DOI:
10.1039/D1RA06569F
(Paper)
RSC Adv., 2021,
11, 38814-38819
The interesting luminescence behavior and rare nonlinear optical properties of the {Ag55Mo6} nanocluster†
Received
1st September 2021
, Accepted 30th November 2021
First published on 3rd December 2021
Abstract
The remarkably reversible thermochromic luminescence behavior and the rare nonlinear optical (NLO) properties of the [Ag55(MoO4)6(C
CtBu)24(CH3COO)18(CH3COO)]·2H2O ({Ag55Mo6} for short) nanocluster reported were investigated experimentally. The important contributions of Ag+, C
C− ions and MoO42− groups to the NLO properties were proved by further density functional theory (DFT) calculations.
Research into high-nuclearity transition-metal clusters has become increasingly intensive due to their fascinating structures and extensive applications.1,2 As for high-nuclearity silver clusters, although many wonderful structures have been discovered, studies of their properties are very few. Therefore, to maximize application of high-nuclearity silver clusters, the research of their physical/chemical properties is highly desirable.3,4
On one hand, studying luminescence properties of high-nuclearity silver clusters has great scientific significance to the development of smart optical materials. In recent years, research on the luminescence property of high-nuclearity silver clusters has been actively pursued because of their numerous potential applications in light emitting devices.5,6 As far as we know, silver chalcogenolate clusters have excellent luminescence properties.7 Wang, Zang and Sun et al. have reported some temperature-sensitive luminescent silver chalcogenolate clusters, which emit weak lights at room temperature while emit bright lights at 77 K.6,8 For example, a polyoxometallate (POM for short) functionalized multiple core–shell Ag84 nanocluster [Ag10@(W7O26)2@Ag74S2(iPrS)40(nPrCOO)18]·2CH3OH shows the temperature-dependent emission property.6 Recently, Zang and Sun et al. have reported turn-on luminescence9 and aggregation-induced emission in silver chalcogenolate cluster metal–organic frameworks/gels.10 However, there is very little research on the luminescence property of high-nuclearity silver alkynyl clusters.11 Firstly, a new silver alkynyl nanocluster [Ag42{Eu(W5O18)2}(tBuC
C)28Cl4][OH]·H2O has been reported by Gao, and this compound exhibits an unusual fluorescence enhancement superior to the polyoxoanionic [Eu(W5O18)2]9− precursor.12 Besides, Wang et al. have reported two unprecedented solution-stable silver alkynyl clusters, [Ag7(C2)(CF3CO2)5(bpy)5]·MeCN·0.5MeOH and [Ag7(C2)(CF3CO2)5(phen)5]·2CH2Cl2. Both of the two compounds are not emissive at room temperature, while the frozen glasses of them (MeCN/MeOH, 1
:
1) are luminescent at 77 K.13 A novel silver-alkynyl cluster based NbO-type framework [Cl@Ag18(cPrC
C)17BF4]n showing unprecedented triple emission spanning from the visible to near-infrared (NIR) region has been reported by Sun.14 The above studies provide positive guidance for the luminescence exploration of silver alkynyl clusters.
On the other hand, studying the nonlinear optical (NLO) properties of silver alkynyl clusters also has great scientific significations to the development of smart optical materials. It is well known that NLO materials with abundant delocalized π electrons and ultrafast NLO response act as an important role in optoelectronic technologies and all-optical switch regions, thus much attention has been focused on investigate the NLO responses of metal clusters. Metal ions and organic ligands have been proved to be two important factors for the NLO original mechanism of metal clusters.15,16 The reason why metal clusters have large third-order NLO properties is that more electronic transitions can be allowed to take place after heavy-metal ions introduction.17 In silver alkynyl clusters with heavy-metal silver involved, d–pπ delocalized and d–dπ conjugated characteristics of alkynyl ligand make them serve as excellent NLO materials.18,19 Currently, although the NLO properties of many metal clusters have been studied, but there are few studies on the NLO properties of silver clusters.19–23 Given this, it is essential to explore the relationship of structures and NLO properties of high-nuclearity silver alkynyl nanoclusters.
The ultrastable [Ag55(MoO4)6(C
CtBu)24(CH3COO)18(CH3COO)]·2H2O ({Ag55Mo6}) silver alkynyl nanocluster possessing a Ag-centered multishell structure has been chemically well-defined and characterized by our group in 2014.24 In this paper, we will focus on the optical properties of the {Ag55Mo6} nanocluster. Luminescence spectra of the {Ag55Mo6} nanocluster from 298 K to 77 K were measured. The results indicate that the {Ag55Mo6} nanocluster presents reversible thermochromic luminescence behavior, which is rare in the known inorganic–organic silver alkynyl hybrids. And the NLO property of the {Ag55Mo6} nanocluster was investigated by femtosecond Z-scan technique and its molecular frontier orbitals were simulated by quantum chemical methods. These results indicate {Ag55Mo6} has a good application prospect for fluorescent probe and NLO materials.
Luminescence property
Luminescence property of the {Ag55Mo6} nanocluster in the solid state was investigated by an F-4500 Fluorescence (FL) Spectrophotometer. It shows a maximum emission peak at 439 nm accompanied by a shoulder peak at 418 nm upon the excitation wavelength of 365 nm at 298 K (Fig. 1a, violet trace). The short lifetime of the maximum emission at 439 nm was measured to be ∼14.0 ns, suggesting the emission is fluorescence. The quantum efficiency of the photoluminescence is ∼0.1%. Interestingly, it also emits orange light at 77 K, exhibiting a maximum emission peak at 608 nm upon the excitation wavelength of 365 nm (Fig. 1a, orange trace). By comparing the two emission spectra at 298 K and at 77 K, it can be found that the intensity of the emission peak at 608 nm gradually increases while the emission peak at 439 nm gradually weakens as the temperature decreases from 298 K to 77 K (Fig. 1a and S1†). To investigate the change of emission peak intensity at 608 nm, temperature dependent luminescence spectra of the {Ag55Mo6} nanocluster were recorded at 298, 255, 173, 130 and 77 K by a combined fluorescence lifetime and steady state spectrometer FLSP920. The maximum emission band of the {Ag55Mo6} nanocluster at 608 nm was still kept upon a series of excitation wavelengths (365 nm, 378 nm, 421 nm and 467 nm) at low temperature. As shown in Fig. 1 and S2,† the emission peak intensity of the {Ag55Mo6} nanocluster at 608 nm stepwise increases upon excitation at 421 nm when the temperature cooling from 298 to 77 K. To explore the luminescence mechanism of the {Ag55Mo6} nanocluster, luminescence properties of AgC
CtBu ligand and the mixture of initial reaction materials were investigated. As shown in Fig. S3 and S4,† at both 298 K and 77 K, no emission peaks around 608 nm were detected from their luminescence spectra, and not to mention intensity. Therefore, we infer the thermochromic luminescence behavior of the {Ag55Mo6} nanocluster should be assigned to the characteristic of the {Ag55Mo6} nanocluster. With reference to the spectra of related silver clusters,25 the emission of the {Ag55Mo6} nanocluster at 298 K should be a ligand-to-metal-charge-transfer (LMCT) transition combined with the cluster-centered (CC) transition disturbed by Ag⋯Ag interactions. While the emission of the {Ag55Mo6} nanocluster at low temperature should be attributable to the CC excited state. Ag⋯Ag distance thermal compression occurred in the {Ag55Mo6} nanocluster leading to enhanced rigidity and faster intersystem crossing process as the temperature decreases, effectively reducing the energy loss of non-radiation decay. As a result, the CC emission stepwise strengthens from 298 to 77 K. The thermochromic luminescence behavior of the {Ag55Mo6} nanocluster is reversible between 298 and 77 K. As well as known, fluorescence of POMs is very weak. Surprisingly, the {Ag55Mo6} nanocluster embedded by six MoO42− POM building units possesses such an excellent luminescence behavior, which is rare in the known inorganic–organic silver alkynyl hybrids. Owing to its eye-detected temperature-sensitive emission, the {Ag55Mo6} nanocluster has great potential for light-emitting materials, sensor for temperatures and temperature probes.
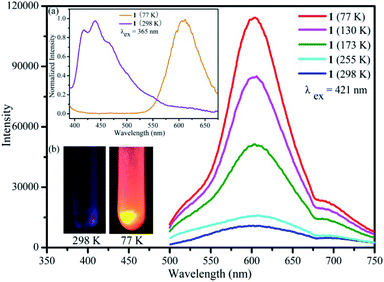 |
| Fig. 1 Emission spectra of the {Ag55Mo6} nanocluster at different temperatures upon excitation at 421 nm. Inset (a) emission (violet trace) and (orange trace) spectra of the {Ag55Mo6} nanocluster at 298 and 77 K upon excitation at 365 nm; (b) luminescence photographs of the {Ag55Mo6} nanocluster at 298 and 77 K excited with a hand-held UV lamp (365 nm). | |
Third-order nonlinear optical property
The linear absorption spectrum of the {Ag55Mo6} nanocluster in CH3OH solution is presented in Fig. 2. While the excitation wavelength of the laser light is 400 nm, which satisfies the non-resonant absorption condition in Z-scan measurement.26
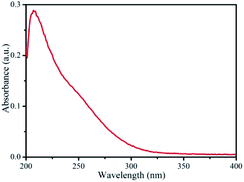 |
| Fig. 2 Absorption spectrum of the {Ag55Mo6} nanocluster in CH3OH solution. | |
The third-order NLO property of the {Ag55Mo6} nanocluster with the concentration of 9.0 × 10−5 mol L−1 was investigated under 190 fs laser pulses with the wavelength of 400 nm. Z-scan data (open-aperture and closed aperture data) of the {Ag55Mo6} nanocluster dissolved in the CH3OH solution are shown in Fig. 3. The results indicate that the {Ag55Mo6} nanocluster shows very strong NLO responses included nonlinear absorptive and refractive effects. The nonlinear absorption component was evaluated by open-aperture Z-scan (Fig. 3a). The open-aperture Z-scan experimental data of the {Ag55Mo6} nanocluster can be well described by eqn (1) and (2),27 which can also explain the nonlinear absorption process:
|
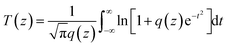 | (1) |
|
 | (2) |
where light transmittance
T represents a function of the sample's
Z-position (with respect to focal point
z = 0),
I0 represents the peak irradiation intensity at focus,
L represents the sample thickness,
z represents the distance of the sample from the focal point,
z0 = π
ω02/
λ (where
ω0 represents the spot radius of the laser pulse at focus and
λ represents the laser wavelength),
t represents the time,
t0 represents the pulse width,
r represents the radial coordinate,
α0 and
β represent linear absorption coefficient and effective third-order NLO absorptive coefficient, respectively. In
Fig. 3a, it is clear to be observed that the normalized transmittance of the {Ag
55Mo
6} nanocluster decreases when it is brought closer to the focal point (
z = 0). It suggests that the {Ag
55Mo
6} nanocluster exhibits strong reverse saturable absorption (RSA). Moreover, the NLO absorptive coefficient (
β) of the {Ag
55Mo
6} nanocluster was calculated to be 6.2 × 10
−12 m
2 W
−1 through curve fitting, shown in
Fig. 3a. As for NLO refractive effect, the {Ag
55Mo
6} nanocluster performed large self-focusing effect for valley-peak configuration, shown in
Fig. 3b.
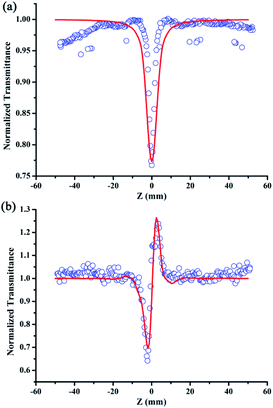 |
| Fig. 3 Z-scan measurement of the {Ag55Mo6} nanocluster in CH3OH solution at 400 nm with the linear transmittance of 60% at 13 μJ pulse: (a) data collected under the open-aperture configuration. (b) Data obtained by dividing the normalized Z-scan data obtained under the closed-aperture configuration by the normalized Z-scan data in (a). Solid lines represent theoretical fits. | |
In addition, in order to eliminate the nonlinear refraction of solvent CH3OH, the nonlinear refraction Z-scan curve of solvent CH3OH shown in Fig. 4 was carried out under the same experimental condition with the one of {Ag55Mo6} nanocluster performed in Fig. 3a. From Fig. 4, the solvent CH3OH also performed nonlinear refraction effect. As a result, when considering the pure NLO effect of {Ag55Mo6} nanocluster, the NLO effect of solvent CH3OH should be subtracted. An effective third-order NLO refractive index n2 of the {Ag55Mo6} nanocluster can be obtained from the difference between the normalized transmittance values at the valley and peak positions (ΔTV–P) using eqn (3),27
|
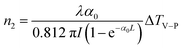 | (3) |
where
I represents the light intensity of incident pulse, and
λ represents the wavelength of the laser. The NLO refractive indices (
n2) of the {Ag
55Mo
6} nanocluster solution and solvent CH
3OH were estimated to be 3.7 × 10
−19 m
2 W
−1 and 1.1 × 10
−19 m
2 W
−1 through curve fitting, respectively. Then the pure NLO refractive index (
n2) of the {Ag
55Mo
6} nanocluster was estimated to be 2.6 × 10
−19 m
2 W
−1.
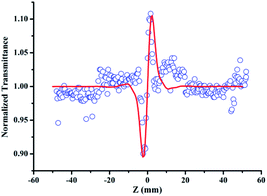 |
| Fig. 4 Data collected under the closed-aperture configuration of CH3OH solution at 400 nm with the linear transmittance of 60% at 13 μJ pulse. Solid lines represent theoretical fits. | |
The real and imaginary parts of χ(3) of the {Ag55Mo6} nanocluster can be obtained by eqn (4) and (5):28
|
Reχ(3) = cn02n2/120π2
| (4) |
|
Imχ(3) = c2n02β/240π2ω
| (5) |
Thus, the coefficient of the effective third-order susceptibility χ(3) was 1.63 × 10−13 esu.
The corresponding coefficient of the hyper-polarizability γ can be calculated by the following eqn (6),29
where
N represents the number density of the {Ag
55Mo
6} nanocluster in the sample solution, is the local field correction factor and the
n0 represents the refractive index of the solvent. Thus, the coefficient of the hyper-polarizability
γ is determined to be 1.22 × 10
−30 esu, which is comparable to silver clusters and other metal complexes,
19–23,30–34 indicating it can be used for the application of NLO domain.
Density functional theory (DFT) calculations
To further explore the origin of the third-order NLO property, the electronic structures of the {Ag55Mo6} nanocluster were simultaneously investigated via theoretical calculations (Fig. 5).
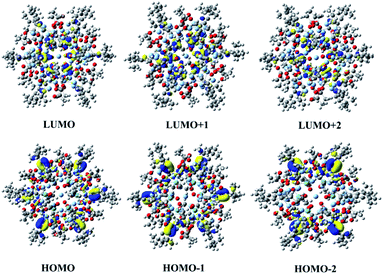 |
| Fig. 5 The frontier molecular orbitals including HOMO−2, HOMO−1, HOMO, LUMO, LUMO+1 and LUMO+2 of the [Ag55(MoO4)6(C CtBu)24(CH3COO)18]+ cluster skeleton in the {Ag55Mo6} nanocluster. | |
This {Ag55Mo6} nanocluster is so large that it is difficult to give a common simulation using density functional theory (DFT), thereby we employed the typical functional B3LYP, 3-21G basis set for non-metal atoms and LAND2DZ basis set for Ag and Mo atoms to carry out single-point calculation in order to obtain the electronic structures.35 The frontier molecular orbitals including the highest occupied molecular orbital (HOMO), HOMO−1, HOMO−2, the lowest unoccupied molecular orbital (LUMO), LUMO+1 and LUMO+2 are shown in Fig. 5. Obviously, the occupied molecular orbitals mainly distribute at C
C− and Ag+ ions, while the unoccupied molecular orbitals are mainly localized at MoO42− groups, which suggest the obvious charge transfer characterization when this nanocluster is excited by the photon. As we all know it, the charge transfer is favourable for the NLO response, thus it can be determined that the contribution of the Ag+ and C
C− ions and the MoO42− groups to the NLO property of the {Ag55Mo6} nanocluster by molecular orbital theory. Therefore, we can deduce that the NLO property of the {Ag55Mo6} nanocluster is controlled by the Ag55Mo6 and C
C− ions. This finding is consistent with the experimental results: the {Ag55Mo6} nanocluster performs strong reverse saturable absorption (RSA) and self-focusing nonlinear refractive effect in our Z-scan measurements. The observed NLO effect can be attributed to the two-photon absorption (TPA) and the excited-state absorption induced by TPA in this case.16,36
An ideal optical limiting (OL) material should have these characteristics: the ability of responding quickly to the incident light and increasing opaque as the light intensity increases. The {Ag55Mo6} nanocluster displays such an OL ability for the ultrafast strong NLO response and showing transparent under low light intensity.
Conclusion
In summary, the optical properties of the chemically well-defined {Ag55Mo6} nanocluster were investigated. Surprisingly, the {Ag55Mo6} nanocluster presents reversible thermochromic luminescence behavior, which is rare in the known inorganic–organic silver alkynyl hybrids. Moreover, the third-order NLO properties of the {Ag55Mo6} nanocluster have been studied by using the Z-scan technique at 400 nm with 190 fs laser pulses, and the results show the {Ag55Mo6} nanocluster displays strong reverse saturable absorption and its second-order hyperpolarizability γ is estimated to be 1.22 × 10−30 esu. The DFT calculations suggest the important contribution of Ag55Mo6 and C
C− ions to the NLO properties. These results indicate that the {Ag55Mo6} nanocluster has great potential in light emitting devices and ultrafast optical limiter in the near future.
Conflicts of interest
There are no conflicts to declare.
Acknowledgements
This work was supported financially by the 61st batch of China Postdoctoral Science Foundation (No. 415-111900347), the NSFC of China (No. 21671034, 21771035), the Education Department of Liaoning Province Youth Project (No. L2020039), Science and Technology Foundation of Tongren City (Grant No. [2017] 47-37), Startup Foundation for Doctoral Research (trxyDH1622) and Science and Technology Foundation of Guizhou Province Education Department (Grant No. KY[2018]343).
Notes and references
-
(a) A. V. Anyushin, A. Kondinski and T. N. Parac-Vogt, Chem. Soc. Rev., 2020, 49, 382–432 RSC;
(b) S. K. Langley, R. A. Stott, N. F. Chilton, B. Moubaraki and K. S. Murray, Chem. Commun., 2011, 47, 6281–6283 RSC;
(c) X.-J. Kong, L.-S. Long, Z.-P. Zheng, R.-B. Huang and L.-S. Zheng, Acc. Chem. Res., 2010, 43, 201–209 CrossRef CAS PubMed;
(d) G. E. Kostakis and A. K. Powell, Coord. Chem. Rev., 2009, 253, 2686–2697 CrossRef CAS;
(e) G. Mezei, C. M. Zaleski and V. L. Pecoraro, Chem. Rev., 2007, 107, 4933–5003 CrossRef CAS PubMed;
(f) S. Horiuchi, S. Moon, A. Ito, J. Tessarolo, E. Sakuda, Y. Arikawa, G. H. Clever and K. Umakoshi, Angew. Chem., Int. Ed., 2021, 60, 10654–10660 CrossRef CAS PubMed.
-
(a) R. J. Wilson, N. Lichtenberger, B. Weinert and S. Dehnen, Chem. Rev., 2019, 119, 8506–8554 CrossRef CAS PubMed;
(b) X.-Y. Zheng, J. Xie, X.-J. Kong, L.-S. Long and L.-S. Zheng, Coord. Chem. Rev., 2019, 378, 222–236 CrossRef CAS;
(c) C. Papatriantafyllopoulou, E. E. Moushi, G. Christou and A. J. Tasiopoulos, Chem. Soc. Rev., 2016, 45, 1597–1628 RSC;
(d) X. K. Fang, L. Hansen, F. Haso, P. C. Yin, A. Pandey, L. Engelhardt, I. Slowing, T. Li, T. B. Liu, M. Luban and D. C. Johnston, Angew. Chem., Int. Ed., 2013, 52, 10500–10504 CrossRef CAS PubMed;
(e) G. E. Kostakis, S. P. Perlepes, V. A. Blatov, D. M. Proserpio and A. K. Powell, Coord. Chem. Rev., 2012, 256, 1246–1278 CrossRef CAS.
-
(a) J. A. Chipman and J. F. Berry, Chem. Rev., 2020, 120, 2409–2447 CrossRef CAS PubMed;
(b) S.-Q. Zhang and L. Zhao, Acc. Chem. Res., 2018, 51, 2535–2545 CrossRef CAS PubMed;
(c) J.-Z. Yan, B. K. Teo and N.-F. Zheng, Acc. Chem. Res., 2018, 51, 3084–3093 CrossRef CAS PubMed;
(d) A. Ghosh, O. F. Mohammed and O. M. Bakr, Acc. Chem. Res., 2018, 51, 3094–3103 CrossRef CAS PubMed;
(e) Z. Lei, X.-K. Wan, S.-F. Yuan, Z.-J. Guan and Q.-M. Wang, Acc. Chem. Res., 2018, 51, 2465–2474 CrossRef CAS PubMed;
(f) R.-C. Jin, C.-J. Zeng, M. Zhou and Y.-X. Chen, Chem. Rev., 2016, 116, 10346–10413 CrossRef CAS PubMed.
-
(a) X. Fan, F. R. Yuan, D. J. Li, S. Chen, Z. B. Cheng, Z. J. Zhang, S. C. Xiang, S.-Q. Zang, J. Zhang and L. Zhang, Angew. Chem., Int. Ed., 2021, 60, 12949–12954 CrossRef CAS PubMed;
(b) Y.-X. Du, H.-T. Sheng, D. Astruc and M.-Z. Zhu, Chem. Rev., 2020, 120, 526–622 CrossRef CAS PubMed;
(c) V. Sudheeshkumar, K. O. Sulaiman and R. W. J. Scott, Nanoscale Adv., 2020, 2, 55–69 RSC;
(d) S. Maity, D. Bain and A. Patra, Nanoscale, 2019, 11, 22685–22723 RSC;
(e) Y.-M. Su, Z. Wang, C.-H. Tung, D. Sun and S. Schein, J. Am. Chem. Soc., 2021, 143, 13235–13244 CrossRef PubMed.
- M.-M. Zhang, X.-Y. Dong, Z.-Y. Wang, X.-M. Luo, J.-H. Huang, S.-Q. Zang and T. C. W. Mak, J. Am. Chem. Soc., 2021, 143(16), 6048–6053 CrossRef CAS PubMed.
- Z. Wang, H.-T. Sun, M. Kurmoo, Q.-Y. Liu, G.-L. Zhuang, Q.-Q. Zhao, X.-P. Wang, C.-H. Tung and D. Sun, Chem. Sci., 2019, 10, 4862–4867 RSC.
-
(a) X.-Y. Li, H.-F. Su, K. Yu, Y.-Z. Tan, X.-P. Wang, Y.-Q. Zhao, D. Sun and L.-S. Zheng, Nanoscale, 2015, 7, 8284–8288 RSC;
(b) G. Li, Z. Lei and Q.-M. Wang, J. Am. Chem. Soc., 2010, 132, 17678–17679 CrossRef CAS PubMed;
(c) H. Y. Yang, J. Lei, B. H. Wu, Y. Wang, M. Zhou, A. D. Xia, L. S. Zheng and N. F. Zheng, Chem. Commun., 2013, 49, 300–302 RSC.
-
(a) X. Kang and M.-Z. Zhu, Chem. Soc. Rev., 2019, 48, 2422–2457 RSC;
(b) Y.-P. Xie, Y.-L. Shen, G.-X. Duan, J. Han, L.-P. Zhang and X. Lu, Mater. Chem. Front., 2020, 4, 2205–2222 RSC.
-
(a) W.-M. He, Z. Zhou, Z. Han, S. Li, Z. Zhou, L.-F. Ma and S.-Q. Zang, Angew. Chem., Int. Ed., 2021, 60, 8505–8509 CrossRef CAS PubMed;
(b) R.-W. Huang, Y.-S. Wei, X.-Y. Dong, X.-H. Wu, C.-X. Du, S.-Q. Zang and T. C. W. Mak, Nat. Chem., 2017, 9, 689–697 CrossRef CAS PubMed;
(c) X.-Y. Li, Z. Wang, H.-F. Su, S. Feng, M. Kurmoo, C.-H. Tung, D. Sun and L.-S. Zheng, Nanoscale, 2017, 9, 3601–3608 RSC.
-
(a) X.-H. Wu, P. Luo, Z. Wei, Y.-Y. Li, R.-W. Huang, X.-Y. Dong, K. Li, S.-Q. Zang and B.-Z. Tang, Adv. Sci., 2019, 6, 1801304 CrossRef PubMed;
(b) Z.-C. Xie, P.-P. Sun, Z. Wang, H.-G. Li, L.-Y. Yu, D. Sun, M.-J. Chen, Y.-T. Bi, X. Xin and J.-C. Hao, Angew. Chem., Int. Ed., 2020, 59, 9922–9927 CrossRef CAS PubMed.
-
(a) Y.-L. Shen, J.-L. Jin, J.-J. Fang, Z. Liu, J.-L. Shi, Y.-P. Xie and X. Lu, Inorg. Chem., 2021, 60, 6276–6282 CrossRef CAS PubMed;
(b) Q.-S. Wu, F. Bigdeli, F. Rouhani, X.-M. Gao, H. Kaviani, H.-J. Li, W. Wang, K.-G. Liu, M.-L. Hu, Xi.-Q. Cai and A. Morsali, Inorg. Chem., 2021, 60, 1523–1532 CrossRef CAS PubMed.
- C.-Y. Song, D.-F. Chai, R.-R. Zhang, H. Liu, Y.-F. Qiu, H.-D. Guo and G.-G. Gao, Dalton Trans., 2015, 44, 3997–4002 RSC.
- H.-B. Wu, Z.-J. Huang and Q.-M. Wang, Chem.–Eur.
J., 2010, 16, 12321–12323 CrossRef CAS PubMed.
- S.-S. Zhang, H.-F. Su, G.-L. Zhuang, X.-P. Wang, C.-H. Tung, D. Sun and L.-S. Zheng, Chem. Commun., 2018, 54, 11905–11908 RSC.
-
(a) H. W. Hou, Y. L. Wei, Y. L. Song, L. W. Mi, M. S. Tang and L. K. Li, Angew. Chem., Int. Ed., 2005, 44, 6067–6074 CrossRef CAS PubMed;
(b) W. F. Sun, M. M. Bader and T. Carvalho, Opt. Commun., 2003, 215, 185–190 CrossRef CAS;
(c) S. Vagin, M. Barthel, D. Dini and M. Hanack, Inorg. Chem., 2003, 42, 2683–2694 CrossRef CAS PubMed.
-
(a) W. B. Lin, Z. Y. Wang and L. Ma, J. Am. Chem. Soc., 1999, 121, 11249–11250 CrossRef CAS;
(b) H. Chao, R.-H. Li, B.-H. Ye, H. Li, X.-L. Feng and J.-W. Cai, J. Chem. Soc., Dalton Trans., 1999, 3711–3717 RSC.
- H. W. Hou, X. R. Meng, Y. L. Song, Y. T. Fan, Y. Zhu and H. J. Lu, Inorg. Chem., 2002, 41, 4068–4075 CrossRef PubMed.
- S. Shi, W. Ji, S. H. Tang, J. P. Lang and X. Q. Xin, J. Am. Chem. Soc., 1994, 116, 3615–3616 CrossRef.
-
(a) Y. Xu, C. Xu, T. Zhou and C. Cheng, J. Mol. Struct.: THEOCHEM, 2009, 893, 88–92 CrossRef;
(b) K. Zhou, C. Qin, L.-K. Yan, W.-E. Li, X.-L. Wang, H.-N. Wang, K.-Z. Shao and Z.-M. Su, Dyes Pigm., 2015, 113, 299–306 CrossRef;
(c) Y. Chang, W. Q. Li and Y. Y. Jiang, Phys. Lett. A, 2012, 376, 2314–2318 CrossRef.
-
(a) S. A. Khan, D. Senapati, T. Senapati, P. Bonifassi, Z. Fan, A. K. Singh, A. Neeley, G. Hill and P. C. Ray, Chem. Phys. Lett., 2011, 512, 92–95 CrossRef;
(b) R. L. M. Gieseking, Chem. Mater., 2019, 31, 6850–6859 CrossRef.
-
(a) B. K. Teo and Y. H. Xu, Inorg. Chem., 2001, 40, 6794–6801 CrossRef PubMed;
(b) J. F. Zhang, S. C. Meng, Y. L. Song, J. Y. Yang, H. Y. Wei, W. J. Huang, M. P. Cifuentes, M. G. Humphrey and C. Zhang, New J. Chem., 2011, 35, 328–338 RSC;
(c) U. R. Felscia, B. J. M. Rajkumar, M. Nidya and P. Sankar, J. Phys. Chem. A, 2018, 122, 1045–1052 CrossRef PubMed.
-
(a) A. Banerjee, T. K. Ghanty, A. Chakrabarti and C. Kamal, J. Phys. Chem. C, 2012, 116, 193–200 CrossRef;
(b) K. B. Bhavitha, A. K. Nair, S. Perumbilavil, S. Joseph, M. S. Kala, A. Saha, R. A. Narayanan, N. Hameed, S. Thomas, O. S. Oluwafemi and N. Kalarikkal, Opt. Mater., 2017, 73, 695e705 CrossRef;
(c) U. R. Felscia and B. J. M. Rajkumar, Mater. Lett., 2018, 221, 318–321 CrossRef.
-
(a) R. Zhang, Y. Liu, L. Kong and X.-Y. Xu, Spectrochim. Acta, Part A, 2019, 223, 117338 CrossRef PubMed;
(b) X. Chen, J. Tao, G. Zou, W. Su, Q. J. Zhang and P. Wang, ChemPhysChem, 2010, 11, 3599–3603 CrossRef PubMed;
(c) J. H. Li, J. F. Zhang, M. G. Humphrey and C. Zhang, Eur. J. Inorg. Chem., 2013, 328–346 CrossRef.
- K. Zhou, Y. Geng, L.-K. Yan, X.-L. Wang, X.-C. Liu, G.-G. Shan, K.-Z. Shao, Z.-M. Su and Y.-N. Yu, Chem. Commun., 2014, 50, 11934–11937 RSC.
-
(a) M.-L. Chen, X.-F. Xu, Z.-X. Cao and Q.-M. Wang, Inorg. Chem., 2008, 47, 1877–1879 CrossRef PubMed;
(b) M.-X. Yang, L.-J. Chen, S. Lin, X.-H. Chen and H. Huang, Dalton Trans., 2011, 40, 1866–1872 RSC.
-
(a) V. Bonačić-Koutecký and R. Antoine, Nanoscale, 2019, 11, 12436–12448 RSC;
(b) I. Russier-Antoine, F. Bertorelle, N. Calin, Ž. Sanader, M. Krstić, C. Comby-Zerbino, P. Dugourd, P.-F. Brevet, V. Bonačić-Koutecký and R. Antoine, Nanoscale, 2017, 9, 1221–1228 RSC;
(c) B. Bhushan, T. Kundu and B. P. Singh, Opt. Commun., 2012, 285, 5420–5424 CrossRef.
- M. Sheik-Bahae, A. Said, T.-H. Wei, D. J. Hagan and E. W. Van Stryland, IEEE J. Quantum Electron., 1990, 26, 760–769 CrossRef.
- P. B. Chapple, J. Staromlynska, J. A. Hermann and T. J. Mckay, J. Nonlinear Opt. Phys. Mater., 1997, 6, 251–293 CrossRef.
- K. Mashima, M. Tanaka, Y. Kaneda, A. Fukumoto, H. Mizomoto and K. Tani, Chem. Lett., 1997, 411–412 CrossRef.
-
(a) H. El Ouazzani, K. Iliopoulos, M. Pranaitis, O. Krupka, V. Smokal, A. Kolendo and B. Sahraoui, J. Phys. Chem. B, 2011, 115, 1944–1949 CrossRef PubMed;
(b) H.-T. Shi, L.-J. Zhou, A.-Q. Jia, Q. Chen and Q.-F. Zhang, J. Coord. Chem., 2013, 66, 3740–3748 CrossRef.
- K. Iliopoulos, A. El-Ghayoury, H. El Ouazzani, M. Pranaitis, E. Belhadj, E. Ripaud, M. Mazari, M. Sallé, D. Gindre and B. Sahraoui, Opt. Express, 2012, 20, 25311–25316 CrossRef PubMed.
-
(a) Z. G. Xiao, J. F. Ge, R. Sun, Y. Fang, Y. C. She, Z. G. Li, X. Z. Wu, S. Liu, L. Li, Y. J. Jian and Y. L. Song, Opt. Mater., 2018, 83, 300–305 CrossRef;
(b) Z. G. Xiao, J. F. Ge, Z. G. Li, X. Z. Wu, Y. Fang, G. Shi, X. R. Zhang, Y. X. Wang and Y. L. Song, Opt. Mater., 2015, 50, 263–267 CrossRef.
-
(a) B. Cichy, D. Wawrzynczyk, M. Samoc and W. Stręk, J. Mater. Chem. C, 2017, 5, 149–158 RSC;
(b) J.-H. Jia, X.-M. Tao, Y.-J. Li, W.-J. Sheng, L. Han, J.-R. Gao and Y.-F. Zheng, Chem. Phys. Lett., 2011, 514, 114–118 CrossRef.
- Z. G. Li, F. Gao, Z. G. Xiao, G. D. Ao, X. Z. Wu, Y. Fang, Z. Q. Nie, T.-H. Wei, J. Y. Yang, Y. X. Wang, X. R. Zhang, J. L. Zuo and Y. L. Song, Dyes Pigm., 2015, 119, 70e74 CrossRef.
- T. Lu and F. W. Chen, J. Comput. Chem., 2012, 33, 580–592 CrossRef CAS PubMed.
- G. M. Chu, I. Fernández and M. A. Sierra, Chem.–Eur. J., 2013, 19, 5899–5909 CrossRef CAS.
Footnote |
† Electronic supplementary information (ESI) available: Details of experimental and physical measurements. See DOI: 10.1039/d1ra06569f |
|
This journal is © The Royal Society of Chemistry 2021 |