DOI:
10.1039/D1RA05286A
(Paper)
RSC Adv., 2021,
11, 33626-33636
Ca(OH)2-mediated activation of peroxymonosulfate for the degradation of bisphenol S†
Received
9th July 2021
, Accepted 20th September 2021
First published on 14th October 2021
Abstract
Alkaline substances could activate peroxymonosulfate (PMS) for the removal of organic pollutants, but relatively high alkali consumption is generally required, which can cause too high pH of the solution after the reaction and lead to secondary pollution. Within this study, PMS activated by a relatively low dosage of Ca(OH)2 (1 mM) exhibited excellent efficiency in the removal of bisphenol S (BPS). The pH of the solution declined to almost neutral (pH = 8.2) during the reaction period and conformed to the direct emission standards (pH = 6–9). In a typical case, BPS was completely degraded within 240 min and followed the kinetics of pseudo-first-order. The degradation efficiency of BPS depended on the operating parameters, such as the Ca(OH)2, PMS and BPS dosages, initial solution pH, reaction temperature, co-existing anions, humic acid (HA), and water matrices. Quenching experiments were performed to verify that singlet oxygen (1O2) and superoxide radicals (O2˙−) were the predominant ROS. Degradation of BPS has been significantly accelerated as the temperature increased. Furthermore, degradation of BPS could be maintained at a high level across a broad range of pH values (5.3–11.15). The SO4−, NO3− did not significantly impact the degradation of BPS, however, both HCO3− and HA inhibited oxidation of BPS by the Ca(OH)2/PMS system, and Cl− had a dual-edged sword effect on BPS degradation. In addition, based on the 4 identified intermediates, 3 pathways of BPS degradation were proposed. The degradation of BPS was lower in domestic wastewater compared to other naturals waters and ultrapure; nevertheless, up to 75.86%, 77.94% and 81.48% of BPS was degraded in domestic wastewater, Yaohu Lake water and Poyang Lake water, respectively. Finally, phenolic chemicals and antibiotics, including bisphenol A, norfloxacin, lomefloxacin hydrochloride, and sulfadiazine could also be efficiently removed via the Ca(OH)2/PMS system.
1 Introduction
Bisphenols, a large group of compounds which contain two phenol rings, have been extensively adopted in the manufacture of polycarbonate plastics, phenolic and epoxy resins.1 Bisphenol A (BPA) is the most widely used bisphenol compound with more than 4.5 million tons produced each year. However, BPA has been restricted in its products and applications in many countries over the past few decades, since it was founded to display potential endocrine disruption activity in human and animals. Bisphenol S (BPS) had been developed as a “safe BPA substitution” for replacing BPA,2 which inevitably led to the leakage of this substance into the environment. BPS has a shorter half-life than BPA in water and sediment, and a reduced biodegradability.3 However, BPS exhibits similar toxicological effects (genotoxicity and cytotoxicity) as BPA, but it showed stronger estrogenic activity than BPA.4 As the demand for BPS increased, BPS has been frequently detected in surface water and soils and whose concentration (geometric mean: 0.181 mg g−1) was almost as high as that BPA,5 which raised potential risk on ecosystems and human health. Unfortunately, numerous treatment processes, including adsorption, coagulation and biodegradation cannot effectively eliminate BPS from water.2,3,6 Therefore, it is crucial to develop reliable methods with higher BPS removal efficiencies.
Advanced oxidation processes (AOPs) have been extensively applied promising technologies for the degradation of recalcitrant organic pollutants. In particular, hydrogen peroxide (H2O2), peroxymonosulfate (PMS) and persulfate (PS) based AOPs have attracted wide attention. Compared to H2O2 and PS, the PMS is easier to activate due to its dissymmetric structure and shorter length of the O–O bond. In general, PMS can be activated by heat, ultrasonic, and transition metal catalysis.7–9 However, these methods were limited to be widely applied due to the high energy-input or metal leaching problems. Therefore, environmentally friendly approaches for the effective removal of organic pollutants are highly desirable.
Recently, alkaline substances, including borate and Na2CO3 have been confirmed to efficiently activate PMS to generate reactive oxygen species (ROS).10,11 The previous study had shown that acid orange 7, BPA and acetaminophen in water could be efficiently removed by PMS at alkaline conditions.12,13 However, the methods of PMS activation by alkaline substances have shown some drawbacks. Such as, after the reacts of alkali activated PMS system, the pH of solution was generally too high and cannot be directly discharged into the actual environment. Secondary treatment undoubted would increase the cost of water pollution treatment. Therefore, it is urgent to optimize this method by adjusting the dosages of alkaline substances to change the pH of solution after reaction to reach the ideal value.
Ca(OH)2 is also a kind of high-performance alkali reagent used in wastewater treatments. Compared with NaOH (263.16 USD per Ton), borate (727.62 USD per Ton) and Na2CO3 (294.14 USD per Ton), Ca(OH)2 has distinct advantages because of a lower price (77.41 USD per Ton). Therefore, in the present study, the economic Ca(OH)2 was chosen as an activator of PMS for the degradation of BPS. The amount of Ca(OH)2 was strictly controlled to ensure that the solution pH after reaction meets to 6–9. Besides, this study intended to explore the degradation of BPS in the Ca(OH)2/PMS system under various experimental conditions (including Ca(OH)2, PMS and BPS dosages, co-existing anions and HA, initial solution pH and temperature, etc.). In addition, the dominant ROS in the Ca(OH)2/PMS system were identified. Furthermore, it also studied the degradation of BPS in the different water matrices and the application to other pollutions. Moreover, the reaction products and the transformation pathways of BPS were also proposed.
2 Materials and methods
2.1 Materials
BPS, PMS, PS, BPA, H2O2, furfuryl alcohol (FFA), thiamphenicol (TAP), lomefloxacin hydrochloride (LOM), enrofloxacin (ENR), and norfloxacin (NOR), were purchased from Aladdin Chemicals Co. (China). Ca(OH)2, NaCl, NaH2PO4·2H2O, NaNO3, NaHCO3, NaOH, H2SO4, Na2SO4, ethanol (EtOH), tert-butyl alcohol (TBA), ascorbic acid (AA) and L-histidine (L-his), nitro blue tetrazolium (NBT) and p-benzoquinone (BQ) were obtained from Sinopharm (China). Humic acid (HA) was purchased from Xiya Reagent Co. (China). Methanol (EtOH) was supplied by CNW Technologies GmbH (Germany). All chemical reagents were at least of analytical grade or higher purity. Ultrapure water with a resistivity of 18.2 MΩ cm−1 produced from a water purification system was used to prepare all solutions.
2.2 Experimental procedure
Except for the influence of the reaction temperature, all degradation experiments were carried out at a constant temperature (25.0 °C ± 0.5 °C) controlled by a thermostat water bath. In detail, a specific aliquot of BPS and other chemicals substance stock solution were transferred into a 40 mL brown glass bottle, followed by the addition of designated volumes of Ca(OH)2 and PMS, then approximately 1 mL of the reaction solution in a bottle at predetermined time intervals and detected by HPLC. The experiments for the removal of BPA, TAP, NOF, LOM and ENR were carried out under identical conditions. To investigating the influence of the initial pH, the initial pH of the solution was adjusted to a specific pH by using 0.1 M H2SO4 and 0.1 M NaOH solution. For verifying the application of the Ca(OH)2/PMS system in actual water, four different water samples were collected, including Poyang Lake water (the largest freshwater lake of China), Yaohu Lake water, tap water, and domestic water (collected from a university campus). All the above water samples were filtered through 0.45 μm filters before the experiment, the detailed physicochemical properties of these water types are shown in Table S1.† 1.5 mM PMS and 1 mM Ca(OH)2 were added to the real water samples for experiment.
In order to identify the contribution of ROS in BPS degradation by the Ca(OH)2/PMS system, different ROS quenchers were added. AA (5 mM and 10 mM) as ROS scavenger, EtOH (10 mM and 100 mM) as both HO˙ and SO4˙− scavengers, TBA (10 mM and 100 mM) as HO˙ scavenger, L-His (5 mM and 10 mM) and NBT (0.02 mM, 0.05 mM and 0.1 mM) were employed to eliminate 1O2 and O2˙−, respectively. All reactions were independently performed in at least triplicate and the values with the standard deviations were presented in figures.
2.3 Analytical methods
The concentrations of BPS, BPA, TAP, ENR, NOR and LOM were measured by high-performance liquid chromatography (HPLC, 1260 II, Agilent, USA) equipped with an Athena C18 column (150 mm × 2.1 mm, 5 μm). Detailed HPLC parameters for target organic compounds analysis were shown in Table S2.† The remaining concentration of PMS in the solution was determined by iodometry.14 The pH values were measured with a pHS-3C pH meter (Shanghai Lei Co., Ltd.). The concentration of NBT was measured at a fixed wavelength (λNBT = 259 nm) by a UV-3300 UV-vis spectrophotometer (Mapada Inc., Shanghai, China).
The intermediated products were identified by using a liquid chromatography-time-of-flight mass spectrometer (LC-TOF-MS, Xevo G2-XS Qtof, Waters, USA) equipped with an ACQUITY UPLC BEHC18 Column (2.1 mm × 100 mm, 1.7 μm). The column temperature was 40 °C, and the mobile phase consisted of acetonitrile (40%) and 0.1% formic acid in water (60%) with a 0.4 mL min−1 flow rate, and the injection volume was 10 μL. The mass spectra were measured with a scan range of 100–2000 Da in a positive mode.
The percentage of compounds remaining were calculated according to eqn (1):
|
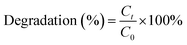 | (1) |
where
C0 is the initial compounds concentration, and
Ct is the compounds concentration at time
t min in the Ca(OH)
2/PMS system.
3 Results and discussion
3.1 Degradation efficiency of BPS in different systems
The performances of different systems on the degradation of 20 μM BPS within 240 min were shown in Fig. 1. No remarkable removal of BPS was observed in the presence of Ca(OH)2 alone, which means that Ca(OH)2 could not directly react with BPS. PMS is a strong oxidant and can directly oxidize tetracyclines.15 However, only 3.57% of BPS was degraded in the single PMS system, which was attributed to the oxidative properties of PMS. In contrast, BPS was almost completely degraded in the Ca(OH)2/PMS system. These results showed that both PMS and Ca(OH)2 were indispensable for the degradation of BPS. The fitting line between ln([Ct/C0]) and reaction time was showed a good linearity (R2 = 0.995), which indicated that the degradation of BPS was well fitted by the pseudo-first-order kinetic model, and the rate constant (kobs) value was 0.0201 min−1. Compared with the Ca(OH)2/PMS system, the Ca(OH)2/PS and Ca(OH)2/H2O2 had no obvious effect on BPS. It could be interpreted that the PMS has an asymmetric molecular structure on both sides of the peroxide bond (–O–O–) inside compared to the PS and H2O2,16 which led to PMS can more easily be activated by Ca(OH)2.
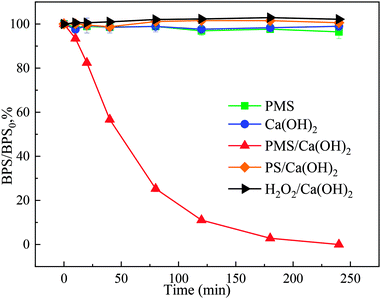 |
| Fig. 1 Degradation efficiency of BPS in the different systems, [Ca(OH)2]0 = 1 mM, [PMS]0 = [PS]0 = [H2O2]0 = 1.5 mM, [BPS]0 = 20 μM. | |
The pH was also monitored as the reaction processes. As shown in Table R3,† the solution pH after the addition of solid PMS dropped to 3.96, the decline of pH was due to large number of H+ produced by PMS dissolved in solution. However, the pH of solution increased to 9.69 with the addition of Ca(OH)2 and finally decreased to 8.20 within 240 min, this phenomenon may be attributed to acidic intermediate products formed. Fortunately, the pH complied with the direct emission standards (6–9) after reaction. It is convenient to practical wastewater treatment without additional pH adjustment.
3.2 ROS identification and possible mechanism of PMS activation
Ca(OH)2 showed excellent performance in PMS activation for BPS degradation. It is necessary to investigate the PMS activation mechanism by Ca(OH)2. Scavenging experiments were used to elucidate the potential involvement of ROS in the Ca(OH)2/PMS system (Fig. 2). as a well-known ROS quencher strongly, AA prohibited the degradation of BPS as shown in Fig. 2(a),17 which suggested that ROS playing an important role in the degradation of BPS. HO˙ and SO4˙− are usually considered to be the main ROS generated in PMS-based AOPs.18 TBA can efficiently quench HO˙ (k = 6 × 108 M−1 s−1), whereas the EtOH can efficiently scavenge both HO˙ (k = 1.2–2.8 × 109 M−1 s−1) and SO4˙− (k = 1.6–7.7 × 107 M−1 s−1).18 With the addition of different concentrations (10 mM and 100 mM) of TBA and EtOH, it was observed that EtOH and TBA have no inhibition effect to the degradation of BPS, indicating that neither HO˙ nor SO4˙− were main ROS involved in the Ca(OH)2/PMS system.
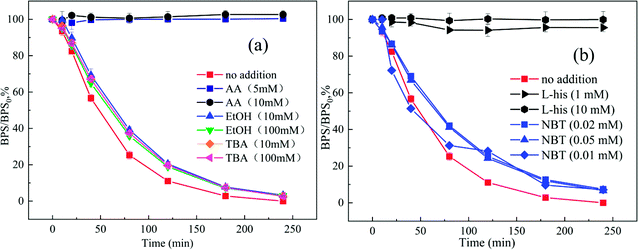 |
| Fig. 2 Effect of AA, EtOH, TBA (a) and L-his, NBT (b) on BPS removal, [Ca(OH)2]0 = 1 mM, [PMS]0 = 1.5 mM, [BPS]0 = 20 μM. | |
Singlet oxygen (1O2) and superoxide radicals (O2˙−) were also proposed to be formed in the PMS activation. L-his is the scavenger of 1O2 (k = 3.2 × 107 M−1 s−1), as shown in Fig. 2(b), the degradation of BPS was intensely inhibited with the addition of L-his, which implied that 1O2 played an essential role in the removal of BPS. NBT was commonly used as a scavenger to trap O2˙− (k = 5.88 × 104 M−1 s−1).20 With the addition of NBT (0.02 mM, 0.05 mM and 0.1 mM), the degradation of BPS was slightly suppressed and decreased from 100% to 92.58%, 93.37% and 92.88%, which implied that O2˙− also participated in the reaction. According to the above-obtained results, the possible activation mechanism of PMS by Ca(OH)2 was proposed as follows:
Previous literature proposed that 1O2 could be slowly generated by self-decomposition of PMS (eqn (2)).19 Besides, under the alkaline conditions, Ca(OH)2 activated PMS generates HO2˙ (eqn (3)), HO2˙ would quickly decompose into O2˙− (eqn (4)). Furthermore, O2˙− generated might be recombined and 1O2 and H2O2 were formed based on eqn (5). Moreover, H2O2 would also generate 1O2 by disproportionation reaction.11 It is worth mentioning that hydrogen ions were continuously generated during reaction, which is also the reason that the pH of solution dropped after reaction.
|
HSO5− + SO52− → HSO4− + SO42− + 1O2
| (2) |
|
3HSO5− + H2O → 2HO2˙ + 3SO42− + 3H+
| (3) |
|
2O2˙− + 2H+ → 1O2 + H2O2
| (5) |
Additionally, to further confirm the generation of O2˙− and 1O2 in Ca(OH)2/PMS system, the selected probe chemical FFA (1 mM) and NBT (1 mM) were employed to detect 1O2 and O2˙−, respectively. As shown in Fig. S1,† the concentration of FFA and NBT decreased as the reaction proceeds. Which implied both 1O2 and O2˙− were involved in the Ca(OH)2/PMS system, and 1O2 played the major role, the O2˙− maybe served as the crucial precursor in the formation of 1O2.
3.3 Degradation of BPS by the Ca(OH)2/PMS system
3.3.1 Influence of Ca(OH)2 concentration. Fig. 3(a) showed that BPS degradation efficiencies with fixed concentration (1.5 mM) of PMS using different Ca(OH)2 concentrations (0–2.00 mM). Only 3.70% of BPS were degraded within 240 min in the presence of 0.25 mM Ca(OH)2, which might be attributed to the unfavorable for PMS activation under low pH condition (pH = 3.66), PMS hardly decomposes to produce ROS under acidic conditions. When the concentrations of Ca(OH)2 were further increased from 0.5 mM to 1 mM, the degradation of BPS increased from 6.42% to 100%. However, when the concentrations were further increased to 1.5 mM and 2 mM, the degradation of BPS were decreased to 82.17% and 38.17%, respectively. A similar trend was also reported in the polyphosphates/PMS process for AO7 degradation.21
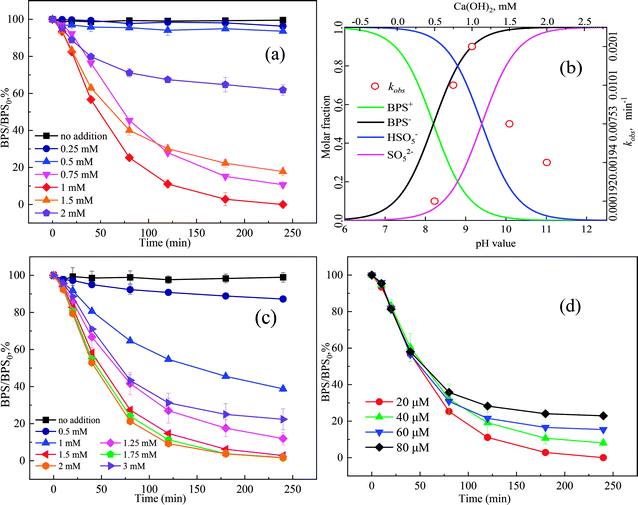 |
| Fig. 3 Removal rate of BPS under different initial Ca(OH)2 concentrations (a), the species of both BPS and PMS in different pH values and changes of kobs under different initial Ca(OH)2 concentrations (b), removal rate of BPS under initial different PMS concentrations (c), the effect of removal rate of BPS at the different BPS concentrations (d), [Ca (OH)2]0 = 0–2 mM, [PMS]0 = 0–3 mM, [BPS]0 = 20–80 μM. | |
The concentrations of Ca(OH)2 play an important in affecting the pH of solution. So, the pKa value of BPS and PMS should be considered. The pKa value of BPS is 8.2,22 therefore, BPS mainly exists in the deprotonated form (BPS−) when the pH > 8.2, and in the protonated form (BPS+) when pH < 8.2 (Fig. 3(b)). Meanwhile, the pKa value of PMS is 9.4, PMS is mainly existing in the form of SO52− when the pH > 9.4, and in the form of HSO5− when the pH < 9.4.23 In addition, the obtained degradation rate constant (kobs) for BPS with different initial Ca(OH)2 concentrations was as follows: 0.00019 min−1 (Ca(OH)2 0.5 mM, pH 8.03) < 0.00194 min−1 (Ca(OH)2 2 mM, pH 10.96) < 0.00753 min−1 (Ca(OH)2 1.5 mM, pH 10.89) < 0.01010 min−1 (Ca(OH)2 0.75 mM, pH 9.24) < 0.02010 min−1 (Ca(OH)2 1 mM, pH 9.69). The kobs value at pH = 9.69 (1 mM Ca(OH)2) was at least 104 times compared to the kobs value at pH = 8.03 (Ca(OH)2 0.5 mM), and the kobs value tended to increase with increasing fraction of SO52− but decreased as the increasing fraction of BPS−. This may be attributed to PMS could generate ROS according to eqn (2)–(6), and PMS decomposed quicker at higher solution pH. However, electrostatic repulsion would be stronger as the increase of SO52− and BPS− concentration, which might result in the decrease of BPS degradation.24 All these indicated that BPS degradation by Ca(OH)2/PMS system exhibited a significant pH dependence, so it is very important to control the dosages of Ca(OH)2.
3.3.2 Influence of PMS concentration. As shown in Fig. 3(c), the effect of different concentrations of PMS on the BPS degradation was also investigated. PMS generally exhibited a positive effect on the target organic contaminant degradation in the Ca(OH)2/PMS system. The degradation of BPS increased from 12.79% to 61.23%, 88.03% and 100%, respectively, as the PMS concentration increased from 0.5 mM to 1 mM, 1.25 mM and 1.5 mM. However, when the PMS concentration continued to increase to 1.75 mM, 2 mM and 3 mM, the BPS degradation significantly decreased to 98.21%, 98.39% and 77.63%, respectively. PMS is the source of ROS, and a certain extent more PMS means more ROS generated.25 Whereas, as the initial concentration of PMS continues increased, the degradation rate of BPS declined instead, the reason may be due to the high PMS concentration in the Ca(OH)2/PMS system resulted self-quenching of ROS, and the generation of low-activity species (SO5˙−),26 then influenced the degradation of BPS. Furthermore, the solution pH decreased to 7.65 with the addition of 3 mM PMS. As illustrated in Section 3.3.1, it was unfavorable for PMS activation in the Ca(OH)2/PMS system when the solution pH was lower than 9.4, thus the dominating role responsible for BPS degradation shifts to the primary solution pH. Given the mentioned issues, 1.5 mM of PMS was chosen for further experiment in the present study.
3.3.3 Influence of PMS adding mode. For further improvement of the oxidation efficiency, the adding mode of PMS was also studied. A total of 1.5 mM PMS was divided equally into n portions (n = 1, 2, 3, 5), which were added sequentially with equal intervals within 240 min as shown in Fig. S2.† With the addition number increased from 1 to 5, the degradation of BPS was decreased from 100% to 77.94%, and the kobs was slowed down from 0.0201 min−1 to 0.00397 min−1. Such a change showed that increasing the addition number of PMS could not result in higher BPS degradation. However, there be reported that the sequential addition of PMS resulted in accelerating the removal of pollutions.27 Compared with metal catalysts, PMS activated by Ca(OH)2 usually consistent and less aggressive because of the slow generation rate of ROS.28 Less addition number of PMS resulted in higher initial PMS dosages in solution, more ROS would be generated and available for the oxidation of BPS, which led to the higher BPS degradation.
3.3.4 Influence of initial BPS concentration. As shown in Fig. 3(d), it was apparent that the efficiency of BPS degradation decreased from 100% to 77.08% with the initial BPS dosages increasing from 20 μM to 80 μM. The continuous consumption of quantitative oxidant (1.5 mM PMS) led to the incomplete removal of high BPS dosages. The kobs for BPS degradation were from 0.02010 min−1 to 0.00660 min−1 with the initial BPS dosages from 20 μM to 80 μM. The decrease in BPS degradation could probably be explained by the fact that the amount of ROS generated at a given PMS concentration was identical. The continuous consumption of quantitative ROS (1.5 mM PMS) caused the incomplete removal of high BPS concentrations.29 At higher initial BPS dosages, the lower ratio of ROS to BPS provided less probability for BPS molecules being attacked by ROS, which led to lower kobs values.30 In addition, as the initial concentration of BPS increased, degradation products would also increase, which increased the competition for ROS.
3.4 Influence of solution temperature
Effect of different reaction temperatures (5 °C, 25 °C, 40 °C, and 50 °C) on the degradation of BPS by the Ca(OH)2/PMS system were investigated (Fig. 4(a)). It was obvious that temperature had a strong influence on the oxidation of BPS. With temperature increased from 5 °C to 25 °C, 40 °C, and 50 °C, BPS degradation was significantly accelerated and the rate climbed from 0.00240 min−1 to 0.20100 min−1, 0.21100 min−1, and 0.59230 min−1, respectively. This phenomenon could be interpreted for the following reason: as temperature increased, the rate of ROS formation was quicker. In addition, the thermal movement of molecules accelerated and the collision probability between molecules increased, thus speeding up the degradation of BPS.31,32 Moreover, the kobs values had a good correlation with the reaction temperature. And the apparent activation energy Ea value for BPS degradation in the Ca(OH)2/PMS system was 90.71 kJ mol−1 according to Arrhenius equation (eqn (7)),33 These results were similar to HPO32+/PMS system removal AO7 (94.4 kJ mol−1).34 The low activation energy implied that the Ca(OH)2/PMS system was promising for heterogeneous catalytic reactions. |
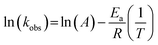 | (7) |
where A represents the pre-exponential factor, Ea represents the activation energy for the reaction (J mol−1), and R represents molar gas constant (8.314 J mol−1 K−1), T represents absolute temperature (K).
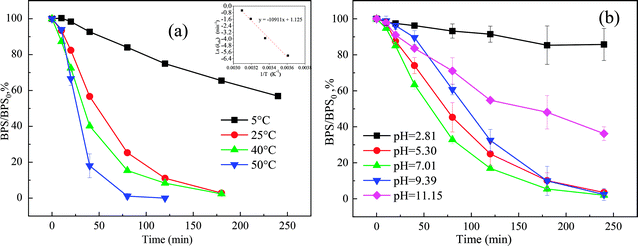 |
| Fig. 4 Removal rate of BPS under different temperatures (a), removal of BPS under different initial pH (b), [Ca(OH)2]0 = 1 mM, [PMS]0 = 1.5 mM, [BPS]0 = 20 μM, temperatures = 5–50 °C, pH = 3.19–11.15. | |
3.5 Influence of initial solution pH
Solution pH affect the oxidation of the target compounds by altering the speciation of both substrate and oxidant. Therefore, initial solution pH usually significantly influences the performance of AOPs in water. Effect of initial solution pH on the removal of BPS was investigated under a series of initial pH conditions (pH = 3.19–11.15) with the results illustrated in Fig. 4(b). The degradation of BPS was only 14.27% and the kobs was 0.00678 min−1 when the initial pH of solution was 3.19, which probably due to the oxidation reaction was ineffective under acidic conditions (pH < 5) in the Ca(OH)2/PMS system. Under acidic conditions, the formation of a strong H-bond between H+ and the O–O bond in PMS was dominated thus inhibited ROS generation.35
As the initial pH changed to 5.30, 7.01 and 9.39, the degradation of BPS degradation increased to 96.50%, 98.03% and 97.62%, respectively, and the kobs was resumed to 0.01400 min−1, 0.01670 min−1 and 0.01530 min−1, respectively. After the addition of Ca(OH)2, the pH of the solution immediately increased from 5.30, 7.01 and 9.39 to 9.44, 10.27 and 10.77, respectively. As interpreted in Section 3.3.1, PMS existed mainly in SO52− when solution pH was higher than 9.4. Therefore, more 1O2 could be generated according to eqn (2)–(6), which allowed BPS to be efficiently degraded. The final pH dropped slightly to 7.64, 9.56 and 9.73, respectively, which was probably due to the decomposition of PMS and the formation of small molecular-weight organic acids as the intermediates of BPS.10
However, when the initial solution pH increased to 11.15, and the final solution pH value dropped to 10.26, the rate of BPS degradation reduced to 63.80% with the kobs decreased to 0.00425 min−1. It indicated that the solution pH in the near-neutral or neutral conditions was more favorable to BPS removal than a stronger alkaline condition. It has been reported that the PMS could be self-decomposed to SO42− and O2 according to eqn (8) at high pH values,11 which resulted in a notable decrease in BPS degradation.
|
2HSO5− + OH− → 2SO42− + H2O + O2
| (8) |
Fortunately, the BPS degradation by Ca(OH)2/PMS system efficiency maintained on a high level in a wide range of pH values, which is favorable to its potential application in the treatment of real wastewater.
3.6 Influence of co-existing water matrix
Anions and natural organic matter (NOM) are widespread in natural water metrices, resulting in a complex aquatic environment. These co-existing substances in water may react with ROS and further inhibit the oxidation of BPS.
As showed in Fig. 5(a), the presence of 1 mM Cl− had slight suppression on BPS degradation, which was similarly observed on the degradation of organic contaminants in PMS-based AOPs.36 Cl− could be oxidized by ROS to generate chlorine-free radicals (Cl˙, Cl2˙−) with lower activity, thereby inhibiting the degradation of BPS.37 However, as the concentration of Cl− further increased to 5 mM and 10 mM, the kobs of BPS were improved from 0.02010 min−1 to 0.02530 min−1 and 0.02780 min−1, respectively. The accelerated BPS degradation in the presence of Cl− could be attributed to the production of reactive chlorine (like HOCl and Cl2) through a two-electron transfer reaction according to eqn (9) and (10).38 Which might participate in the degradation of BPS and accelerated the removal of BPS.
|
Cl− + HSO5− → SO42− + HOCl
| (9) |
|
2Cl− + HSO5− + H+ → SO42− + Cl2 + H2O
| (10) |
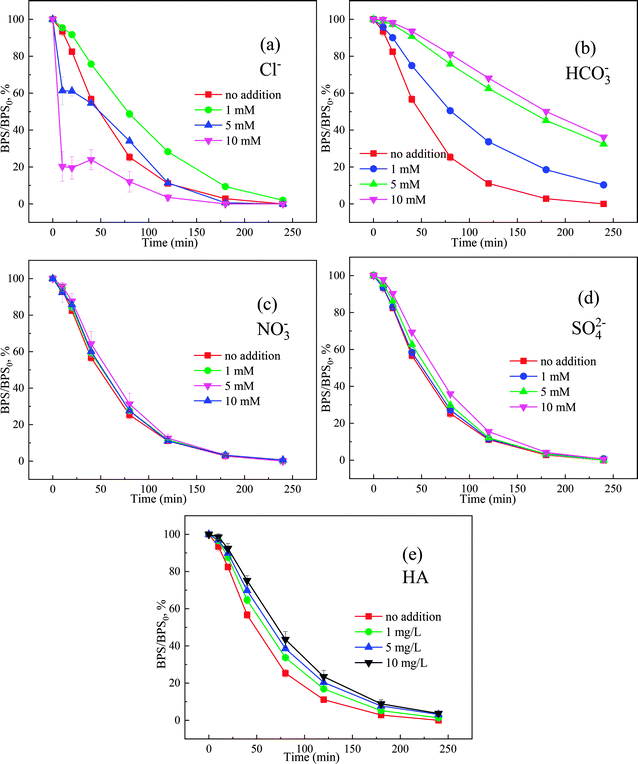 |
| Fig. 5 The effect of Cl− (a), SO42− (b), NO3− (c), HCO3− (d), HA (e) to the Ca(OH)2/PMS system, [Ca(OH)2]0 = 1 mM, [PMS]0 = 1.5 mM, [BPS]0 = 20 μM. | |
Fig. 5(b) showed the influence of HCO3− on BPS removal. The BPS degradation declined to 89.71% in the presence of 1 mM HCO3−, and the degradation rate further declined to 67.58% (5 mM HCO3−) and 63.83% (10 mM HCO3−). According to previous research,39 HCO3− would react with PMS to generate HCO4−. However, HCO4− was erratic, and it would resolve into HCO3− and H2O2, which could create an unfavorable condition for BPS degradation (eqn (11) and (12)). Besides, the addition of HCO3− would strongly influence solution pH, the solution pH was decreased to 10.16, 9.64 and 9.50 as the dosages of HCO3− increased to 1 mM, 5 mM and 10 mM, which would slow the degradation of BPS. In addition, it reported that HCO3− could quench ROS to produce less reactive carbonate ones (HCO3˙ and CO3˙−) according to Fig. 5(c) and (d).40 the degradation of BPS was not significantly affected with the addition of SO42− and NO3−, which implied that both of these two anions don't react with 1O2 and O2˙−.41
|
HSO5− + HCO3− → HCO4− + HSO4−
| (11) |
|
HCO4− + H2O → HCO3− + H2O2
| (12) |
Fig. 5(e) showed the influence of HA on the degradation of BPS. In this study, the degradation of BPS in the presence of 5 mg L−1, 20 mg L−1 and 50 mg L−1 HA was 98.62%, 96.84% and 96.32%, respectively. As the concentration of HA increased, the degree of inhibition was stronger. Similar trends also appeared in the UV/PMS removal of BPA by UV/PMS system.42 HA containing plenty of carboxyl and hydroxyl groups can inhibit the degradation process by quenching radicals and competing with target contaminants for ROS.43 Nevertheless, the removal efficiency of BPS was higher than 96% in the presence of 50 mg L−1 HA, revealing that the Ca(OH)2/PMS system was a promising process for the decontamination of BPS in water even contained a high concentration of HA.
3.7 Pathway of BPS degradation
In the Ca(OH)2/PMS system, BPS was completely degraded and about 60% PMS was consumed within 240 min. Interestingly, PMS was no longer consumed (Fig. 6(a)) after 80 min. It may be due to the pH decreasing gradually as the reaction progress, which was not conducive to the decomposition of PMS. An important indicator of organic removal is the degree of mineralization, which can be determined by the TOC removal. However, the TOC removal percentage of the Ca(OH)2/PMS system was only 17.52% within 240 min (Fig. 6(b)), which indicated incomplete mineralization of BPS and presence of intermediate products. A total of 4 degradation products of BPS were identified by LC-TOF-MS. According to these intermediates, 3 possible transformation pathways were proposed as shown in Fig. 7. 1O2 and O2˙− initially attacked the phenol moiety of BPS and acquired one electron, and then transformed into some phenoxy radical (R1) and other resonance forms (R2 and R3) due to the delocalization of the unpaired electron.2 R1 and R2 could react with each other and generate resultant dimers (P1 and P2) by C–C and C–O coupling.2 The radicals (R3) with high electron density at the para position would generate R4 and R5 via β-scission reaction. Then, 4-hydroxy-benzenesulfinic acid (P3) would be formed from the hydroxylation of R4.44 In the other way, the resonance form (R2) was hydroxylated directly to formed P4.
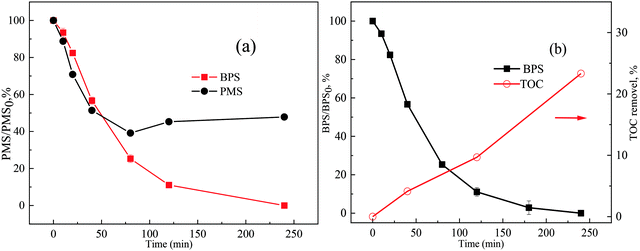 |
| Fig. 6 Removal of PMS (a), BPS and TOC (b) in the Ca(OH)2/PMS system. | |
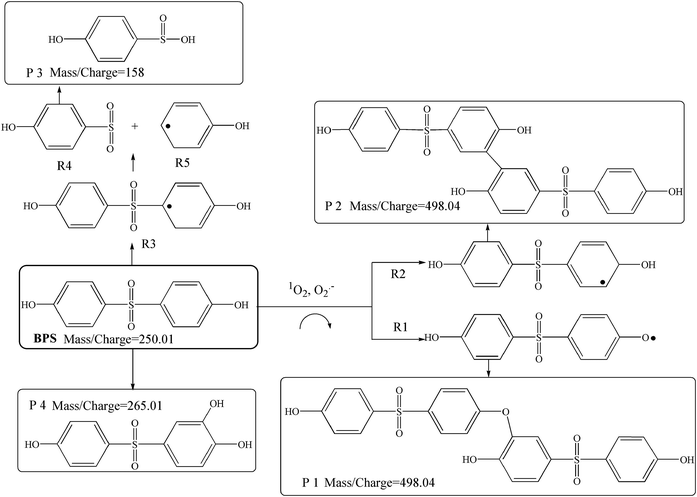 |
| Fig. 7 Possible degradation pathways of BPS in the Ca(OH)2/PMS system. | |
3.8 Influence of different water matrices
The influence of different water matrices including ultrapure water, Poyang lake water, Yaohu lake water and domestic wastewater on BPS degradation in the Ca(OH)2/PMS system were investigated. As illustrated in Fig. 8(a), 100% BPS removal was achieved in the ultrapure water, but it was slightly inhibited in Poyang Lake water (81.48%), Yaohu Lake water (77.94%) and domestic wastewater (75.86%). Accordingly, the kobs values of BPS removal were decreased to 0.00730 min−1, 0.00660 min−1 and 0.00630 min−1, respectively. Based on the detailed physicochemical properties of real water samples (Table S1†) and the results about co-existing anions and HA from Section 3.7. This phenomenon could attribute to the fact that real water matrices were rich in NOM, which may act as ROS scavenger, further affect the degradation of BPS.
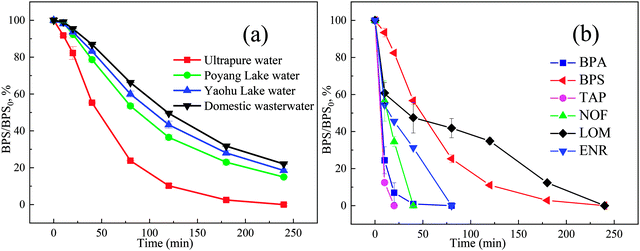 |
| Fig. 8 The reaction in different water matrices (a), the degradation of different pollutants in the Ca(OH)2/PMS system (b), [Ca(OH)2]0 = 1 mM, [PMS]0 = 1.5 mM, [BPS]0 = 20 μM. | |
3.9 Wide applicability of the Ca(OH)2/PMS system
Apart from BPS, the performances of the Ca(OH)2/PMS system were also evaluated by degrading several typical organic pollutants, including antibiotics (TAP, NOF, LOM, and ENR), and endocrine disruptor (BPA) at 20 μM (Fig. 8(b)). Just like the removal of BPS in the Ca(OH)2/PMS system, the whole of TAP, NOF, BPA, ENR and LOM could be removed within 20 min, 40 min, 80 min, 80 min and 240 min, respectively. 1O2 could oxidize electron-rich compounds (electron-rich phenols, olefins, and sulfides),45 therefore, the Ca(OH)2/PMS system can be widely used to degrade a variety of organic pollutants. However, the specific degradation mechanism needs further exploration.
4 Conclusions
In the present study, the enhanced effect of the Ca(OH)2 on BPS removal by the Ca(OH)2/PMS system was observed. 100% BPS were efficiently removed in a certain condition ([Ca(OH)2]0 = 1 mM, [PMS]0 = 1.5 mM). The degradation of BPS maintained on a high level in a wide range of pH values and optimized alkali activated PMS making the final pH close to neutral. BPS degradation was significantly accelerated with the temperature increased. The adding mode of PMS implied that the higher initial PMS concentration led to the higher BPS degradation. The SO4−, NO3− had no significant influence on BPS degradation, however, the HCO3− and HA inhibited the BPS oxidation. Cl− had a dual effect on BPS degradation, a low concentration of Cl− will inhibit the degradation of BPS, but a higher concentration of Cl− would promote the degradation of BPS due to the generation of reactive chlorine. Based on the quenching experiments, it was indicated that both O2˙− and 1O2 have participated in the Ca(OH)2/PMS system, and 1O2 played a major role on BPS degradation. Only 17.52% of TOC were removed, which indicated the incomplete mineralization of BPS and the presence of intermediate products. A total of 4 degradation intermediates of BPS were identified and possible transformation pathways were proposed. BPS degradation efficiency in the real waters was lower than that in ultrapure water due to the presence of matrixes. Furthermore, other pollutants including (TAP, NOF, LOM and ENR) exhibited an efficient degradation in the Ca(OH)2/PMS system.
Author contributions
Leliang Wu: writing, methodology, conceptualization, validation. Yiting Lin: methodology, validation. Yimin Zhang: methodology. Peng Wang: supervision. Mingjun Ding: supervision. Minghua Nie: ideas, supervision, conceptualization, investigation, writing – review & editing. Caixia Yan: investigation, writing – review & editing, supervision.
Conflicts of interest
The authors declare that they have no known competing financial interests or personal relationships that could have appeared to influence the work reported in this paper.
Acknowledgements
This work was financially supported by the National Natural Science Foundation of China (No. 42067034, 42067058), the Jiangxi Provincial Natural Science Foundation (20202BAB203015, 20202BAB203014), and the Open Fund of Key Laboratory of Eco-geochemistry, Ministry of Natural Resources (ZSDHJJ202004).
References
- T. B. Nguyen and R. Doong, Heterostructure ZnFe2O4/TiO2 nanocomposites with a highly recyclable visible-light-response for bisphenol A degradation, RSC Adv., 2017, 7, 50006–50016 RSC.
- T. Yang, L. Wang, Y. Liu, Z. Huang, H. He, X. Wang, J. Jiang, D. Gao and J. Ma, Comparative study on ferrate oxidation of BPS and BPAF: kinetics, reaction mechanism, and the improvement on their biodegradability, Water Res., 2019, 148, 115–125 CrossRef CAS PubMed.
- L. Li, D. Xu and Z. Pei, Kinetics and thermodynamics studies for bisphenol S adsorption on reduced graphene oxide, RSC Adv., 2016, 6, 60145–60151 RSC.
- S. Kitamura, T. Suzuki, S. Sanoh, R. Kohta, N. Jinno, K. Sugihara, S. Yoshihara, N. Fujimoto, H. Watanabe and S. Ohta, Comparative study of the endocrine-disrupting activity of bisphenol A and 19 related compounds, Toxicol. Sci., 2005, 84, 249–258 CrossRef CAS PubMed.
- S. B. Hammouda, F. Zhao, Z. Safaei, D. L. Ramasamy, B. Doshi and M. Sillanpaa, Sulfate radical-mediated degradation and mineralization of bisphenol F in neutral medium by the novel magnetic Sr2CoFeO6 double perovskite oxide catalyzed peroxymonosulfate: influence of co-existing chemicals and UV irradiation, Appl. Catal., B, 2018, 233, 99–111 CrossRef.
- Z. Fang, Y. Gao, X. Wu, X. Xu, A. K. Sarmah, N. Bolan, B. Gao, S. M. Shaheen, J. Rinklebe, Y. S. Ok, S. Xu and H. Wang, A critical review on remediation of bisphenol S (BPS) contaminated water: Efficacy and mechanisms, Environ. Sci. Technol., 2020, 50, 476–522 CAS.
- F. Ghanbari and M. Moradi, Application of peroxymonosulfate and its activation methods for degradation of environmental organic pollutants, Chem. Eng. J., 2017, 310, 41–62 CrossRef CAS.
- W. Tang, Y. Zhang, H. Guo and Y. Liu, Heterogeneous activation of peroxymonosulfate for bisphenol AF degradation with BiOI0.5Cl0.5, RSC Adv., 2019, 9, 14060 RSC.
- Y. Li, Y. Shi, D. Huang, Y. Wu and W. Dong, Enhanced activation of persulfate by Fe (III) and catechin without light: Reaction kinetics, parameters and mechanism, J. Hazard. Mater., 2021, 413, 125420 CrossRef CAS PubMed.
- M. Nie, W. Zhang, C. Yan, W. Xu, L. Wu, Y. Ye, Y. Hu and W. Dong, Enhanced removal of organic contaminants in water by the combination of peroxymonosulfate and carbonate, Sci. Total Environ., 2019, 647, 734–743 CrossRef CAS PubMed.
- C. Qi, X. Liu, J. Ma, C. Lin, X. Li and H. Zhang, Activation of peroxymonosulfate by base: Implications for the degradation of organic pollutants, Chemosphere, 2016, 151, 280–288 CrossRef CAS PubMed.
- J. Zhang, X. Shao, C. Shi and S. Yang, Decolorization of acid orange 7 with peroxymonosulfate oxidation catalyzed by granular activated carbon, Chem. Eng. J., 2013, 232, 259–265 CrossRef CAS.
- X. Zhou, A. Jawad, M. Luo, C. Luo, T. Zhang, H. Wang, J. Wang, S. Wang, Z. Chen and Z. Chen, Regulating activation pathway of Cu/persulfate through the incorporation of unreducible metal oxides: Pivotal role of surface oxygen vacancies, Appl. Catal., B, 2021, 286, 119914 CrossRef CAS.
- H. Lee, H. J. Lee, J. Jeong, J. Lee, N. B. Park and C. Lee, Activation of persulfates by carbon nanotubes: oxidation of organic compounds by nonradical mechanism, Chem. Eng. J., 2015, 266, 28–33 CrossRef CAS.
- Y. Zhou, Y. Gao, J. Jiang, Y. Shen, S. Pang, Z. Wang, J. Duan, Q. Guo, C. Guan and J. Ma, Transformation of tetracycline antibiotics during water treatment with non-activated peroxymonosulfate, Chem. Eng. J., 2020, 379, 122378 CrossRef CAS.
- Y. Guan, J. Ma, Y. Ren, Y. Liu, J. Xiao, L. Lin and C. Zhang, Efficient degradation of atrazine by magnetic porous copper ferrite catalyzed peroxymonosulfate oxidation via the formation of hydroxyl and sulfate radicals, Water Res., 2013, 47, 5431–5438 CrossRef CAS PubMed.
- A. D. Bokare, R. C. Chikate, C. V. Rode and K. M. Paknikar, Effect of surface chemistry of Fe-Ni nanoparticles on mechanistic pathways of azo dye degradation, Environ. Sci. Technol., 2007, 41, 7437–7443 CrossRef CAS PubMed.
- W. Wu, S. Zhu, X. Huang, W. Wei and B. Ni, Mechanisms of persulfate activation on biochar derived from two different sludges: dominance of their intrinsic compositions, J. Hazard. Mater., 2021, 408, 124454 CrossRef CAS PubMed.
- Y. Zhou, J. Jiang, Y. Gao, J. Ma, S. Pang, J. Li, X. Lu and L. Yuan, Activation of peroxymonosulfate by benzoquinone: a novel nonradical oxidation process, Environ. Sci. Technol., 2015, 49, 12941–12950 CrossRef CAS PubMed.
- Y. Peng, H. Tang, B. Yao, X. Gao, X. Yang and Y. Zhou, Activation of peroxymonosulfate (PMS) by spinel ferrite and their composites in degradation of organic pollutants: A Review, Chem. Eng. J., 2021, 414, 128800 CrossRef CAS.
- X. Lou, C. Fang, Z. Geng, Y. Jin, D. Xiao, Z. Wang, J. Liu and Y. Guo, Significantly enhanced base activation of peroxymonosulfate by polyphosphates: Kinetics and mechanism, Chemosphere, 2017, 173, 529–534 CrossRef CAS PubMed.
- E. Baralla, V. Pasciu, M. V. Varoni, M. Nieddu, R. Demuro and M. P. Demontis, Bisphenols' occurrence in bivalves as sentinel of environmental contamination, Sci. Total Environ., 2021, 785, 147263 CrossRef CAS PubMed.
- J. Deng, S. Feng, K. Zhang, J. Li, H. Wang, T. Zhang and X. Ma, Heterogeneous activation of peroxymonosulfate using ordered mesoporous Co3O4 for the degradation of chloramphenicol at neutral pH, Chem. Eng. J., 2017, 308, 505–515 CrossRef CAS.
- J. Tang, L. Tang, H. Feng, G. Zeng, H. Dong, C. Zhang, B. Huang, Y. Deng, J. Wang and Y. Zhou, pH-dependent degradation of p-nitrophenol by sulfidated nanoscale zerovalent iron under aerobic or anoxic conditions, J. Hazard. Mater., 2016, 320, 581–590 CrossRef CAS PubMed.
- J. Yu, J. Zhang, T. Zeng, H. Wang, Y. Sun, L. Chen, S. Song and H. Shi, Stable incorporation of MnOx quantum dots into N-doped hollow carbon: a synergistic peroxymonosulfate activator for enhanced removal of bisphenol A, Sep. Purif. Technol., 2019, 213, 264–275 CrossRef CAS.
- F. Ghanbari, M. Ahmadi and F. Gohari, Heterogeneous activation of peroxymonosulfate via nanocomposite CeO2-Fe3O4 for organic pollutants removal: the effect of UV and US irradiation and application for real wastewater, Sep. Purif. Technol., 2019, 228, 115732 CrossRef CAS.
- S. H. Do, J. H. Jo, Y. H. Jo, H. K. Lee and S. H. Kong, Application of a peroxymonosulfate/cobalt (PMS/Co (II)) system to treat diesel-contaminated soil, Chemosphere, 2009, 77, 1127–1131 CrossRef CAS PubMed.
- M. Nie, Y. Yang, Z. Zhang, C. Yan, X. Wang, H. Li and W. Dong, Degradation of chloramphenicol by thermally activated persulfate in aqueous solution, Chem. Eng. J., 2014, 246, 373–382 CrossRef CAS.
- S. Wang, J. Tian, Q. Wang, F. Xiao, S. Gao, W. Shi and F. Cui, Development of CuO coated ceramic hollow fiber membrane for peroxymonosulfate activation: a highly efficient singlet oxygen-dominated oxidation process for bisphenol a degradation, Appl. Catal., B, 2019, 256, 117783 CrossRef CAS.
- J. A. Khan, X. He, H. M. Khan, N. S. Shah and D. D. Dionysiou, Oxidative degradation of atrazine in aqueous solution by UV/H2O2/Fe2+, UV/Fe2+ and UV/Fe2+ processes: a comparative study, Chem. Eng. J., 2013, 218, 376–383 CrossRef CAS.
- J. Deng, Y. Shao, N. Gao, Y. Deng, S. Zhou and X. Hu, Thermally activated persulfate (TAP) oxidation of antiepileptic drug carbamazepine in water, Chem. Eng. J., 2013, 228, 765–771 CrossRef CAS.
- L. Zhang, J. Chen, W. Li, Z. Wang and T. Huang, Kinetics for degradation of Orange G with peroxymonosulfate activated by carbon nanotubes, Environ. Sci. Technol., 2016, 37, 2601–2609 Search PubMed.
- J. Yan, J. Li, J. Peng, H. Zhang and B. Lai, Efficient degradation of sulfamethoxazole by the CuO@Al2O3 (EPC) coupled PMS system: optimization, degradation pathways and toxicity evaluation, Chem. Eng. J., 2019, 359, 1097–1110 CrossRef CAS.
- G. Peng, W. You, W. Zhou, G. Zhou, C. Qi and Y. Hu, Activation of peroxymonosulfate by phosphite: kinetics and mechanism for the removal of organic pollutants, Chemosphere, 2021, 266, 129016 CrossRef CAS PubMed.
- G. Lente, J. Kalmar, Z. Baranyai, A. Kun, I. Kek, D. Bajusz, M. Takacs, L. Veres and I. Fabian, One-versus two-electron oxidation with peroxomonosulfate ion: reactions with iron (II), vanadium (IV), halide ions, and photoreaction with cerium (III), Inorg. Chem., 2009, 48, 1763–1773 CrossRef CAS PubMed.
- G. Peng, T. Li, B. Ai, S. Yang, J. Fu, Q. He, G. Yu and S. Deng, Highly efficient removal of enrofloxacin by magnetic montmorillonite via adsorption and persulfate oxidation, Chem. Eng. J., 2019, 360, 1119–1127 CrossRef CAS.
- L. Xu, R. Yuan, Y. Guo, D. Xiao, Y. Cao, Z. Wang and J. Liu, Sulfate radical-induced degradation of 2,4,6-trichlorophenol: a de novo formation of chlorinated compounds, Chem. Eng. J., 2013, 217, 169–173 CrossRef CAS.
- R. Yuan, S. N. Ramjaun, Z. Wang and J. Liu, Effects of chloride ion on degradation of acid orange 7 by sulfate radical-based advanced oxidation process: implications for formation of chlorinated aromatic compounds, J. Hazard. Mater., 2011, 196, 173–179 CrossRef CAS PubMed.
- Z. Huang, Y. Yao, J. Lu, C. Chen, W. Lu, S. Huang and W. Chen, The consortium of heterogeneous cobalt phthalocyanine catalyst and bicarbonate ion as a novel platform for contaminants elimination based on peroxymonosulfate activation, J. Hazard. Mater., 2016, 301, 214–221 CrossRef CAS PubMed.
- Y. Peng, H. Shi, Z. Wang, Y. Fu and Y. Liu, Kinetics and reaction mechanism of photochemical degradation of diclofenac by UV-activated peroxymonosulfate, RSC Adv., 2021, 11, 6804 RSC.
- Z. Fang, J. Zhao, Y. Li, Y. Wang, T. Qiu, Y. Wu, W. Dong and G. Mailhot, Improving Fenton-like system with catechin, an environmental-friendly polyphenol: effects and mechanism, Chem. Eng. J., 2021, 426, 127946 CrossRef CAS.
- S. Tang, J. Tang, D. Yuan, Z. Wang, Y. Zhang and Y. Rao, Elimination of humic acid in water: comparison of UV/PDS and UV/PMS, RSC Adv., 2020, 10, 17627–17634 RSC.
- L. Chen, D. Ding, C. Liu, H. Cai, Y. Qu, S. Yang, Y. Gao and T. Cai, Degradation of norfloxacin by CoFe2O4-GO composite coupled with peroxymonosulfate: a comparative study and mechanistic
consideration, Chem. Eng. J., 2018, 334, 273–284 CrossRef CAS.
- J. Peng, H. Zhou, W. Liu, Z. Ao, H. Ji, Y. Liu, S. Su, G. Yao and B. Lai, Insights into heterogeneous catalytic activation of peroxymonosulfate by natural chalcopyrite: pH-dependent radical generation, degradation pathway and mechanism, Chem. Eng. J., 2020, 397, 125387 CrossRef CAS.
- H. Kim, W. Kim, Y. Mackeyev, G. Lee, J. Kim, T. Tachikawa, S. Hong, S. Lee, J. Kim, L. Wilson, T. Majima, P. Alvarez, W. Choi and J. Lee, Selective oxidative degradation of organic pollutants by singlet oxygen-mediated photosensitization: Tin porphyrin versus C60 amino fullerene systems, Environ. Sci. Technol., 2012, 46, 9606–9613 CrossRef CAS PubMed.
Footnote |
† Electronic supplementary information (ESI) available. See DOI: 10.1039/d1ra05286a |
|
This journal is © The Royal Society of Chemistry 2021 |
Click here to see how this site uses Cookies. View our privacy policy here.