional observation by scanning electron microscopy. Results are representative of two independent experiments and four replicates. | |
Hard anodization, consisting in electrolysis in a sulfuric acid solution (H2SO4: 1–15% w/v), is performed at a low temperature (between −5 and 5 °C) with a high current density (20 mA cm−2) and an anodization time varying between 60 to 90 min.63,64 Therefore, bacillus-shaped (≃1.0 μm wide by 3.0 μm long) and coccus-shaped (≃ 1.0 μm diameter) bacteria would be in contact with ≃780 and 200 pores, respectively, when deposited on these surfaces.
3.1.2. Release kinetics of antibacterial agents impregnated on anodized aluminum materials.
Quaternary ammonium compounds (QACs) release kinetics. The release kinetics of the QACs were carried out on AA, AA–AgNO3, AA–QACs and AA–AgNO3–QACs (Fig. 3). The quantification of QACs release was calculated from a standard curve (Fig. S2†). As expected, no release of QACs was detected from both AA (negative control) and AA–AgNO3. The release kinetics for AA–QACs and AA–AgNO3–QACs were very similar and showed an extremely fast QACs release, from the first minutes of immersion in water, followed by a slower release up to 72 h of immersion (around 20 and 40 mg L−1 of QACs released after 5 min and 1 h of immersion, respectively). These two distinct phases are characteristic of release patterns from nanoporous anodized aluminum structures in non-agitated systems.65,66
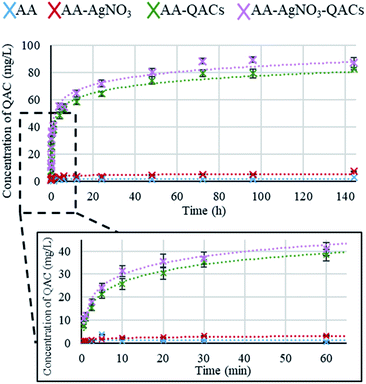 |
| Fig. 3 Amount of QACs released (mg L−1) from anodized aluminum materials after immersion in nanopure water for different periods of time. Results are means ± SD (n = 4; N = 12). | |
Therefore, the use of these materials in liquid environments (immersed) is not recommended.
Silver ion (Ag+) release kinetics. Tests using ICP-OES, allowing an ion detection level of 23 ppb, were carried out to assess the release of Ag+ from AA–AgNO3–QACs. No Ag+ could be detected following an immersion for up to 1 h and 24 h, which is consistent with the study carried out by Valiei et al.36 Hence, the impact of Ag+ on the antibacterial activity of the impregnated surfaces is expected to be minimal compared to that of QACs, as suggested also by our Minimal Inhibitory Concentration (MIC) and Minimal Bactericidal Concentration (MBC) assays.
3.2. Minimal Inhibitory Concentration (MIC) and Minimal Bactericidal Concentration (MBC) assay
Before assessing the antibacterial efficiency of impregnated materials, it was essential to first characterize the activity of antibacterial agents selected for impregnation of the surface materials. The MIC and MBC of (i) AgNO3 (1% w/v), (ii) QACs-based (10.9% w/v) solution and (iii) a combination of both AgNO3 (1% w/v) and QACs-based (10.9% w/v) solutions were determined on the pathogenic bacteria S. aureus, C. difficile, vancomycin-resistant E. faecium and E. faecalis, E. coli, streptomycin-resistant S. typhimurium and K. pneumoniae after 18–24 h incubation (Table 2).
Table 2 MIC and MBC of impregnation solutions (AgNO3, QACs and AgNO3 + QACs) on different Gram-positive and Gram-negative bacteria stainsa
|
AgNO3 (mg L−1 of AgNO3) |
QACs (mg L−1 of QACs) |
AgNO3 + QACs (mg L−1 of QACs) |
MIC |
MBC |
MIC |
MBC |
MIC |
MBC |
Results are means ± SEM (n = 4; N = 16). |
Gram-positive |
S. aureus |
35.40 ± 5.91 |
95.10 ± 15.80 |
0.22 ± 0.03 |
1.62 ± 0.29 |
0.27 ± 0.03 |
1.88 ± 0.23 |
C. difficile |
58.60 ± 5.03 |
87.83 ± 10.96 |
0.87 ± 0.09 |
1.19 ± 0.17 |
0.94 ± 0.10 |
1.11 ± 0.08 |
Vancomycin-resistant E. faecium |
28.09 ± 3.33 |
63.43 ± 13.95 |
0.98 ± 0.09 |
1.53 ± 0.19 |
0.98 ± 0.09 |
1.53 ± 0.19 |
Vancomycin-resistant E. faecalis |
90.22 ± 17.19 |
302.90 ± 55.42 |
1.02 ± 0.09 |
1.02 ± 0.09 |
1.06 ± 0.09 |
1.36 ± 0.15 |
Gram-negative |
E. coli |
5.65 ± 0.88 |
11.59 ± 1.66 |
2.56 ± 0.25 |
3.07 ± 0.38 |
3.92 ± 0.35 |
4.09 ± 0.35 |
Streptomycin-resistant S. typhimurium |
25.63 ± 2.35 |
42.73 ± 5.70 |
3.03 ± 0.40 |
4.94 ± 1.19 |
3.75 ± 0.34 |
7.84 ± 1.76 |
K. pneumoniae |
13.42 ± 1.22 |
25.63 ± 2.81 |
3.41 ± 0.30 |
3.41 ± 0.30 |
3.41 ± 0.30 |
3.41 ± 0.30 |
The mechanism of action of AgNO3 is induced by the release of Ag+ in solution. These ions bind to the thiol groups of proteins in cell membranes, enzymes and DNA, causing their denaturation and affecting their functions.67,68 For all the bacteria, MIC and MBC obtained with the AgNO3 solution varied between 5.65 and 90.22 mg L−1 and between 11.59 and 302.90 mg L−1, respectively (Table 2). These values are in accordance with the MIC range identified in the literature for S. aureus, E. coli and vancomycin-resistant enterococci.69–72 MIC and MBC values were also significantly higher for Gram-positive bacteria (MIC between 28.09 and 90.22 mg L−1 and MBC between 63.43 and 302.90 mg L−1) than for Gram-negative bacteria (MIC between 5.65 and 25.63 mg L−1 and MBC between 11.59 and 42.73 mg L−1) (p < 0.0001; Table 2). On the other hand, the MIC and MBC values for bacteria resistant to vancomycin (E. faecium and E. faecalis) or streptomycin (S. typhimurium), were similar to other bacteria of their Gram-positive and Gram-negative groups, respectively (Table 2).
QACs mainly damage the cytoplasmic membrane of bacteria by disrupting the lipid bilayers through the alkyl chains of QACs molecules.73 This mode of action is attributed to various factors such as molecular weight, molecular charge density or the length of the QACs N-alkyl chains.74–82 The evaluation of the QACs solutions showed MIC ranging from 0.22 to 3.41 mg L−1 and MBC ranging from 1.02 to 4.94 mg L−1, coinciding perfectly with values found in the literature for chlorinated QACs (0.25 to 5.0 mg L−1).83–86 A significant difference between Gram-positive and Gram-negative bacteria was observed for the QACs solution and was inversed with what was observed for the AgNO3 solution. Indeed, MIC and MBC values were significantly lower for Gram-positive bacteria (MIC between 0.22 and 1.02 mg L−1 and MBC between 1.02 and 1.62 mg L−1) than for Gram-negative bacteria (MIC between 2.56 and 3.41 mg L−1 and MBC between 3.07 to 4.94 mg L−1) (p < 0.0001; Table 2).
Although QACs are broad-spectrum antibacterial agents against Gram-positive and Gram-negative bacteria, the cell wall of Gram-positive cells is composed of a single plasma membrane, generally making them more sensitive to QACs than Gram-negative bacteria that possess two lipid bilayers.87,88
In addition, for all the tested bacteria, MIC and MBC obtained with the AgNO3 solution were significantly higher (100 to 1500 times) than those obtained with the QACs solutions (p < 0.0001), demonstrating the strong antibacterial efficiency of QACs on Gram-positive and Gram-negative bacteria. This likely explains why MIC and MBC values with the AgNO3 + QACs combination were quite similar to those obtained with the QACs alone (Table 2).
All the results described above were performed on vegetative bacteria, i.e., metabolically active bacteria. Since bacterial spores represent important vehicles for dissemination of certain bacterial pathogens, the sporicidal potential of the impregnating solutions was assessed on C. difficile spores (≃1.6 × 105 spores per mL), following contact times of 1, 4 and 24 h. The sporicidal disinfectant Virox™5 was used as a comparison control (Fig. S3†). The results showed that none of the QACs-containing solutions (QACs alone or AgNO3 + QACs) induced a significant decrease in the number of C. difficile spores, as opposed to the sporicidal controls (1/16 (v/v): Virox™5/water) for which no viable spores were detected (Fig. S3†). These results are in agreement with the literature showing that QACs are generally non-sporicidal.89
However, in this study, all the vegetative bacteria showed great sensitivity to the QACs-containing solutions, thus justifying the interest of their use for manufacturing antibacterial surfaces.
3.3. Assessing the antibacterial properties of materials on different pathogenic bacteria
The antibacterial activity of materials was evaluated against both Gram-positive (S. aureus, C. difficile, vancomycin-resistant E. faecium) and Gram-negative pathogens (streptomycin-resistant S. typhimurium and K. pneumoniae possessing a capsule) following contact times from 0.25 to 60 min. In addition, we compared three different inoculation methods (Fig. 1): (i) a swab liquid-inoculation assay (Fig. 4), (ii) a humid-transfer inoculation assay from a contaminated gel (Fig. 5) and (iii) a dry-transfer inoculation assay from a contaminated filter (Fig. 6). These different methodologies were chosen to allow comparison of the antibacterial activity of materials under different humidity conditions, to mimic wet (fomites, aerosols) and dry (contaminated hands of patients, medical equipment or other inert objects) environments associated with transmission of bacterial pathogens.90–92 Fig. 4–6 show the results with copper (antibacterial control), AA (negative control) and AA–QACs, while results obtained with Al, AA–AgNO3 (identical results to the AA control) and AA–AgNO3–QACs (identical results to AA–QACs) are presented in ESI (Fig. S4–S6†).
3.3.1. Antibacterial activity of materials using swab liquid-inoculation assay. The initial number of bacteria recovered from negative control AA was ≃1.6 × 106 CFU per surface, as determined after a 0 min contact time. This inoculum was sufficient to observe a 3-log decrease in bacterial viability (99.9% antibacterial activity).43 Bacterial counts were stable on both Al and AA negative control materials with ≃1.6 × 106 CFU per surface within 60 min for all tested bacteria (Fig. 4 and S4†), showing that the anodization process alone did not confer antibacterial property on Al. Compared to AA, copper showed a significant decrease in bacterial counts (99.9%, corresponding to a 3-log CFU per surface decrease) after 15 min with S. aureus and C. difficile (1.5 × 103 and 1.4 × 102 CFU per surface, respectively), and after 60 min with S. typhimurium (3.1 × 102 CFU per surface) (Fig. 4). These values are in agreement with the well-known antibacterial properties of copper (C70600 alloy), inducing 99.9% antibacterial activity after 15 and 30 min of contact with E. coli and S. aureus, respectively.93,94 Another study showed that against C. difficile, copper showed the same antibacterial activity (99.9%) after 240 min.95
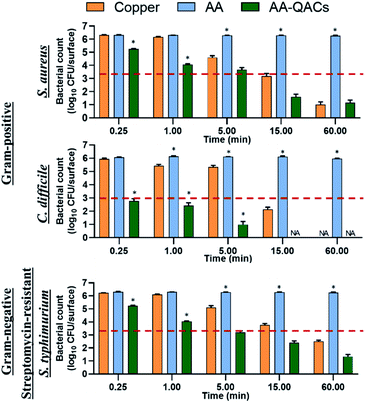 |
| Fig. 4 Swab liquid-inoculation assay evaluating the antibacterial activity of different materials. Results are means ± SEM (n = 2; N = 8). The reference of 99.9% antibacterial activity is indicated by the dotted red line. Statistical effect compared to copper: *p < 0.001. NA: no bacteria counted. | |
AA–AgNO3 had no significant effect on bacterial viability for all contact times, demonstrating that impregnation with an AgNO3 (1% w/v) solution did not induce antibacterial activity on AA within 60 min (Fig. S4†). This was expected based on our MIC and MBC assays (Table 2), as well as the release kinetics assays (Fig. 3). In contrast, the AA–QACs caused a greater bacterial decrease than AA and copper after 0.25 and 1 min of contact, for all the bacteria (p < 0.001: Fig. 4). For example, AA–QACs showed 99.9% antibacterial activity after 0.25 min for C. difficile (p < 0.001: 6.0 × 103 CFU per surface), 5 min for S. typhimurium (p < 0.001: 1.5 × 103 CFU per surface) and 15 min of contact with S. aureus (p < 0.001: 4.1 × 101 CFU per surface). The antibacterial effect of AA–QACs was even more pronounced after longer contact times, decreasing the quantity of surviving bacteria to <2.0 × 101 CFU per surface after 5 min for C. difficile (1.04 × 101 CFU per surface) and 60 min for S. aureus and S. typhimurium (1.4 × 101 and 1.9 × 101 CFU per surface, respectively) (Fig. 4). Finally, similar results to AA–QACs were obtained with AA–AgNO3–QACs (Fig. S4†).
Although we did not observe sporicidal activity in MIC and MBC assays with the various impregnation solutions (Fig. S3†), we also tested the sporicidal activity of the different materials on C. difficile spores after 1 and 24 h of contact. As expected, no sporicidal effect could be observed when compared to the AA negative control (Fig. S4†).
In summary, under liquid conditions using the swab test, anodized aluminum materials impregnated with QACs showed a faster and stronger antibacterial activity against both Gram-positive and Gram-negative bacteria compared to copper, whose alloy is approved as an antibacterial reference by EPA.39 In addition, the impregnation with AgNO3, alone or in combination with QACs, did not improve the overall activity of materials (Fig. S4†). Therefore, consistent with the MIC and MBC results, the effect of AgNO3 compared to QACs appeared to be negligible in this study.
3.3.2. Antibacterial activity of materials using a humid-transfer inoculation assay. We next wanted to assess the antibacterial properties of the different materials using a humid-transfer protocol, to mimic a wet contamination (fecal spills, contaminated aerosols, or food). To do this, we developed a gel contamination assay, which recreates conditions of high humidity, but without excessive liquid like with the swab method. Contact kinetics of 0.25, 1, 5, 15 and 60 min were done with S. aureus, C. difficile, vancomycin-resistant E. faecium, streptomycin-resistant S. typhimurium and K. pneumoniae (Fig. 5). The number of bacteria at time zero on contaminated gels was ≃6.0 × 105 CFU cm−2, allowing a sufficient bacterial inoculum deposit on the materials to assess their antibacterial properties (6.0 × 104 and 4.0 × 105 CFU per surface depending on the type of bacteria).43 The number of bacteria was stable over time on both AA and Al negative controls and their contaminated gels (Fig. 5 and S5†).
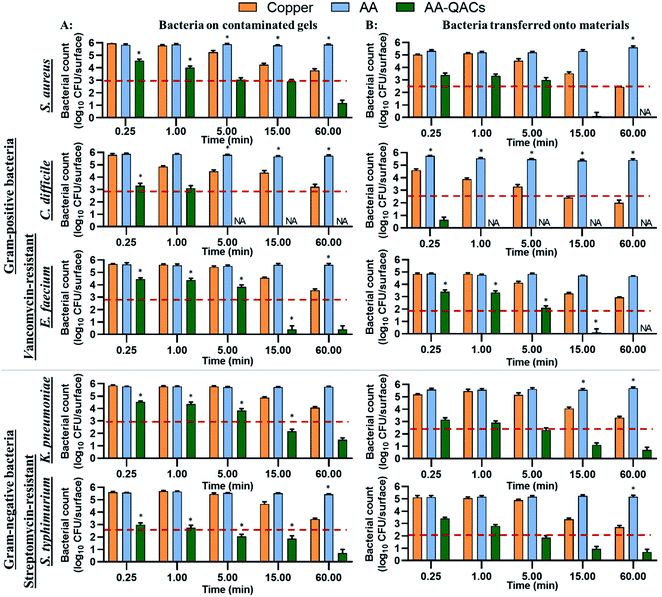 |
| Fig. 5 Humid-transfer inoculation assay from contaminated gel evaluating the antibacterial activity of materials. The graph shows total counts of bacteria that survived (A) on the contaminated gel or (B) after transfer from the gel to the different materials. The reference of 99.9% antibacterial activity is indicated by the dotted red line. Results are means ± SEM (n = 2; N = 8). Statistical significance when compared to copper: *p < 0.001. NA: no bacteria counted. | |
The copper induced a significant decrease of 99% in bacterial counts (2-log CFU cm−2 decrease) on contaminated gels compared to AA after 60 min of contact with S. aureus, C. difficile, E. faecium and S. typhimurium (p < 0.001, Fig. 5A). In contrast, copper had little antibacterial activity on gels contaminated with K. pneumoniae (not significant). The low efficiency of copper on K. pneumoniae may be explained by the fact that this bacterium produces a thick polysaccharide capsule which provides protection against antibacterial agents, in addition to contributing to its virulence.96–98 However, when assessing bacterial survival after transfer from the gel to the surface of the different materials, copper induced a significant decrease of 99.9% in S. aureus and C. difficile counts (p < 0.001) and of 99% in counts of K. pneumoniae, S. typhimurium (p < 0.001) and E. faecium (p < 0.01) after a contact time of 60 min (Fig. 5B). The release of copper ions is considered to be the key mechanism of antibacterial activity of these materials.99,100 In the humid-transfer inoculation assay, the reduced water content limits the release of copper ion, which might explain why copper presented a lower activity with the gel inoculation protocol than with the swab or international standards methodology.39,43
Furthermore, while AA and AA–AgNO3 gave results similar to Al (Fig. S5†), AA–QACs had a greater antibacterial activity on contaminated gels after 0.25 min of contact for all bacteria, compared to the copper control (p < 0.001: Fig. 5A). For example, the AA–QACs showed 99.9% antibacterial activity on contaminated gels after 5 min for C. difficile and S. typhimurium (p < 0.001: 0 and 1.1 × 102 CFU per surface, respectively), and 15 min for S. aureus, E. faecium and K. pneumoniae (p < 0.001: 8.2 × 102, 2.5 × 100 and 1.5 × 102 CFU per surface, respectively). The antibacterial activity of AA–QACs was greater and faster following transfer of bacteria onto materials (<1.0 × 102 CFU per surface; Fig. 5B), resulting in 99.995% of bacteria killed after 0.25 min of contact with C. difficile (p < 0.001: 4.2 × 101 CFU per surface) and after 15 min of contact with S. aureus, E. faecium, K. pneumoniae and S. typhimurium (p < 0.001: 1.2 × 100, 1.3 × 100, 1.3 × 101 and 8.8 × 101 CFU per surface, respectively).
Finally, AA–AgNO3–QACs showed a great efficiency, equivalent to AA–QACs, confirming that the addition of AgNO3 did not improve the overall activity of the materials (Fig. S5†).
3.3.3. Antibacterial activity of materials using a dry-transfer inoculation assay. The dry contamination of high-touch surfaces, for example through contaminated hands, tissues, medical devices or other objects, actively participates in the transmission of pathogenic bacteria in both hospital and community environments.90–92 Therefore, tests mimicking bacterial dry-transfer were carried out to assess the killing efficiency of antibacterial materials in a similar context. To this end, we developed a filter contamination assay that recreates low humidity conditions (<20% RH). Contact times of 0.25, 1 and 5 min were used in assays with S. aureus, C. difficile, vancomycin-resistant E. faecium, streptomycin-resistant S. typhimurium and K. pneumoniae (Fig. 6). The number of bacteria at time zero on the contaminated filters was ≃1.0 × 106 CFU cm−2. The initial number of bacteria deposited on each material varied between 6.9 × 104 and 7.8 × 105 CFU per surface depending on the bacterial species tested. The initial quantity of inoculum on all contaminated filters, was stable over time (0.25, 1 and 5 min) for all bacteria (Fig. 6A). The different materials tested had no impact on the viability of bacteria present on the filters and the number of bacteria transferred to both AA and Al negative controls remained stable over time for all bacteria species (Fig. 6B). The copper showed only an antibacterial activity of 99% (2-log CFU per surface decrease) after 1 and 5 min of contact with C. difficile (p < 0.001: 7.4 × 102 CFU per surface) and killed less than 90% (≤1-log CFU per surface decrease) of S. aureus, E. faecium, K. pneumoniae and S. typhimurium (Fig. 6). These results differ from those obtained with the gel inoculation protocol, where copper had antibacterial activity greater than 99.9% (≥3 log of CFU per surface decrease) after 60 min of contact (Fig. 5B). This difference can be explained by the fact that contact times ≤ 5 min in dry conditions would not allow the sufficient release of copper ions to induce bacterial death.99,100 This demonstrates the relevance of assessing different methodologies and contamination protocols mimicking real conditions to which materials will be exposed to (short contact time, low humidity, etc.).101,102 Moreover, the number of bacteria transferred to AA–AgNO3 was not affected over time for all bacteria (Fig. S6B†). In contrast, AA–QACs induced a significant decrease of more than 99.5% bacteria after 1 min of contact with S. aureus, C. difficile, E. faecium, K. pneumoniae and S. typhimurium (p < 0.001: 1.2 × 103, 2.9 × 102, 2.6 × 102, 1.3 × 103 and 2.5 × 103 CFU per surface, respectively) (Fig. 6). Similar results were obtained with AA–AgNO3–QACs (Fig. S6B†).
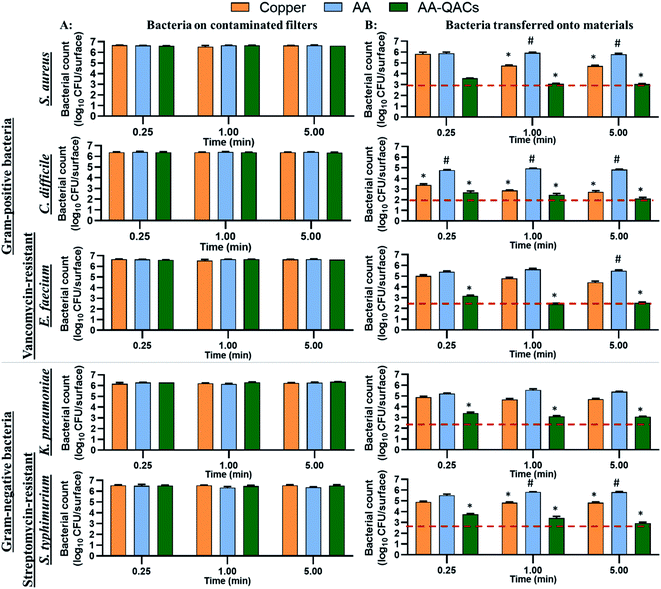 |
| Fig. 6 Dry-transfer inoculation assay evaluating the antibacterial activity of materials after contact with a contaminated filter under low humidity conditions. Bacterial counts in log10 CFU per surface are indicated for (A) contaminated filters and (B) after transfer onto materials. Results are means ± SEM (n = 2; N = 8). The reference of 99.9% antibacterial activity is indicated by the dotted red line. Statistical effect compared to AA: *p < 0.001 and to copper: #p < 0.001. | |
To summarize, by mimicking liquid and humid transmission routes (swab and humid-transfer assay), responsible for frequent hospital and community contaminations (fecal spills, aerosols, contaminated food), AA containing QACs showed a high and quick antibacterial efficiency (more than 99.95% in less than 15 min) on all pathogenic vegetative bacteria tested, thus exceeding the antibacterial activity required by standardization agencies43,103 (Fig. 4 and 5). In addition, by mimicking contamination under dry conditions, these materials showed promising results even for short contact times (e.g., 1 min) and were more efficient than the copper reference (Fig. 6). This high antibacterial performance in short contact times is a highly desired feature in the manufacturing of high-touch antibacterial surfaces since it is crucial to reduce pathogenic transmission linked to the contaminated inert environment. In fact, in public areas with high contact surfaces, such as in hospitals, the average elapsed time between two people touching the same surface is estimated at 5 min.101,102 Following humid contact, the AA–QACs allowed the contaminated gels to be “partially sterilized” (99.9% decrease in bacteria in less than 5 or 15 min of contact) (Fig. 5). However, AA–QACs had no impact on the viability of bacteria on the contaminated filters. Thus, the mode of action of AA–QACs appears to require the presence of humidity to induce sufficient release of the active agents from the material, consistent with the rapid release kinetic of QACs observed following material immersion (Fig. 3).
In our assays, the filter was the source of the contamination and it's relative humidity was ≃20%, which is similar to the superficial layers of Stratum Corneum in the human epithelium.45,104 Under these conditions, the release and transfer of QACs to the filter was negligeable. However, it was sufficient to allow effective and rapid antibacterial activity against bacteria transferred onto the materials. These results suggest that under RH conditions similar to those of the skin, AA–QACs may not have harmful impacts on the human skin or its microbiome (good bacteria colonizing the human skin playing an essential protective role105), while enable self-disinfection of the contaminated surfaces. This would constitute a very important advantage since the disruption (dysbiosis) of this microbial balance can affect immune defenses, cause excessive inflammation of the skin tissue and lead to the development of pathologies such as acne or psoriasis.106,107 However, the impact of AA–QACs on the microbiome or human skin tissue must be determined in a future study.
3.4. Effectiveness of antibacterial materials after washing with cleaning products
The inert surfaces in public environments (door knobs, stair railings, support ramps, etc.) are regularly cleaned using specific sanitizing and disinfecting agents.108 To assess longevity of the AA–QACs materials, the effects of several cleaning products on the antibacterial properties of the materials were evaluated using S. aureus and a constant contact time of 1 h (Fig. 7). The tests assessed the impact of 0, 5 and 10 consecutive washes with different cleaning solutions containing: (a) sterile water, (b) ethanol, (c) Virox™5, and (d) a QACs-based commercial solution. No significant decrease in the number of surviving bacteria was observed on the Al and AA controls following 5 or 10 washes with sterile water or ethanol. However, a slight decrease in CFU per surface (1 log reduction), was observed on the Al and AA following 10 washes with Virox™5 (5.8 × 105 and 4.3 × 105 CFU per surface, respectively; p < 0.0001) and 5 or 10 washes with the QACs solution (1.7 × 105 and 1.4 × 105 CFU per surface for Al and 2.7 × 104 and 8.7 × 103 CFU per surface for AA, respectively; p < 0.0001) (Fig. 7). Antibacterial residues from the series of washes could explain this slight drop in bacterial counts on these negative control materials. Indeed, after their use, the elimination of QACs (cationic surfactant) requires the use of abundant rinsing.109 In addition, QACs such as ADBAC and DDAC have extremely low vapor pressures (3.53 × 10−12 mm Hg (4.7 × 10−10 Pa) and 2.33 × 10−11 mm Hg (3.1 × 10−9 Pa), respectively).110,111 Therefore, the potential residues of these QACs do not volatilize spontaneously and persist on materials.
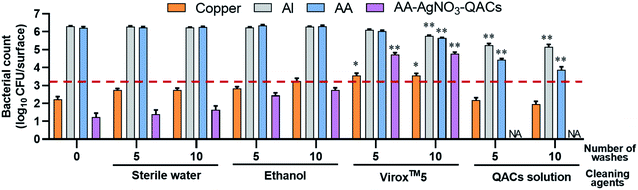 |
| Fig. 7 Impact of 0, 5 or 10 consecutive washes with different cleaning solutions on the antibacterial properties of materials tested against S. aureus. The reference of 99.9% antibacterial activity is indicated by the dotted red line. Results are means ± SEM (n = 3; N = 12). Statistical effect compared to the corresponding material without cleaning agent: *p < 0.01 and **p < 0.0001. NA: no bacteria counted. | |
The antibacterial activity of copper remained relatively unaffected after washes with sterile water, ethanol or QACs solution. Similarly, the antibacterial activity of AA–AgNO3–QACs was relatively unaffected by 5 or 10 washes with sterile water or ethanol. However, Virox™5 washes resulted in a significant decrease in the antibacterial activity of copper (3.6 × 103 CFU per surface, p < 0.01) and AA–AgNO3–QACs (5.4 × 104 CFU per surface, p < 0.0001) (Fig. 7). Hydrogen peroxide (H2O2) is a strong oxidizing agent (Eo = 1.763 V at pH 0, Eo = 0.878 V at pH 14).112 Thus, it alters the copper by electrochemical dissolution, resulting in a maximum elimination rate of copper with 1% H2O2 (Virox™5 use concentration ≃0.5% H2O2).113 Moreover, clogging of anodized aluminum materials gives them stability and protection against corrosion and abrasion.55,114,115 However, in this study, the AA surface is covered by a nanoporous layer. Hence, H2O2 can penetrate into the anodized layer, affecting its integrity.
Of note, the washes carried out with the QACs solution improved the antibacterial properties of AA–AgNO3–QACs, with no viable counts detectable after 5 washes (Fig. 7). As in previous experiments, results similar to those obtained with AA–AgNO3–QACs are expected from AA–QACs materials. In summary, the integrity of the AA impregnated with QACs, and their antibacterial properties must be maintained by using appropriate cleaning solutions (e.g., QACs solution), if repeated washing procedures are to be applied. The added advantage of such cleaning step is that it seems to improve the overall antibacterial efficiency of the materials.
3.5. Effectiveness of antibacterial materials following immersions in water
As demonstrated by our release kinetics assays, immersion of AA–QACs (or AA–AgNO3–QACs) in water leads to a rapid release of QACs from the impregnated surfaces. To assess the impact of water immersion on the antibacterial activity of AA–AgNO3–QACs, and to determine the suitable applications for these materials, we tested the impact of a series of successive 12 h immersions in water. The swab liquid-inoculation assay was performed on S. aureus with a constant contact time of 1 h (Fig. 8). Without immersion, the AA–AgNO3–QACs showed a significant 99.9999% antibacterial activity compared to AA (p < 0.001). No surviving bacteria could be detected on these materials after 1 h of contact. Similar results were observed after 1, 2, and 3 rounds of immersions (≃0 CFU per surface, p < 0.001). Following 4 and 5 rounds of immersion, the antibacterial activity of the AA–AgNO3–QACs decreased slightly (1.0 × 102 CFU per surface after 5 rounds), while still maintaining a great efficiency by eliminating 99.99% of bacteria. However, after 6, 7, and 8 rounds of immersion, the AA–AgNO3–QACs surfaces gradually lost their antibacterial activity (2.4 × 103, 2.7 × 104, 6.7 × 104 CFU per surface, respectively) (Fig. 8). After 9 and 10 immersions, the materials completely lost their antibacterial properties and were comparable to the AA control (6.2 × 105 and 9.0 × 105 CFU per surface; Fig. 8). This phenomenon is likely explained by the fact that following immersion in water, the antibacterial QACs compounds were quickly released from the materials (AA–QACs or AA–AgNO3–QACs), as demonstrated in Fig. 2, and several consecutive immersions led to complete emptying of the nanopores. These results demonstrate that AA materials impregnated with QACs would not be recommended for applications where surfaces are frequently or constantly immersed in a liquid environment (e.g., baths, toilets, taps, etc.).
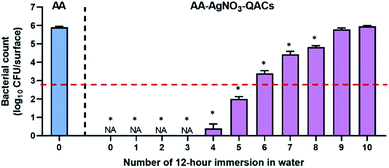 |
| Fig. 8 Impact of successive 12 h immersions in sterile water on the antibacterial properties of materials on S. aureus. The reference of 99.9% antibacterial activity is indicated by the dotted red line. Results are means ± SEM (n = 3; N = 12). Statistical effect compared to AA: *p < 0.0001. NA: no bacteria counted. | |
Altogether, our results demonstrate the need to define the appropriate applications and the most suitable and least damaging sanitation protocols for antibacterial materials in order to assess the durability of their antibacterial properties.116,117 Along the same line, most international standards are in the process of being updated since they do not currently assess the time and cleaning products' impacts on the antibacterial properties of materials, and they do not consider the conditions of use.
4. Conclusions
One of the biggest challenges in the design of antibacterial high-touch surfaces is the sustainable conservation of their properties. The antibacterial properties of materials (inherent material activity or surface modifications) fluctuate depending on the type of bacteria with which they interact, the typical surface wear (e.g., fouling), the conditions of their use (humidity, temperature, dry or liquid environment, etc.) and the cleaning and disinfection procedures. These parameters should always be properly characterized and considered when developing and evaluating their antibacterial properties. In this study, nanoporous AA–QACs surfaces demonstrated excellent and rapid antibacterial efficiency on a wide range of Gram-positive and Gram-negative bacteria, independent of the presence of a capsule or antibiotic resistance. Although AA–QACs surfaces were not sporicidal, they nonetheless outperformed copper alloys, considered to be reference antibacterial materials. Furthermore, this high performance in short contact times has been demonstrated under conditions mimicking various surface contamination scenarios: liquid (swab), humid (contaminated gel) and dry (contaminated filter). Importantly, these materials must be used in dry environments (not submerged in liquids) and should be cleaned with QACs-based solutions to preserve their antibacterial properties. Hard-anodized aluminum materials impregnated with QACs constitute a promising innovative strategy to prevent the transmission of pathogenic bacteria linked to the contaminated inert environment.
Author contributions
JJ and OD performed the experiments. JJ, OD, NF and LCF analyzed the data. JJ, OD GS, XGC, NF and LCF designed the study and experiments. MAG provided the materials. JJ, NF and LCF wrote the manuscript and all authors revised and approved the final version.
Conflicts of interest
The authors declare that they have no known competing financial interests or personal relationships that could have appeared to influence the work reported in this paper.
Acknowledgements
This research was supported by a Rio Tinto graduate scholarship and by a Fonds de recherche du Québec – Nature et technologies (FRQNT) doctoral research scholarship (#304067) to JJ, as well as a team grant from FRQNT Programme de recherche en partenariat sur la production et la transformation de l'aluminium (#2018-LU-210883). The authors would like to thank Maxime Dumont (A3 Surfaces) who performed the anodization and impregnation process, and Jean-Luc Bernier for insightful discussions during the project.
References
- World Health Organization, Report on the Burden of Endemic Health Care-Associated Infection Worldwide, WHO Libr. Cat. Data, 2012, pp. 1–25 Search PubMed.
- World Health Organization, Plan d’action mondial pour combattre la résistance aux antimicrobiens, WHO Libr. Cat. Data, 2016, pp. 1–32 Search PubMed.
- Interagency Coordination Group on Antimicrobial Resistance, No time to Wait: Securing the Future from Drug-Resistant Infections, Rep. to Secr. Gen. United Nations, 2019, vol. 1, pp. 1–28 Search PubMed.
- U.S. Department of Health and Human Services, Antibiotic Resistance Threats in the United States, CDC Centers Dis. Control Precention, 2019, vol. 1, pp. 1–150 Search PubMed.
- World Bank Group, Drug-Resistant Infections: A Threat to Our Economic Future, 2017 Search PubMed.
- G. Suleyman, G. Alangaden and A. C. Bardossy, The Role of Environmental Contamination in the Transmission of Nosocomial Pathogens and Healthcare-Associated Infections, Curr. Infect. Dis. Rep., 2018, 20, 1–11 CrossRef PubMed.
- J. A. Otter and G. L. French, The Role Played by Contaminated Surfaces in the Transmission of Nosocomial Pathogens, Infect. Control Hosp. Epidemiol., 2011, 32, 687–699 CrossRef PubMed.
- T. Z. Wang, M. S. Simon, L. F. Westblade, L. Saiman, E. Y. Furuya and D. P. Calfee, Quantitative characterization of high-touch surfaces in emergency departments and hemodialysis facilities, Infect. Control Hosp. Epidemiol., 2021, 42, 474–476 CrossRef PubMed.
- M. M. Querido, L. Aguiar, P. Neves, C. C. Pereira and J. P. Teixeira, Self-disinfecting surfaces and infection control, Colloids Surf., B, 2019, 178, 8–21 CrossRef CAS PubMed.
- B. C. Sousa, C. J. Massar, M. A. Gleason and D. L. Cote, On the emergence of antibacterial and antiviral copper cold spray coatings, J. Biol. Eng., 2021, 15, 1–15 CrossRef PubMed.
- V. M. Villapún, L. G. Dover, A. Cross, S. González and C. R. Arciola, Antibacterial Metallic Touch Surfaces, Materials, 2016, 9, 736 CrossRef PubMed.
- D. J. Weber and W. A. Rutala, American Journal of Infection Control Self-disinfecting surfaces: Review of current methodologies and future prospects, Am. J. Infect. Control, 2013, 41, 31–35 CrossRef PubMed.
- C. Espírito Santo, E. Wen Lam, C. G. Elowsky, D. Quaranta, D. W. Domaille, C. J. Chang and G. Grass, Bacterial Killing by Dry Metallic Copper Surfaces, Appl. Environ. Microbiol., 2011, 77, 794–802 CrossRef PubMed.
- J. J. Green, K. Drye, W. Llp and K. Street, Antimicrobial Copper Alloys – Group I (EPA Reg. No. 82012-1) and Associated Fabricated Products, Washington, D.C, 2014 Search PubMed.
- M. Colin, F. Klingelschmitt, E. Charpentier, J. Josse, L. Kanagaratnam, C. De Champs and S. C. Gangloff, Copper alloy touch surfaces in healthcare facilities: An effective solution to prevent bacterial spreading, Materials, 2018, 11, 1–12 CrossRef PubMed.
- D. Mitra, E. T. Kang and K. G. Neoh, Antimicrobial Copper-Based Materials and Coatings: Potential Multifaceted Biomedical Applications, ACS Appl. Mater. Interfaces, 2020, 12, 21159–21182 CrossRef CAS PubMed.
- F. Pietsch, A. J. O'Neill, A. Ivask, H. Jenssen, J. Inkinen, A. Kahru, M. Ahonen and F. Schreiber, Selection of resistance by antimicrobial coatings in the healthcare setting, J. Hosp. Infect., 2020, 106, 115–125 CrossRef CAS PubMed.
- S. J. Dancer, How Much Impact Do Antimicrobial Surfaces Really Have on Healthcare-acquired Infection?, Clin. Infect. Dis., 2020, 71, 1814–1816 CrossRef PubMed.
- J. O'Gorman and H. Humphreys, Application of copper to prevent and control infection. Where are we now?, J. Hosp. Infect., 2012, 81, 217–223 CrossRef PubMed.
- M. P. Muller, C. MacDougall, M. Lim, I. Armstrong, A. Bialachowski, S. Callery, W. Ciccotelli, M. Cividino, J. Dennis, S. Hota, G. Garber, J. Johnstone, K. Katz, A. McGeer, V. Nankoosingh, C. Richard and M. Vearncombe, Antimicrobial surfaces to prevent healthcare-associated infections: A systematic review, J. Hosp. Infect., 2016, 92, 7–13 CrossRef CAS PubMed.
- I. Pineda, R. Hubbard and F. Rodriguez, The role of copper surfaces in reducing the incidence of healthcare-associated infections: A systematic review and meta-analysis, Can. J. Infect. Control, 2017, 32, 13–24 Search PubMed.
- E. M. Hetrick and M. H. Schoenfisch, Reducing implant-related infections: active release strategies, Chem. Soc. Rev., 2006, 35, 780 RSC.
- M. Zilberman and J. J. Elsner, Antibiotic-eluting medical devices for various applications, J. Controlled Release, 2008, 130, 202–215 CrossRef CAS PubMed.
- A. Tripathy, P. Sen, B. Su and W. H. Briscoe, Natural and bioinspired nanostructured bactericidal surfaces, Adv. Colloid Interface Sci., 2017, 248, 85–104 CrossRef CAS PubMed.
- E. P. Ivanova, J. Hasan, H. K. Webb, V. K. Truong, G. S. Watson, J. A. Watson, V. A. Baulin, S. Pogodin, J. Y. Wang, M. J. Tobin, C. Löbbe and R. J. Crawford, Natural bactericidal surfaces: Mechanical rupture of pseudomonas aeruginosa cells by cicada wings, Small, 2012, 8, 2489–2494 CrossRef CAS PubMed.
- X. He, X. Suo, X. Bai, C. Yuan and H. Li, Functionalizing aluminum substrata by quaternary ammonium for antifouling performances, Appl. Surf. Sci., 2018, 440, 300–307 CrossRef CAS.
- H. Agbe, D. K. Sarkar, X. G. Chen, N. Faucheux, G. Soucy and J. L. Bernier, Silver-Polymethylhydrosiloxane Nanocomposite Coating on Anodized Aluminum with Superhydrophobic and Antibacterial Properties, ACS Appl. Bio Mater., 2020, 3, 4062–4073 CrossRef CAS.
- H. Agbe, D. K. Sarkar and X. G. Chen, Tunable superhydrophobic aluminum surfaces with anti-biofouling and antibacterial properties, Coatings, 2020, 10, 1–10 CrossRef.
- C. P. Gerba, Quaternary Ammonium Biocides: Efficacy in Application, Appl. Environ. Microbiol., 2015, 81, 464–469 CrossRef PubMed.
- G. Mcdonnell and A. D. Russell, Antiseptics and Disinfectants: Activity, Action, and Resistance, Clin. Microbiol. Rev., 1999, 12, 147–179 CrossRef CAS PubMed.
- D. Druvari, N. D. Koromilas, G. Ch Lainioti, G. Bokias, G. Vasilopoulos, A. Vantarakis, I. Baras, N. Dourala and J. K. Kallitsis, Polymeric Quaternary Ammonium-Containing Coatings with Potential Dual Contact-Based and Release-Based Antimicrobial Activity, ACS Appl. Mater. Interfaces, 2016, 8, 35593–35605 CrossRef CAS PubMed.
- M. C. Jennings, K. P. C. Minbiole and W. M. Wuest, Quaternary Ammonium Compounds: An Antimicrobial Mainstay and Platform for Innovation to Address Bacterial Resistance, ACS Infect. Dis., 2015, 1, 288–303 CrossRef CAS PubMed.
- S. Bai, X. Li, Y. Zhao, L. Ren and X. Yuan, Antifogging/Antibacterial Coatings Constructed by N-Hydroxyethylacrylamide and Quaternary Ammonium-Containing Copolymers, ACS Appl. Mater. Interfaces, 2020, 12, 12305–12316 CrossRef CAS PubMed.
- W. A. Rutala and D. J. Weber, New disinfection and sterilization methods, Emerging Infect. Dis., 2001, 7, 348–353 CrossRef CAS PubMed.
- N. Silvestry-Rodriguez, E. E. Sicairos-Ruelas, C. P. Gerba and K. R. Bright, Silver as a disinfectant, Rev. Environ. Contam. Toxicol., 2007, 191, 23–45 CrossRef CAS PubMed.
- A. Valiei, M. Okshevsky, N. Lin and N. Tufenkji, Anodized Aluminum with Nanoholes Impregnated with Quaternary Ammonium Compounds Can Kill Pathogenic Bacteria within Seconds of Contact, ACS Appl. Mater. Interfaces, 2018, 10, 41207–41214 CrossRef CAS PubMed.
- S. Liu, J. Tian and W. Zhang, Fabrication and application of nanoporous anodic aluminum oxide: A review, Nanotechnology, 2021, 32, 1–20 Search PubMed.
- M. Dumont, J. Lambert, G. Leblanc, M. Lambert, M. Lavoie, J. P. Collard, M. Auclair-Gilbert, S. Gagnon, Anodized biocidal metallic material, process for making the material and method for reactivating the material, WO2021/113972A1, 2021.
- Copper Development Association Inc., Standard Designation for Wrought Copper Alloy, https://www.copper.org/resources/properties/db/datasheets/all-alloys.pdf, accessed 11 January 2021 Search PubMed.
- J. E. Galan and R. O. Y. Curtiss, Distribution of the invA, -B, -C, and -D Genes of Salmonella typhimurium among Other Salmonella Serovars: invA Mutants of Salmonella typhi Are Deficient for Entry into Mammalian Cells, Infect. Immun., 1991, 59, 2901–2908 CrossRef CAS PubMed.
- A. F. Gaudy, F. Abu-Niaaj and E. T. Gaudy, Statistical study of the spot-plate technique for viable-cell counts, Appl. Microbiol., 1963, 11, 305–309 CrossRef PubMed.
- J. Wang, M. Woo and C. Yan, Spot Plating Assay for the Determination of Survival and Plating Efficiency of Escherichia coli in sub-MIC Levels of Antibiotics, JEMI Methods, 2017, 1, 26–29 Search PubMed.
- International Organization for Standardization, ISO 22196: Measurement of antibacterial activity on plastics and other non-porous surfaces, Int. Stand., 2011, 1–15 Search PubMed.
- Food and Drug Administration, Pharmaceutical Microbiology Manual, Off. Regul. Sci., 2020, vol. 2, pp. 1–96 Search PubMed.
- P. J. Caspers, G. W. Lucassen, E. A. Carter, H. A. Bruining and G. J. Puppels, In vivo confocal Raman microspectroscopy of the skin: Noninvasive determination of molecular concentration profiles, J. Invest. Dermatol., 2001, 116, 434–442 CrossRef CAS PubMed.
- F. Hizal, N. Rungraeng, J. Lee, S. Jun, H. J. Busscher, H. C. Van Der Mei and C. Choi, Nanoengineered Superhydrophobic Surfaces of Aluminum with Extremely Low Bacterial Adhesivity, ACS Appl. Mater. Interfaces, 2017, 9, 12118–12129 CrossRef CAS PubMed.
- D. Wojcieszak, M. Mazur, D. Kaczmarek, P. Mazur, B. Szponar, J. Domaradzki and L. Kepinski, Influence of the surface properties on bactericidal and fungicidal activity of magnetron sputtered Ti–Ag and Nb–Ag thin films, Mater. Sci. Eng., C, 2016, 62, 86–95 CrossRef CAS PubMed.
- J. G. Buijnsters, R. Zhong, N. Tsyntsaru and J. Celis, Surface Wettability of Macroporous Anodized Aluminum Oxide, ACS Appl. Mater. Interfaces, 2013, 5, 3224–3233 CrossRef CAS PubMed.
- Y. Li, S. Yang, Y. Chen and D. Zhang, Hydrophobic and anti-fouling performance of surface on parabolic morphology, Int. J. Environ. Res. Public Health, 2020, 17, 644–655 CrossRef CAS PubMed.
- C. Ran, G. Ding, W. Liu, Y. Deng and W. Hou, Wetting on Nanoporous Alumina Surface: Transition between Wenzel and Cassie States Controlled by Surface Structure, Langmiur, 2008, 24, 9952–9955 CrossRef CAS PubMed.
- S. P. Rodrigues, C. F. A. Alves, A. Cavaleiro and S. Carvalho, Applied Surface Science Water and oil wettability of anodized 6016 aluminum alloy surface, Appl. Surf. Sci., 2015, 422, 430–442 CrossRef.
- C. Jeong and H. Ji, Single-Step Direct Fabrication of Pillar-on-Pore Hybrid Nanostructures in Anodizing Aluminum for Superior Superhydrophobic Efficiency, ACS Appl. Mater. Interfaces, 2012, 4, 842–848 CrossRef CAS PubMed.
- J. Zang, S. Yu, G. Zhu and X. Zhou, Fabrication of superhydrophobic surface on aluminum alloy 6061 by a facile and effective anodic oxidation method, Surf. Coat. Technol., 2019, 370, 125078 CrossRef.
- C. Jeong and H. Ji, Systematic control of anodic aluminum oxide nanostructures for enhancing the superhydrophobicity of 5052 aluminum alloy, Materials, 2019, 12, 3231–3242 CrossRef CAS PubMed.
- S. U. Ofoegbu and F. A. O. Fernandes, The Sealing Step in Aluminum Anodizing: A Focus on Sustainable Strategies for Enhancing Both Energy Efficiency and Corrosion Resistance, Coatings, 2020, 10, 1–55 CrossRef.
- C. P. Gerba, Quaternary Ammonium Biocides: Efficacy in Application, Appl. Environ. Microbiol., 2015, 81, 464–469 CrossRef PubMed.
- F. Bureš, Quaternary Ammonium Compounds: Simple in Structure,
Complex in Application, Top. Curr. Chem., 2019, 377, 1–21 CrossRef PubMed.
- J. Song, H. Kong and J. Jang, Bacterial adhesion inhibition of the quaternary ammonium functionalized silica nanoparticles, Colloids Surf., B, 2011, 82, 651–656 CrossRef CAS PubMed.
- J. Pant, J. Gao, M. J. Goudie, S. Hopkins, J. Locklin and H. Handaa, A Multi-defense Strategy: Enhancing Bactericidal Activity of a Medical Grade Polymer with a Nitric Oxide Donor and Surface immobilized Quaternary Ammonium Compound, Acta Biomater., 2017, 58, 421–431 CrossRef CAS PubMed.
- D. Wojcieszak, D. Kaczmarek, A. Antosiak, M. Mazur, Z. Rybak, A. Rusak, M. Osekowska, A. Poniedzialek, A. Gamian and B. Szponar, Influence of Cu–Ti thin film surface properties on antimicrobial activity and viability of living cells, Mater. Sci. Eng., C, 2015, 56, 48–56 CrossRef CAS PubMed.
- F. Keller, M. S. Hunter and D. L. Robinson, Structural Features of oxide coating on Aluminium, J. Electrochem. Soc., 1953, 100, 411–419 CrossRef CAS.
- P. G. Sheasby and R. Pinner, The surface Treatment and Finishing of Aluminium and its Alloys, Finish. Publ. Ltd., ASM Int., 6th edition, 2001, pp. 327–400, 405–410, 744–818 and 985 Search PubMed.
- H. Takahashi, in ASM Handbook Corrosion: Fundamentals, Testing, and Protection, 2003, pp. 736–740 Search PubMed.
- R. Zhang, K. Jiang and G. Ding, Surface morphology control on porous anodic alumina in phosphoric acid, Thin Solid Films, 2010, 518, 3797–3800 CrossRef CAS.
- M. Porta-i-Batalla, E. Xifré-Pérez, C. Eckstein, J. Ferré-Borrull and L. F. Marsal, 3D nanoporous anodic alumina structures for sustained drug release, Nanomaterials, 2017, 7, 227–239 CrossRef PubMed.
- S. McGinty, T. T. N. Vo, M. Meere, S. McKee and C. McCormick, Some design considerations for polymer-free drug-eluting stents: A mathematical approach, Acta Biomater., 2015, 18, 213–225 CrossRef CAS PubMed.
- S. Y. Liau, D. C. Read, W. J. Pugh, J. R. Furr and A. D. Russell, Interaction of silver nitrate with readily identifiable groups: Relationship to the antibacterial action of silver ions, Lett. Appl. Microbiol., 1997, 25, 279–283 CrossRef CAS PubMed.
- P. Singh, Y. J. Kim, H. Singh, C. Wang, K. H. Hwang, M. E. A. Farh and D. C. Yang, Biosynthesis, characterization, and antimicrobial applications of silver nanoparticles, Int. J. Nanomed., 2015, 10, 2567–2577 CAS.
- C. Greulich, D. Braun, A. Peetsch, J. Diendorf, B. Siebers, M. Epple and M. Köller, The toxic effect of silver ions and silver nanoparticles towards bacteria and human cells occurs in the same concentration range, RSC Adv., 2012, 2, 6981–6987 RSC.
- G. Mulley, A. T. A. Jenkins and N. R. Waterfield, Inactivation of the antibacterial and cytotoxic properties of silver ions by biologically relevant compounds, PLoS One, 2014, 9, 2–10 CrossRef PubMed.
- E. D. Cavassin, L. F. P. de Figueiredo, J. P. Otoch, M. M. Seckler, R. A. de Oliveira, F. F. Franco, V. S. Marangoni, V. Zucolotto, A. S. S. Levin and S. F. Costa, Comparison of methods to detect the in vitro activity of silver nanoparticles (AgNP) against multidrug resistant bacteria, J. Nanobiotechnol., 2015, 13, 1–16 CrossRef PubMed.
- C. Krishnaraj, E. G. Jagan, S. Rajasekar, P. Selvakumar, P. T. Kalaichelvan and N. Mohan, Synthesis of silver nanoparticles using Acalypha indica leaf extracts and its antibacterial activity against water borne pathogens, Colloids Surf., B, 2010, 76, 50–56 CrossRef CAS PubMed.
- S. Wessels and H. Ingmer, Modes of action of three disinfectant active substances: A review, Regul. Toxicol. Pharmacol., 2013, 67, 456–467 CrossRef CAS PubMed.
- T. Ikeda, H. Hirayama, H. Yamaguchi, S. Tazuke and M. Watanabe, Polycationic biocides with pendant active groups: Molecular weight dependence of antibacterial activity, Antimicrob. Agents Chemother., 1986, 30, 132–136 CrossRef CAS PubMed.
- J. He, E. Söderling, P. K. Vallittu and L. V. J. Lassila, Investigation of double bond conversion, mechanical properties, and antibacterial activity of dental resins with different alkyl chain length quaternary ammonium methacrylate monomers (QAM), J. Biomater. Sci., Polym. Ed., 2013, 24, 565–573 CrossRef CAS PubMed.
- M. Kong, X. G. Chen, K. Xing and H. J. Park, Antimicrobial properties of chitosan and mode of action: A state of the art review, Int. J. Food Microbiol., 2010, 144, 51–63 CrossRef CAS PubMed.
- P. Gilbert and L. E. Moore, Cationic antiseptics: Diversity of action under a common epithet, J. Appl. Microbiol., 2005, 99, 703–715 CrossRef CAS PubMed.
- M. Tischer, G. Pradel, K. Ohlsen and U. Holzgrabe, Quaternary ammonium salts and their antimicrobial potential: Targets or nonspecific interactions?, ChemMedChem, 2012, 7, 22–31 CrossRef CAS PubMed.
- B. Gottenbos, D. W. Grijpma, H. C. Van Der Mei, J. Feijen and H. J. Busscher, Antimicrobial effects of positively charged surfaces on adhering Gram-positive and Gram-negative bacteria, J. Antimicrob. Chemother., 2001, 48, 7–13 CrossRef CAS PubMed.
- A. Cavallaro, A. Mierczynska, M. Barton, P. Majewski and K. Vasilev, Influence of immobilized quaternary ammonium group surface density on antimicrobial efficacy and cytotoxicity, Biofouling, 2016, 32, 13–24 CrossRef CAS PubMed.
- D. R. Perinelli, D. Petrelli, D. Vllasaliu, L. A. Vitali, G. Bonacucina, M. Cespi, G. Giorgioni and G. F. Palmieri, Quaternary ammonium leucine-based surfactants: The effect of a benzyl group on physicochemical properties and antimicrobial activity, Pharmaceutics, 2019, 11, 287–288 CrossRef CAS PubMed.
- Y. Jiao, L. Niu, S. Ma, J. Li, F. R. Tay and J. Chen, Progress in Polymer Science Quaternary ammonium-based biomedical materials: State-of-the-art, toxicological aspects and antimicrobial resistance, Prog. Polym. Sci., 2017, 71, 53–90 CrossRef CAS PubMed.
- Y. Jiao, L. Niu, S. Ma, J. Li, F. R. Tay and J. Chen, Quaternary ammonium-based biomedical materials: State-of-the-art, toxicological aspects and antimicrobial resistance, Prog. Polym. Sci., 2017, 71, 53–90 CrossRef CAS PubMed.
- C. J. Ioannou, G. W. Hanlon and S. P. Denyer, Action of disinfectant quaternary ammonium compounds against Staphylococcus aureus, Antimicrob. Agents Chemother., 2007, 51, 296–306 CrossRef CAS PubMed.
- Y. H. Xiao, J. H. Chen, M. Fang, X. D. Xing, H. Wang, Y. J. Wang and F. Li, Antibacterial effects of three experimental quaternary ammonium salt (QAS) monomers on bacteria associated with oral infections, J. Oral Sci., 2008, 50, 323–327 CrossRef CAS PubMed.
- J. Li, Z. Sha, W. Zhang, F. Tao and P. Yang, Preparation and antibacterial properties of gelatin grafted with an epoxy silicone quaternary ammonium salt, J. Biomater. Sci., Polym. Ed., 2016, 27, 1017–1028 CrossRef CAS PubMed.
- D. R. Perinelli, D. Petrelli, L. A. Vitali, D. Vllasaliu, M. Cespi, G. Giorgioni, E. Elmowafy, G. Bonacucina and G. F. Palmieri, Quaternary ammonium surfactants derived from leucine and methionine: Novel challenging surface active molecules with antimicrobial activity, J. Mol. Liq., 2019, 283, 249–256 CrossRef CAS.
- A. D. Russell, W. B. Hugo and G. A. J. Ayliffe's, Principles and Practice of Disinfection, Preservation & Sterilization, John Wiley, Hoboken, USA, 2008 Search PubMed.
- A. Muñoz-Bonilla and M. Fernández-García, Polymeric materials with antimicrobial activity, Prog. Polym. Sci., 2012, 37, 281–339 CrossRef.
- B. Hota, Contamination, disinfection, and cross-colonization: Are hospital surfaces reservoirs for nosocomial infection?, Clin. Infect. Dis., 2004, 39, 1182–1189 CrossRef PubMed.
- D. J. Weber, W. A. Rutala, M. B. Miller, K. Huslage and E. Sickbert-Bennett, Role of hospital surfaces in the transmission of emerging health care-associated pathogens: Norovirus, Clostridium difficile, and Acinetobacter species, Am. J. Infect. Control, 2010, 38, S25–S33 CrossRef PubMed.
- D. J. Weber, D. Anderson and W. A. Rutala, The role of the surface environment in healthcare-associated infections, Curr. Opin. Infect. Dis., 2013, 26, 338–344 CrossRef PubMed.
- S. L. Warnes, S. M. Green, H. T. Michels and C. W. Keevil, Biocidal efficacy of copper alloys against pathogenic enterococci involves degradation of genomic and plasmid DNAs, Appl. Environ. Microbiol., 2010, 76, 5390–5401 CrossRef CAS PubMed.
- A. Różzańska, A. Chmielarczyk, D. Romaniszyn, A. Sroka-Oleksiak, M. Bulanda, M. Walkowicz, P. Osuch and T. Knych, Antimicrobial properties of selected copper alloys on Staphylococcus aureus and Escherichia coli in different simulations of environmental conditions: With vs. without organic contamination, Int. J. Environ. Res. Public Health, 2017, 14, 813–838 CrossRef PubMed.
- L. Weaver, H. T. Michels and C. W. Keevil, Survival of Clostridium difficile on copper and steel: futuristic options for hospital hygiene, J. Hosp. Infect., 2008, 68, 145–151 CrossRef CAS PubMed.
- M. K. Paczosa and J. Mecsas, Klebsiella pneumoniae: Going on the Offense with a Strong Defense, Microbiol. Mol. Biol. Rev., 2016, 80, 629–661 CrossRef CAS PubMed.
- C. Opoku-Temeng, S. D. Kobayashi and F. R. DeLeo, Klebsiella pneumoniae capsule polysaccharide as a target for therapeutics and vaccines, Comput. Struct. Biotechnol. J., 2019, 17, 1360–1366 CrossRef CAS PubMed.
- M. A. Bachman, P. Breen, V. Deornellas, Q. Mu, L. Zhao, W. Wu, J. D. Cavalcoli and H. L. T. Mobley, Genome-wide identification of Klebsiella pneumoniae fitness genes during lung infection, mBio, 2015, 6, 1–9 CrossRef PubMed.
- M. Rosenberg, H. Vija, A. Kahru, C. Keevil and A. Ivask, Rapid in situ assessment of Cu-ion mediated effects and antibacterial efficacy of copper surfaces, Sci. Rep., 2018, 8, 1–12 CAS.
- C. E. Santo, E. W. Lam, C. G. Elowsky, D. Quaranta, D. W. Domaille, C. J. Chang and G. Grass, Bacterial killing by dry metallic copper surfaces, Appl. Environ. Microbiol., 2011, 77, 794–802 CrossRef CAS PubMed.
- Durham University, Metal-Related Antimicrobials Showcase Event, http://www.ncbi.nlm.nih.gov/pubmed/28773856, accessed 26 April 2019 Search PubMed.
- V. C. C. Cheng, P. H. Chau, W. M. Lee, S. K. Y. Ho, D. W. Y. Lee, S. Y. C. So, S. C. Y. Wong, J. W. M. Tai and K. Y. Yuen, Hand-touch contact assessment of high-touch and mutual-touch surfaces among healthcare workers, patients, and visitors, J. Hosp. Infect., 2015, 90, 220–225 CrossRef CAS PubMed.
- United States Environmental Protection Agency (US EPA), Protocol for the evaluation of bactericidal activity of hard, non-porous copper/copper-alloy surfaces, 02/03/15, 2015, pp. 5–13 Search PubMed.
- C. L. Silva, D. Topgaard, V. Kocherbitov, J. J. S. Sousa, A. A. C. C. Pais and E. Sparr, Stratum corneum hydration: Phase transformations and mobility in stratum corneum, extracted lipids and isolated corneocytes, Biochim. Biophys. Acta, Biomembr., 2007, 1768, 2647–2659 CrossRef CAS PubMed.
- A. L. Byrd, Y. Belkaid and J. A. Segre, The human skin microbiome, Nat. Rev. Microbiol., 2018, 16, 143–155 CrossRef CAS PubMed.
- D. D. Balci, N. Duran, B. Ozer, R. Gunesacar, Y. Onlen and J. Z. Yenin, High prevalence of Staphylococcus aureus cultivation and superantigen production in patients with psoriasis, Eur. J. Dermatol., 2009, 19, 238–242 CAS.
- H. Brüggemann, Insights in the pathogenic potential of Propionibacterium acnes from its complete genome, Semin. Cutaneous Med. Surg., 2005, 24, 67–72 CrossRef PubMed.
- INSPQ, COVID-19: Nettoyage et désinfection de surfaces résidentiels et les lieux publics concernant le virus, Inst. Natl. Santé Publique du Québec, 2020, pp. 1–9 Search PubMed.
- R. S. Boethling and D. G. Lynch, in Detergents, Springer Berlin Heidelberg, Berlin, Heidelberg, 1992, pp. 145–177 Search PubMed.
- U.S. EPA: U.S. Environmental Protection Agency, Reregistration Eligibility Decision (RED) for Alkyl Dimethyl Benzyl Ammonium Chloride (ADBAC), 2006 Search PubMed.
- U.S. EPA: U.S. Environmental Protection Agency, Reregistration Eligibility Decision for Aliphatic Alkyl Quaternaries (DDAC), 2006 Search PubMed.
- J. M. Campos-Martin, G. Blanco-Brieva and J. L. G. Fierro, Hydrogen peroxide synthesis: An outlook beyond the anthraquinone process, Angew. Chem., Int. Ed., 2006, 45, 6962–6984 CrossRef CAS PubMed.
- T. Du, A. Vijayakumar and V. Desai, Effect of hydrogen peroxide on oxidation of copper in CMP slurries containing glycine and Cu ions, Electrochim. Acta, 2004, 49, 4505–4512 CrossRef CAS.
- H. Jo, S. Lee, D. Kim and J. Lee, Low temperature sealing of anodized aluminum alloy for enhancing corrosion resistance, Materials, 2020, 13, 1–12 Search PubMed.
- S. Wernick, R. Pinner and P. Sheasby, The Surface Treatments of Aluminium and Its Alloys, OH, USA, 5th edn, 1987 Search PubMed.
- C. Adlhart, J. Verran, N. F. Azevedo, H. Olmez, M. M. Keinänen-Toivola, I. Gouveia, L. F. Melo and F. Crijns, Surface modifications for antimicrobial effects in the healthcare setting: a critical overview, J. Hosp. Infect., 2018, 99, 239–249 CrossRef CAS PubMed.
- M. Ojeil, C. Jermann, J. Holah, S. P. Denyer and J. Y. Maillard, Evaluation of new in vitro efficacy test for antimicrobial surface activity reflecting UK hospital conditions, J. Hosp. Infect., 2013, 85, 274–281 CrossRef CAS PubMed.
Footnote |
† Electronic supplementary information (ESI) available. See DOI: 10.1039/d1ra07159a |
|
This journal is © The Royal Society of Chemistry 2021 |