DOI:
10.1039/D1RA04834A
(Paper)
RSC Adv., 2021,
11, 30635-30645
Retracted Article: Rapid and selective detection of aluminum ion using 1,2,3-triazole-4,5-dicarboxylic acid-functionalized gold nanoparticle-based colorimetric sensor†
Received
22nd June 2021
, Accepted 5th September 2021
First published on 15th September 2021
Abstract
A highly selective, sensitive, rapid, low-cost, simple and visual colorimetric system for Al3+ ion detection was developed based on gold nanoparticles (AuNPs) modified with 1,2,3-triazole-4,5-dicarboxylic acid (TADA). The modified gold nanoparticles (TADA–AuNPs) were first prepared by sodium citrate (Na3Ct) reduction of chloroauric acid (HAuCl4) and then capped with a TADA ligand. Five TADA–AuNPs sensors were constructed with sodium citrate (Na3Ct)/chloroauric acid (HAuCl4) under different molar ratios. Results showed that the molar ratio of Na3Ct/HAuCl4, TADA–AuNPs concentration, pH range and detection time had obvious influences on the performance of this colorimetric method. The optimal detection conditions for Al3+ ions were as follows: Na3Ct/HAuCl4 molar ratio of 6.4
:
1, 0.1 mM of TADA–AuNPs concentration, 4–10 pH range and 90 s of detection time. Under the optimal conditions and using diphenyl carbazone (DPC) as a Cr3+ masking agent, this colorimetric sensor exhibited outstanding time efficiency, selectivity and sensitivity for Al3+ detection. In particular, the detection limits of this sensor obtained via UV-vis and the naked eye were 15 nM and 1.5 μM, respectively, which were much lower than the current limit (3.7 μM) for drinking water in WHO regulation and better than the previous reports. Moreover, this colorimetric sensing system could be used to for on-site, trace level and real-time rapid detection of Al3+ in real water samples.
1. Introduction
Aluminum (Al) is one of the richest metals in nature, and it is widely used in modern industry and everyday life, such as in packaging foils, containers, cooking utensils, and food additives. However, aluminum products will release aluminum ion (Al3+) slowly, contaminating food, drinking water or soil. Moreover, Al3+ can also accumulate in the human body through the food chain. An increasing level of Al3+ in the human body can trigger a series of diseases, including senile dementia and Parkinson's disease. Therefore, Al3+ has been restricted as a toxic metal by the World Health Organization (WHO) with a maximum consumption limit of 7 mg per kilogram body weight weekly, or 3.7 μM (0.1 mg L−1) and 7.4 μM (0.2 mg L−1) in drinking water for large and small water treatment facilities, respectively.1–4
Several traditional detection methods, such as atomic absorption spectrometry (AAS),5 inductively coupled plasma optical emission spectroscopy (ICP-OES)6 and inductively coupled plasma mass spectrometry (ICP-MS),7 have been employed to monitor trace levels of Al3+ in food or in the environment. Although these methods can provide high sensitivity and accurate results, the detection process is time-consuming and generally requires costly sophisticated instrumentation and skilled professionals, which limit their applications, especially for on-site and real-time detection. Thus, it is urgent to develop an inexpensive, highly efficient, instrument-free and easily operated method for trace level Al3+ detection.
Colorimetric sensors based on gold nanoparticles (AuNPs) have attracted widespread attention because of their fast and visual output, as well as no need for expensive instruments. Taking advantages of unique surface plasmon resonance (SPR) absorption, easy surface functionalization and variable solution colors, AuNPs have been used for colorimetric sensing in food security, environmental monitoring, and health care.8–11 Potential applications include the determination of metal ions,12–18 pesticide residues,19,20 bisphenol A (BPA),21,22 phosphate,23 azodicarbonamide (ADA),24 antibiotics,25–27 adenosine and nucleic acids,28–30 dopamine,31 bacteria,32,33 and virus.34,35
In recent years, rapid detection of Al3+ using AuNPs as a colorimetric sensor have been reported, in which the AuNPs' surface were functionalized by different Al3+ recognition ligands, such as diaminodiphenyl sulfone,36 ascorbic acid,37 11-mercaptoundecanoic acid,38 5-mercaptomethyltetrazole,39 poly(acrylic acid),40 triazole ether,41 catechol,42 and xylenol orange.43 Their detection limits via UV-vis and the naked eye, detection time, and interference ions are summarized in Table 1. It is clear that different ligands have great influence on the sensitivity and selectivity of the sensors. However, most of these sensors show low sensitivity with naked eye detection, which is higher than the current limit (3.7 μM) in drinking water,36–41 need long detection time38–41 or suffer from interferences.36 Some efforts have been made to decrease the naked eye detection limits to 3.5 μM,42 and 2.8 μM,43 which can almost meet the limit requirement in drinking water. 1,2,3-Triazole-4,5-dicarboxylic acid (TADA), with both triazole and carboxyl groups in the structure, was found to have strong binding force to AuNPs.44 In addition, abundant lone pair electrons in the TADA may possess it of superior capability in coordinating with metal cations.
Table 1 Comparison of ligand-functionalized gold nanoparticles-based colorimetric sensors for Al3+ detection
Ligand |
Detection limit (μM) |
Detection time (s) |
Interference ions |
Eliminating interference method |
Ref. |
UV-vis |
Naked eye |
Diaminodiphenyl sulfone |
0.62 |
— |
— |
Fe3+, Cr3+ |
— |
36 |
Ascorbic acid |
0.46 |
— |
120 |
Ca2+ |
Using anion exchange resin |
37 |
11-Mercap-to-undecanoic acid |
0.57 |
20 |
1800 |
Ca2+, Mg2+, Pb2+ |
Using EDTA masking agent |
38 |
5-Mercap-to-methyltetrazole |
0.53 |
10 |
900 |
— |
— |
39 |
Poly(acrylic acid) |
2 |
8 |
900 |
— |
— |
40 |
Triazole ether |
0.018 |
5 |
600 |
— |
— |
41 |
Catechol |
0.81 |
3.5 |
60 |
— |
— |
42 |
Xylenol orange |
0.44 |
2.8 |
— |
— |
— |
43 |
1,2,3-Triazole-4,5-dicarboxylic acid |
0.015 |
1.5 |
90 |
Cr3+ |
Using DPC masking agent |
This work |
We herein for the first time constructed a colorimetric sensor based on TADA-modified AuNPs for highly efficient detection of Al3+ ion. The TADA–AuNPs were easily synthesized from the pre-synthesized citrate-covered AuNPs (citrate–AuNPs), as the triazole group of TADA more readily binds to Au, compared to the carboxyl group.44 The principle of this colorimetric sensing method is that TADA on the surface of AuNPs can specifically recognize Al3+, resulting in an aggregation-induced color change from dark red to violet or blue. This change was confirmed by Fourier transform infrared (FTIR), Raman spectroscopy, UV-visible spectrophotometer (UV-vis), transmission electron microscopy (TEM), and dynamic light scattering (DLS). Several TADA–AuNPs sensors with different particle sizes were prepared under different molar ratios of sodium citrate (Na3Ct)/chloroauric acid (HAuCl4). The selectivity and sensitivity of the method were evaluated using optimized detection parameters, including Na3Ct/HAuCl4 molar ratio, TADA–AuNPs concentration, pH range, and detection time. The detection time is 90 s and the detection limits via UV-vis and the naked eye were determined to be 15 nM and 1.5 μM, respectively, better than the previous reports.36–43 We propose that this TADA–AuNPs colorimetric method might be suitable for real-time rapid detection of Al3+ ion in water, milk, soil, and other foods.
2. Materials and methods
2.1. Chemicals
Chloroauric acid (HAuCl4), sodium citrate (Na3Ct), and 1,2,3-triazole-4,5-dicarboxylic acid (TADA) were analytical grade and purchased from Lab Network (Shanghai, China). Sodium hydroxide (NaOH), phosphate buffered saline (PBS), hydrochloric acid (HCl), AlCl3, MnCl2, MgCl2, FeCl3, K2Cr2O7, CrCl3, InCl3, CoCl2, CdCl2, CaCl2, BaCl2, CuCl2, NiCl2, PbCl2, Hg(NO3)2, Fe(NH4)2(SO4)2, NaCl, KCl, Na2SO4, and Na3PO4 were analytical grade and purchased from Aladdin (Shanghai, China). GaCl3 was analytical grade and purchased from Bidepharm (Shanghai, China). Al3+ standard solution was purchased from Macklin (Shanghai, China). Diphenyl carbazone (DPC), analytical grade, was used as a Cr3+ masking agent and purchased from Macklin (Shanghai, China). Ultrapure water, 18.2 MΩ cm at 25 °C, was used for preparing solutions.
2.2. Synthesis of TADA-functionalized AuNPs (TADA–AuNPs) sensor
The TADA–AuNPs were prepared using a previously reported method, with some improvement.18 To synthesize citrate-terminated AuNPs (citrate–AuNPs), 100 mL of 0.25 mM chloroauric acid (HAuCl4) aqueous solution were boiled, followed by the rapid addition of 4 mL of 40 mM sodium citrate (Na3Ct) solution. The Na3Ct/HAuCl4 molar ratio was 6.4
:
1. The solution was kept boiling for 30 min with stirring and then cooled to room temperature. The solution color changed from light yellow to dark red, indicating that citrate–AuNPs had formed in the solution. To modify citrate–AuNPs with TADA, 100 mL synthesized citrate–AuNPs solution was transferred to a 500 mL round bottom flask and then mixed with 145 mL of 0.25 mM TADA solution and 5 mL PBS solution. The pH was adjusted to 7.41 with 0.5 mM NaOH solution. The mixed solution was heated to 60 °C, incubated for 1 h with stirring, and then cooled to room temperature. The mixed solution was centrifuged for 25 min at 15
000 rpm to remove the excess NaOH, TADA, and sodium citrate. The TADA–AuNPs precipitate was resuspended in 10 mL of ultrapure water to obtain the stock solution. A series of TADA–AuNPs solutions with concentrations ranged from 0.025 mM to 0.55 mM were prepared by diluting the stock solution with appropriate amount of ultrapure water. Five TADA–AuNPs solutions with different particle size were obtain by controlling the Na3Ct/HAuCl4 molar ratio as follows: 0.8
:
1, 1.6
:
1, 3.2
:
1, 6.4
:
1, and 12.8
:
1, respectively. The synthesis process is shown in Scheme 1.
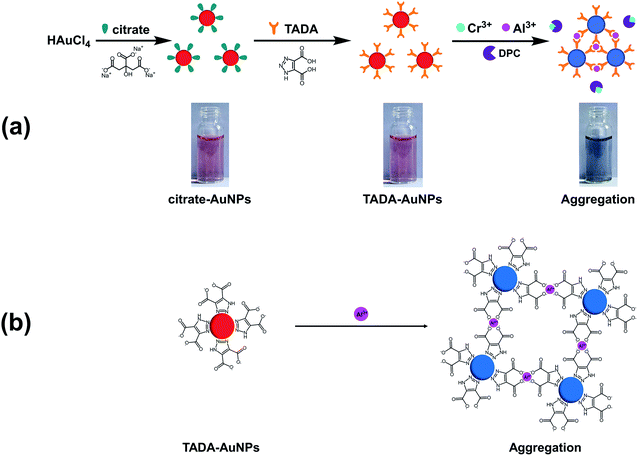 |
| Scheme 1 Schematic of the design strategy for the Al3+ colorimetric sensor. | |
2.3. Characterization
UV-vis absorption spectra were measured via a Shimadzu UV-2700 UV-vis spectrophotometer. The transmission electron microscopy (TEM) images were obtained on a ThermoFisher Tecnai G2 Spirit TEM microscope. The Fourier transform infrared (FTIR) spectra were recorded on a Shimadzu IRAffinity-1S spectrophotometer. The dynamic light scattering (DLS) images were generated on a Malvern ZEN3700 DLS and Zeta Sizer. The Raman spectra were measured by a Renishaw's inVia Raman spectrometer.
3. Results and discussion
3.1. Construction and characterization of Al3+ colorimetric sensor
A main advantage of TADA–AuNPs for target analytes (Al3+) is their excellent selective colorimetric response, through which the ion content can be visualized by the naked eye or detected by UV-vis spectrophotometer.
The design strategy for the Al3+colorimetric sensor is illustrated in Scheme 1. First, stable dark red citrate–AuNPs solution was obtained by reducing HAuCl4 with Na3Ct. Then, citrate–AuNPs were functionalized by TADA, and well-dispersed dark red AuNPs capped with TADA (TADA–AuNPs) were obtained. The solution color changes from dark red to violet or blue due to the aggregation of TADA–AuNPs induced by Al3+ in the presence of a masking agent (DPC) to eliminate the interference of Cr3+ (Scheme 1a). The triazole groups on TADA–AuNPs function as metal ion binding ligands. Since Al3+ was bound between the triazoles which from TADA, the TADA–AuNPs aggregated (Scheme 1b).
Fig. 1 gives the FTIR and Raman spectra of citrate–AuNPs and TADA–AuNPs. As shown in Fig. 1a, with the exception of the citrate–AuNPs characteristic peaks at 1655 cm−1 (was, C
O) and 3300–3600 cm−1 (O–H), there exhibited two new characteristic peaks at 1570 cm−1 (N
N) and 3480 cm−1 (N–H) in the TADA–AuNPs spectra, which belong to the triazole groups in TADA. Thus, this result indicates that TADA ligand was attached to the citrate–AuNPs surface successfully.45 To further confirm the binding mode of the ligand TADA on the surface of citrate–AuNPs, Raman spectra of citrate–AuNPs and TADA–AuNPs are measured. As shown in Fig. 1b, TADA–AuNPs has an obvious new characteristic peak at 560 cm−1 compared with citrate–AuNPs, which belongs to Au–N bond.46 Therefore, combining FTIR and Raman spectroscopy, it clearly demonstrates that the ligand TADA was modified to the surface of citrate–AuNPs through its triazole group's N atom.
 |
| Fig. 1 (a) Fourier infrared (FTIR) and (b) Raman spectra of citrate–AuNPs and TADA–AuNPs. | |
In order to explore the response of TADA–AuNPs to Al3+, the photographs and UV-vis absorption spectra of citrate–AuNPs, TADA–AuNPs and their corresponding solutions containing Al3+ are obtained. As shown in Fig. 2, the appearance of dark red color and the shift in the peak at 520 nm to 525 nm in the UV-vis absorption spectra (Fig. 2b), compared to citrate–AuNPs (Fig. 2a), indicate the formation of AuNPs and surface functionalization with TADA. When 2 μM of Al3+ was added to the TADA–AuNPs solution, the solution color changed from red to blue, and a new absorption peak at 625 nm appeared along with weaker absorption found at 525 nm in the UV-vis spectrum (Fig. 2c). In comparison, the UV-vis spectrum and the solution color of citrate–AuNPs mixed with 5 μM Al3+ were nearly the same with those of citrate–AuNPs (Fig. 2a and d). These results illustrate that the TADA-modification possesses the AuNPs with specific Al3+ recognition, resulting in the color change of the solution from red to blue.
 |
| Fig. 2 UV-vis spectra and photographs (inset) of (a) citrate–AuNPs (0.05 mM), (b) TADA–AuNPs (0.05 mM), (c) TADA–AuNPs (0.05 mM) mixed with 2 μM Al3+ and (d) citrate–AuNPs (0.05 mM) mixed with 5 μM Al3+. | |
This result can be further confirmed by TEM and DLS measurements. Fig. 3a and b show that both citrate–AuNPs and TADA–AuNPs are well-dispersed, spherical nanoparticles with a hydrodynamic average particle size of about 30 nm (Fig. 3a′ and b′). Upon the addition of 2 μM Al3+ to TADA–AuNPs, obvious particle aggregation can be observed in TEM image and the hydrodynamic average particle size increases to about 192 nm (Fig. 3c′). These results may be due to the large number of carboxyl groups present on the surface of TADA–AuNPs, causing its zeta potential to be −43.9 mV in pH = 6.3 (see Fig. S1 in ESI†) and making it exhibit a specific and strong targeting force to Al3+.47 At the same time, “interparticle cross-linking mechanism” will occur between Al3+ and TADA–AuNPs, resulting in induced aggregation of TADA–AuNPs (Scheme 1b).37,47 Conversely, for citrate–AuNPs, the addition of Al3+ had no effects on the shape or size of AuNPs, indicating no interaction between Al3+ ions and citrate–AuNPs.
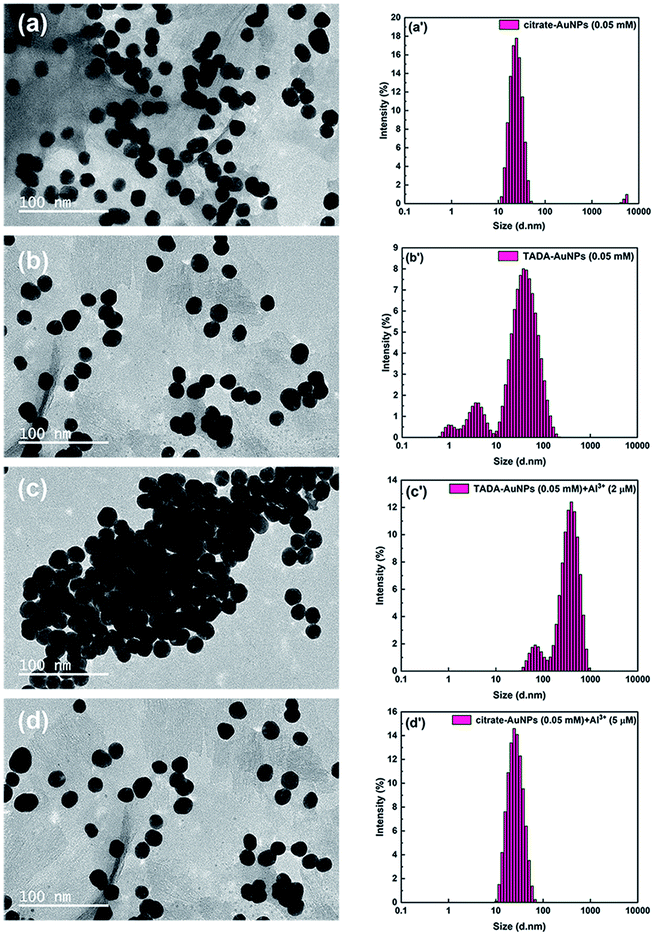 |
| Fig. 3 TEM and DLS images of (a and a′) citrate–AuNPs (0.05 mM), (b and b′) TADA–AuNPs (0.05 mM), (c and c′) TADA–AuNPs (0.05 mM) mixed with 2 μM Al3+ and (d and d′) citrate–AuNPs (0.05 mM) mixed with 5 μM Al3+. | |
It is well known that the color change of AuNPs is closely related to the factors such as particle size, shape, aggregation state, shape, capping agent, and solvent refractive index.48 Thus, according to Fig. 2 and 3, it is likely that Al3+ induced the aggregation of TADA–AuNPs, causing a significant change in particle size, resulting in the solution color change from red to blue.
To estimate the optimum particles size of TADA–AuNPs, five different molar ratios of Na3Ct/HAuCl4 (0.8
:
1, 1.6
:
1, 3.2
:
1, 6.4
:
1, and 12.8
:
1) were introduced for the preparation of TADA–AuNPs, and the HAuCl4 concentration was 0.25 mM. Particle morphologies and solution colors were obtained from TEM and digital camera (Fig. 4). The average sizes of TADA–AuNPs decreased from 56 nm to 14 nm when the Na3Ct/HAuCl4 molar ratio ranged from 0.8
:
1 to 3.2
:
1 (Fig. 4 a–c). Then, the particle size became stable when further increasing the Na3Ct proportion (Fig. 4 d and e), which was similar to a previous report.49 Meanwhile, the solution colors of the obtained TADA–AuNPs changed from purple to red along with the Na3Ct ratio increase (Fig. 4f). As mentioned above, the detection of Al3+ ion bases on the color change of TADA–AuNPs, the color difference between red and blue is more obvious than that between purple and blue. Therefore, dark red-colored TADA–AuNPs solutions synthesized with Na3Ct/HAuCl4 molar ratio larger than 6.4
:
1 are suitable for the colorimetric detection of Al3+. Subsequently, TADA–AuNPs synthesized under different Na3Ct/HAuCl4 molecular ratios were used as Al3+ colorimetric detection sensors, and the detection limits of Al3+ determined by the naked eye (naked eye detection limit of Al3+) are illustrated in Table 2. While purple-colored TADA–AuNPs showed no obviously response to Al3+, the red-colored TADA–AuNPs turned blue by adding Al3+ and had detection limits of 1.5 and 2.0 μM for Na3Ct/HAuCl4 molar ratios 6.4
:
1 and 12.8
:
1 synthetic NPs, respectively. This phenomenon may be due to the different surface area-volume ratio of gold nanoparticles in different sizes (see Fig. S2 in ESI†). Since the smaller the diameter, the larger the surface area-volume ratio, which may affect the optical properties of gold nanoparticles, thereby influencing the detection sensitivity.50 The TADA–AuNPs synthesized with Na3Ct/HAuCl4 (molar ratio of 6.4
:
1) had a lower naked eye detection limit, and therefore was selected as the Al3+ colorimetric detection sensor. The effect of the TADA–AuNPs concentration on the naked eye detection limit of Al3+ was further investigated, and the results are exhibited in Table 3. The naked eye detection limit of Al3+ increased with the increase of TADA–AuNPs concentration. For enhanced detection sensitivity, 0.1 mM TADA–AuNPs synthesized using 6.4
:
1 Na3Ct/HAuCl4 molecular ratio was chosen to construct the colorimetric sensor for Al3+ detection and used in the following studies.
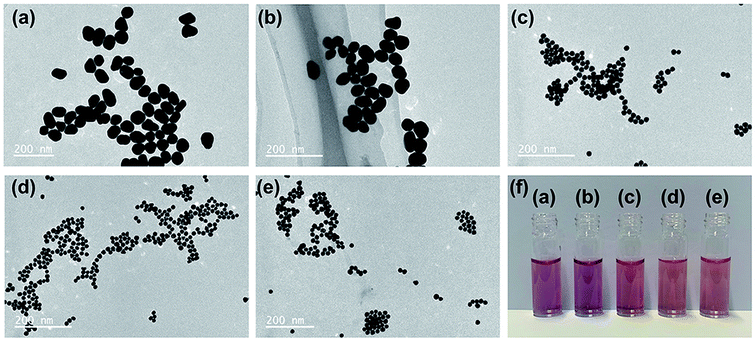 |
| Fig. 4 TEM images (a–e) and photographic images (f) of synthesized TADA–AuNPs solutions in different Na3Ct/HAuCl4 molar ratios using an initial HAuCl4 concentration of 0.25 mM. The Na3Ct/HAuCl4 molar ratios in a, b, c, d, and e are 0.8 : 1, 1.6 : 1, 3.2 : 1, 6.4 : 1, and 12.8 : 1, respectively. | |
Table 2 Optimization of Na3Ct/HAuCl4 molar ratio for Al3+ determination via the naked eye
Na3Ct/HAuCl4 molar ratio |
TADA–AuNPs solution color |
Naked eye detection limit of Al3+ (μM) |
0.8 : 1 |
Purple |
No obvious color change |
1.6 : 1 |
Purple |
No obvious color change |
3.2 : 1 |
Slight purple |
No obvious color change |
6.4 : 1 |
Dark-red |
1.5 |
12.8 : 1 |
Dark-red |
2.0 |
Table 3 Optimization of TADA–AuNPs concentration (Na3Ct/HAuCl4 = 6.4
:
1) for Al3+ determination via the naked eye
Concentration of TADA–AuNPs (mM) |
Naked eye detection limit of Al3+ (μM) |
0.025 |
Too light color, not suitable for sensor |
0.05 |
1 |
0.1 |
1.5 |
0.2 |
2.5 |
0.3 |
3.5 |
0.4 |
4.5 |
0.5 |
5.5 |
0.55 |
Too dark color, not suitable for sensor |
3.2. Method selectivity
In order to evaluate the method selectivity, the UV-vis absorption spectra of TADA–AuNPs solution reacting with Al3+and 21 common interference ions, including 18 metal ions (Cr3+, Fe3+, Hg2+, Pb2+, In3+, Ga3+, Cr6+, Mg2+, Fe2+, Mn2+, Co2+, Cd2+, Ca2+, Ba2+, Cu2+, Ni2+, Na+, K+) and 3 non-metal (NO3−, SO42−, PO43−) ions were tested. In addition, 10 mM diphenyl carbazone (DPC) was used as a Cr3+ masking agent. As shown in Fig. 5a, both Al3+ and Cr3+ can induce the TADA–AuNPs aggregation without DPC, leading to a color change from dark red to blue and an SPR absorption peak shift from 525 nm to 650 nm. While in the presence of DPC, the interference of Cr3+ is eliminated and only Al3+ can induce TADA–AuNPs aggregation (Fig. 5b). Other ions do not influence the solution color and the SPR absorption spectra in the presence or absence of the masking agent, illustrating no aggregation occurred. For quantitative testing of Al3+, DPC was used as a Cr3+ masking agent, and the competitive experiments of Al3+ (4 μM) with the 21 interference ions (50 μM) mentioned above were carried out, using the relative UV-vis absorption ratio (A650 nm/A525 nm) to evaluate the degree of AuNPs aggregation under various parameters. The higher the ratio of A650 nm/A525 nm, the more severe aggregation of TADA–AuNPs was observed. The results in Fig. 5c illustrate that the UV-vis absorption ratios (A650 nm/A525 nm) of TADA–AuNPs binding with 21 interference ions are much lower than that of Al3+. Additionally, the A650 nm/A525 nm ratios (black bars) in the presence of interfering ions are almost consistent with that of the absence interfering ions, which further showed that only Al3+ can induce TADA–AuNPs aggregation. This Al3+ colorimetric detection method shows excellent selectivity.
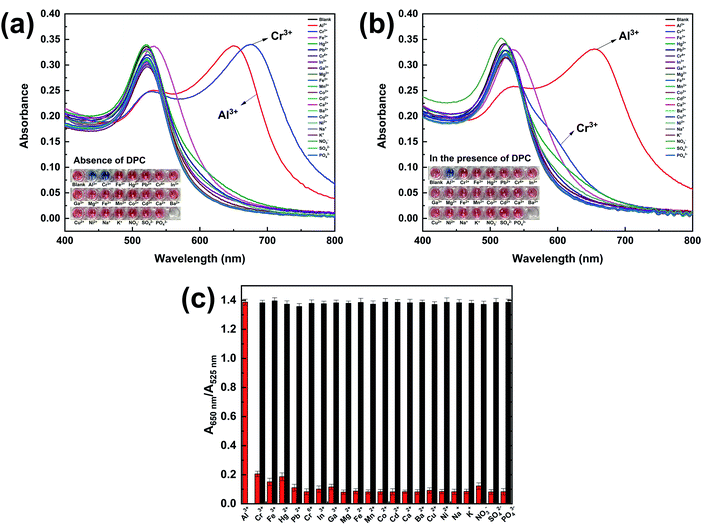 |
| Fig. 5 UV-vis absorption spectra and photographic images (inset) of TADA–AuNPs solution in the presence of various ions (a) without and (b) with DPC masking agent (10 mM); (c) UV-vis absorption ratios (A650 nm/A525 nm) of TADA–AuNP solutions reacting individually with various inference ions. The red bars and the black bars represent the individual ions and the single interference ion mixed with Al3+, respectively. The concentration of Al3+ was 4 μM. The concentration of the other ions was 50 μM. | |
3.3. Optimization of detection parameters for Al3+ ion
According to the principle of the Al3+ colorimetric sensor, the experimental conditions of pH and detection time had great effects on the performance of the test method. In order to explore the effective pH range for Al3+ detection, a pH titration test of TADA–AuNPs without Al3+ was conducted. The SPR absorption of TADA–AuNPs in the pH range of 1–13 was performed, and the corresponding photographic images are shown in Fig. 6a. When the pH ranged from 4 to 12, the UV-vis absorption spectra of TADA–AuNPs exhibited a single peak at 525 nm, and the colors of the corresponding solutions were dark red. This indicates that TADA–AuNPs are stable under these pH conditions. Conversely, when the pH was <4 or >12, the UV-vis absorption spectra of TADA–AuNPs exhibited a red-shifted new peak. Additionally, the color of the corresponding solution became blue, illustrating that the TADA–AuNPs under these pHs formed aggregates. The reason why aggregation happens in strongly acidic and basic conditions may be because of the chemical changes on the surface of the gold nanoparticles. When pH < 4, after being protonated, negatively charged citrate may reduce its binding ability with AuNPs, resulting in a decrease in the electrostatic repulsion ability of the AuNPs; At the same time, the protonated TADA may reduce its coordination ability with AuNPs, making it unable to protect AuNPs. Under the combined effect of the two, AuNPs aggregated.51 When pH > 12, under strong alkaline conditions, the carboxyl groups of citrate and TADA may be deprotonated, and their coordination ability with AuNPs may decrease, making them unable to protect AuNPs, leading to aggregation of AuNPs covered with hydroxyl groups.18 Thus, the effects of the pH range from 4 to 12 on the relative UV-vis absorption ratio (A650 nm/A525 nm) was further studied in the presence of Al3+, and the results are shown in Fig. 6b. At pH 4–10, the stable TADA–AuNP solution underwent “interparticle cross-linked” aggregation in the presence of Al3+ (4 μM). The color changed from dark red to blue and the A650 nm/A525 nm ratio increased. While at pH 11–12, the TADA–AuNPs solution still stabled, with the appearance of a dark red color and a decrease in the A650 nm/A525 nm ratio. This is due to the interaction between OH− and Al3+, forming the Al(OH)3 colloid. Therefore, the optimal pH range for the Al3+ assay is 4–10. The pH of 6.3 was selected for the following studies.
 |
| Fig. 6 (a) The change in UV-vis absorption spectra of TADA–AuNPs solution (0.1 mM) with pH and the corresponding photographic images (inset); (b) changes in UV-vis absorption ratios (A650 nm/A525 nm) of the mixed solution of TADA–AuNPs (0.1 mM) and Al3+ (4 μM) as a function of pH and the corresponding photographic images (inset); (c) changes in UV-vis absorption ratios (A650 nm/A525 nm) of the mixed solution of TADA–AuNPs (0.1 mM) and Al3+ (4 μM) as a function of pH and the corresponding photographic images (inset). All of the above are in the presence of the DPC masking agent (10 mM). | |
The effect of detection time on the relative UV-vis absorption ratio (A650 nm/A525 nm) of TADA–AuNPs mixed with different concentrations of Al3+ (0–4 μM) was investigated. As shown in Fig. 6c, the value of A650 nm/A525 nm depends on the concentration of Al3+. A650 nm/A525 nm increased rapidly with the increase of detection time and then became steady. Additionally, with the increase in Al3+ concentration, the detection time to saturation decreased. For 4 μM Al3+, the A650 nm/A525 nm ratio reached saturation when the detection time was 90 s, which means that Al3+ has induced the aggregation of TADA–AuNPs completely. Thus, the optimal detection time is 90 s.
3.4. Detection sensitivity
Under the optimal detection parameters, the detection sensitivity of this colorimetric sensor for Al3+ was studied, and the results are outlined in Fig. 7. There is a good linear correlation between A650 nm/A525 nm and Al3+ concentration in the range of 1.5–4.0 μM (R2 = 0.9976), from which the detection limit of Al3+ was determined to be 15 nM (Fig. S3 in ESI,† 3 times signal to noise ratio (S/N)). More importantly, this colorimetric assay can be used for visual determination of Al3+. As shown in the inset photograph in Fig. 7, when the Al3+ concentration was 1.5 μM, the color of the TADA–AuNP solution began to turn from dark red to violet, and this change could be easily identified and detected by the naked eye. Thus, the detection limit of Al3+ via naked eye is 1.5 μM, which is much lower than the current limit (3.7 μM) for drinking water in the WHO regulation and better than the previous reports.
 |
| Fig. 7 UV-vis absorption spectra of TADA–AuNPs bound with various Al3+concentrations (0, 1.50, 1.75, 2.00, 2.25, 2.50, 2.75, 3.00, 3.25, 3.50, 3.75, and 4.00 μM) in the presence of the DPC masking agent (10 mM) and the corresponding photographic images (inset) and the linear relationship between A650 nm/A525 nm absorbance ratio and Al3+ concentration. | |
3.5. Application in environmental water samples
Recovery experiments performed on spiked river water samples were carried out to determine the practical application effect of the as-constructed Al3+ colorimetric sensor. The water samples were spiked with four concentration levels of Al3+ (1.50, 2.50, 3.50, and 4.00 μM) after filtration through a 0.2 μm membrane. The Al3+ concentration in each sample was analyzed by the colorimetric sensor through UV-vis spectra (Fig. S4†) and the calculated results are shown in Table 4. The recoveries ranged from 95%–109%, and the relative standard deviations (RSD) were less than 3.5% (n = 3). This indicates that the designed TADA–AuNPs sensor is applicable for Al3+ detection in water samples.
Table 4 Detection of Al3+ in spiked real water samples
Added concentration of Al3+ (μM) |
Found concentration of Al3+a (μM) |
Recovery (%) |
RSD (%) |
Detection based on three independent samples (n = 3); values: mean ± SD. |
0 |
— |
— |
— |
1.50 |
1.63 ± 0.046 |
108.7 |
2.8 |
2.50 |
2.54 ± 0.086 |
101.6 |
3.4 |
3.50 |
3.58 ± 0.11 |
102.3 |
3.1 |
4.00 |
3.82 ± 0.057 |
95.5 |
1.5 |
4. Conclusions
A selective, sensitive, rapid, low-cost and simple colorimetric system for the visual detection of Al3+ based on 1,2,3-triazole-4,5-dicarboxylic acid (TADA)-functionalized gold nanoparticles (TADA–AuNPs) has been successfully developed. This sensing system shows high selectivity for Al3+ quantitative detection using diphenyl carbazone (DPC) as a Cr3+ masking agent. Moreover, the sensing system has the advantages of fast response (90 s) and low detection limits, which are 15 nM and 1.5 μM for UV-vis detection and naked eye identification, respectively. The detection limits are much lower than the current limit (3.7 μM) for drinking water in the WHO regulation. In addition, this colorimetric sensing system can be used to accurately detect the Al3+ in real water samples, and it shows great potential for rapid, in situ and real-time determination of trace Al3+. More significantly, this colorimetric assay can be easily modified to detect other element ions or restricted substances. Simply changing the ligand to recognize the target analyte allows for versatile and broad application. The sensor shows great potential for food safety, agriculture, and environmental monitoring applications.
Conflicts of interest
There are no conflicts of interest to declare.
Acknowledgements
The project was funded by Key Field (Rural Revitalization) Project of Colleges and Universities in Guangdong Province, China (2020ZDZX1009), Basic Research Project of Shenzhen Natural Science Foundation (JCYJ20190813103601660), and Shenzhen Technology University Self-Made Experimental Equipment Project (2020XZY009).
Notes and references
- Z. M. Shen, A. Perczel, M. Hollosi, I. Nagypal and G. D. Fasman, Study of Al3+ Binding and Conformational Properties of the Alanine-Substituted C-Terminal Domain of the NF-M Protein and Its Relevance to Alzheimer's Disease, Biochemistry, 1994, 33, 9627–9636 CrossRef CAS PubMed.
- A. L. Suhermana, E. E. L Tannera, S. Kussa, S. V. Sokolova, J. Holter, N. P. Young and R. G. Comptona, Voltammetric Determination of Aluminium(III) at Tannic Acid capped-Gold Nanoparticle Modified Electrodes, Sens. Actuators, B, 2018, 265, 682–690 CrossRef.
- R. Painulia, P. Joshia and D. Kumar, Cost-Effective Synthesis of Bifunctional Silver Nanoparticles for Simultaneous Colorimetric Detection of Al(III) and Disinfection, Sens. Actuators, B, 2018, 272, 79–90 CrossRef.
- Guidelines For Drinking-Water Quality: Fourth Edition Incorporating the First Addendum, World Health Organization, Geneva, 4th edn, 2017 Search PubMed.
- N. Altunay, E. Yildirim and R. Gürkan, Extraction and Preconcentration of Trace Al and Cr from Vegetable Samples by Vortex-Assisted Ionic Liquid-Based Dispersive Liquid-Liquid Microextraction Prior to Atomic Absorption Spectrometric Determination, Food Chem., 2018, 245, 586–594 CrossRef CAS PubMed.
- J. Chamier, M. Wicht, L. Cyster and N. P. Ndindi, Aluminium (Al) Fractionation and Speciation; Getting Closer to Describing the Factors Influencing Al3+ in Water Impacted by Acid Mine Drainage, Chemosphere, 2015, 130, 17–23 CrossRef CAS PubMed.
- A. Martín-Cameán, A. Jos, M. Puerto, A. Calleja, A. Iglesias-Linares, E. Solano and A. M. Cameán, In Vivo Determination of Aluminum, Cobalt, Chromium, Copper, Nickel, Titanium and Vanadium in Oral Mucosa Cells From Orthodontic Patients with Mini-Implants by Inductively Coupled Plasma-Mass Spectrometry (ICP-MS), J. Trace Elem. Med. Biol., 2015, 32, 13–20 CrossRef PubMed.
- S. Gao, L. W. Zhang, G. H. Wang, K. Yang, M. L. Chen, R. Tian, Q. J. Ma and L. Zhu, Hybrid Graphene/Au Activatable Theranostic Agent for Multimodalities Imaging Guided Enhanced Photothermal Therapy, Biomaterials, 2016, 79, 36–45 CrossRef CAS PubMed.
- L. Zhan, S. Z. Guo, F. Y. Song, Y. Gong, F. Xu, D. R. Boulware, M. C. McAlpine, W. C. W. Chan and J. C. Bischof, The Role of Nanoparticle Design in Determining Analytical Performance of Lateral Flow Immunoassays, Nano Lett., 2017, 17, 7207–7212 CrossRef CAS PubMed.
- J. J. Zhang, L. Mou and X. Y. Jiang, Chemistry of Gold Nanoparticles for Health Related Applications, Chem. Sci., 2020, 11, 923–936 RSC.
- H. B. Dong, F. Zou, X. J. Hu, H. Zhu, K. Koh and H. X. Chen, Analyte Induced AuNPs Aggregation Enhanced Surface Plasmon Resonance for Sensitive Detection of Paraquat, Biosens. Bioelectron., 2018, 117, 605–612 CrossRef CAS PubMed.
- Y. Y. Qi, J. X. Ma, X. D. Chen, F. R. Xiu, Y. T. Chen and Y. W. Lu, Practical Aptamer-Based Assay of Heavy Metal Mercury Ion in Contaminated Environmental Samples: Convenience and Sensitivity, Anal. Bioanal. Chem., 2020, 412, 439–448 CrossRef CAS PubMed.
- H. Z. Yuan, W. Ji, S. W. Chu, Q. Liu, S. Y. Qian, J. Y. Guang, J. B. Wang, X. Y. Han, J.-F. Masson and W. Peng, Mercaptopyridine-Functionalized Gold Nanoparticles for Fiber-Optic Surface Plasmon Resonance Hg2+ Sensing, ACS Sens., 2019, 4, 704–710 CrossRef CAS PubMed.
- Y. X. Xia, C. X. Zhu, J. Bian, Y. X. Li, X. Y. Liu and Y. Liu, Highly Sensitive and Selective Colorimetric Detection of Creatinine Based on Synergistic Effect of PEG/Hg2+–AuNPs, Nanomaterials, 2019, 9, 1424–1438 CrossRef CAS PubMed.
- K. Mao, H. Zhang, Z. L. Wang, H. R. Cao, K. K. Zhang, X. Q. Li and Z. G. Yang, Nanomaterial-Based Aptamer Sensors for Arsenic Detection, Biosens. Bioelectron., 2020, 148, 111785–111800 CrossRef CAS PubMed.
- M. Solra, R. Bala, N. Wangoo, G. K. Soni, M. Kumar and R. K. Sharma, Optical Pico-Biosensing of Lead Using Plasmonic Gold Nanoparticles and a Cationic Peptide-Based Aptasensor, Chem. Commun., 2020, 56, 289–292 RSC.
- Y. Gan, T. Liang, Q. W. Hu, L. J. Zhong, X. Y. Wang, H. Wan and P. Wang, In situ Detection of Cadmium with Aptamer Functionalized Gold Nanoparticles Based on Smartphone-Based Colorimetric system, Talanta, 2020, 208, 120231–120238 CrossRef CAS PubMed.
- P. Mondal and J. L. Yarger, Colorimetric Dual Sensors of Metal Ions Based on 1,2,3-Triazole-4,5-Dicarboxylic Acid-Functionalized Gold Nanoparticles, J. Phys. Chem. C, 2019, 123, 20459–20467 CrossRef CAS.
- Y. Y. Qi, Y. T. Chen, F. R. Xiu and J. X. Hou, An Aptamer-Based Colorimetric Sensing of Acetamiprid in Environmental Samples: Convenience, Sensitivity and Practicability, Sens. Actuators, B, 2020, 304, 127359–127367 CrossRef CAS.
- Q. Tu, T. X. Yang, Y. Q. Qu, S. Y. Gao, Z. Y. Zhang, Q. M. Zhang, Y. L. Wang, J. Y. Wang and L. L. He, In Situ Colorimetric Detection of Glyphosate on Plant Tissues Using Cysteamine-Modified Gold Nanoparticles, Analyst, 2019, 144, 2017–2025 RSC.
- M. Jia, J. Y. Sha, Z. H. Li, W. J. Wang and H. Y. Zhang, High Affinity Truncated Aptamers for Ultra-Sensitive Colorimetric Detection of Bisphenol A with Label-Free Aptasensor, Food Chem., 2020, 317, 126459–126467 CrossRef CAS PubMed.
- E. H. Lee, S. K. Lee, M. J. Kim and S. W. Lee, Simple and Rapid Detection of Bisphenol A Using a Gold Nanoparticle-Based Colorimetric Aptasensor, Food Chem., 2019, 287, 205–213 CrossRef CAS PubMed.
- A. R. Esfahani, Z. Sadiq, O. D. Oyewunmi, S. H. S. Tali, N. Usen, D. C. Boffito and S. Jahanshahi-Anbuhi, Portable, Stable, and Sensitive Assay to Detect Phosphate in Water With Gold Nanoparticles (AuNPs) and Dextran Tablet, Analyst, 2021, 146, 3697–3708 RSC.
- Z. Q. Chen, L. A. Chen, L. Lin, Y. N. Wu and F. F. Fu, A Colorimetric Sensor for the Visual Detection of Azodicarbonamide in Flour Based on Azodicarbonamide-Induced Anti-Aggregation of Gold Nanoparticles, ACS Sens., 2018, 3, 2145–2151 CrossRef CAS PubMed.
- Y. Y. Wu, P. C. Huang and F. Y. Wu, A Label-Free Colorimetric Aptasensor Based on Controllable Aggregation of AuNPs for the Detection of Multiplex Antibiotics, Food Chem., 2020, 304, 125377–125384 CrossRef CAS PubMed.
- J. J. Zhou, Y. Q. Li, W. J. Wang, Z. C. Lu, H. Y. Han and J. W. Liu, Kanamycin Adsorption on Gold Nanoparticles Dominates Its Label Free Colorimetric Sensing with Its Aptamer, Langmuir, 2020, 36, 11490–11498 CrossRef CAS PubMed.
- Y. Y. Wu, B. W. Liu, P. C. Huang and F. Y. Wu, A Novel Colorimetric Aptasensor for Detection of Chloramphenicol Based on lanthanum Ion–Assisted Gold Nanoparticle Aggregation and Smartphone Imaging, Anal. Bioanal. Chem., 2019, 411, 7511–7518 CrossRef CAS PubMed.
- F. Zhang, P. J. J. Huang and J. W. Liu, Sensing Adenosine and ATP by Aptamers and Gold Nanoparticles: Opposite Trends of Color Change from Domination of Target Adsorption Instead of Aptamer Binding, ACS Sens., 2020, 5, 2885–2893 CrossRef CAS PubMed.
- W. Zhou, K. Q. Hu, S. Kwee, L. Tang, Z. H. Wang, J. F. Xia and X. J. Li, Gold Nanoparticle Aggregation-Induced Quantitative Photothermal Biosensing Using a Thermometer: a Simple and Universal Biosensing Platform, Anal. Chem., 2020, 92, 2739–2747 CrossRef CAS PubMed.
- G. Tatulli and P. P. Pompa, An Amplification-Free Colorimetric Test for Sensitive DNA Detection Based on the Capturing of Gold Nanoparticle Clusters, Nanoscale, 2020, 12, 15604–15610 RSC.
- X. X. Liu, F. He, F. Zhang, Z. J. Zhang, Z. C. Huang and J. W. Liu, Dopamine and Melamine Binding to Gold Nanoparticles Dominates Their Aptamer-Based Label-Free Colorimetric Sensing, Anal. Chem., 2020, 92, 9370–9378 CrossRef CAS PubMed.
- Y. R. Ren, J. Wei, Y. X. He, Y. Wang, M. F. Bai, C. Zhang, L. P. Luo, J. L. Wang and Y. R. Wang, Ultrasensitive Label-Free Immunochromatographic Strip Sensor for Salmonella Determination Based on Salt-Induced Aggregated Gold Nanoparticles, Food Chem., 2021, 343, 128518–128526 CrossRef CAS PubMed.
- L. Y. Zheng, G. Z. Cai, W. Z. Qi, S. Y. Wang, M. H. Wang and J. H. Lin, Optical Biosensor for Rapid Detection of Salmonella Typhimurium Based on Porous Gold@Platinum Nanocatalysts and a 3D Fluidic Chip, ACS Sens., 2020, 5, 65–72 CrossRef CAS PubMed.
- H. Kim, M. Park, J. Hwang, J. H. Kim, D. R. Chung, K. S. Lee and M. Kang, Development of Label-Free Colorimetric Assay for MERS-CoV Using Gold Nanoparticles, ACS Sens., 2019, 4, 1306–1312 CrossRef CAS PubMed.
- P. Moitra, M. Alafeef, K. Dighe, M. B. Frieman and D. Pan, Selective Naked-Eye Detection of SARS-CoV-2 Mediated by N Gene Targeted Antisense Oligonucleotide Capped Plasmonic Nanoparticles, ACS Nano, 2020, 14, 7617–7627 CrossRef CAS PubMed.
- R. Meena, V. N. Mehta, J. R. Bhamore, P. T. Rao, T. J. Park and S. K. Kailasa, Diaminodiphenyl Sulfone as a Novel Ligand for Synthesis of Gold Nanoparticles for Simultaneous Colorimetric Assay of Three Trivalent Metal Cations (Al3+, Fe3+ and Cr3+), J. Mol. Liq., 2020, 312, 113409–113417 CrossRef CAS.
- L. Rastogi, K. Dash and A. Ballal, Selective Colorimetric/Visual Detection of Al3+ in Ground Water Using Ascorbic Acid Capped Gold Nanoparticles, Sens. Actuators, B, 2017, 248, 124–132 CrossRef CAS.
- R. Q. Zhu, J. P. Song, Q. Ma, Y. Zhou, J. Yang, S. M. Shuang and C. Dong, A Colorimetric Probe for the Detection of Aluminum Ions Based on 11-Mercaptoundecanoic Acid Functionalized Gold Nanoparticles, Anal. Methods, 2016, 8, 7232–7236 RSC.
- D. S. Xue, H. Y. Wang and Y. B. Zhang, Specific and Sensitive Colorimetric Detection of Al3+ using 5- mercaptomethyltetrazole Capped Gold Nanoparticles in Aqueous Solution, Talanta, 2014, 119, 306–311 CrossRef CAS PubMed.
- A. Kumar, M. Bhatt, G. Vyas, S. Bhatt and P. Paul, Sunlight Induced Preparation of Functionalized Gold Nanoparticles as Recyclable Colorimetric Dual Sensor for Aluminum and Fluoride in Water, ACS Appl. Mater. Interfaces, 2017, 9, 17359–17368 CrossRef CAS PubMed.
- Y. C. Chen, I. L. Lee, Y. M. Sung and S. P. Wu, Colorimetric Detection of Al3+ Ions Using Triazole–Ether Functionalized Gold Nanoparticles, Talanta, 2013, 117, 70–74 CrossRef CAS PubMed.
- X. L. Luo, X. Xie, Y. C. Meng, T. L. Sun, J. S. Ding and W. H. Zhou, Ligands Dissociation Induced Gold Nanoparticles Aggregation for Colorimetric Al3+ Detection, Anal. Chim. Acta, 2019, 1087, 76–85 CrossRef CAS PubMed.
- N. Garg, S. Bera and A. Ballal, SPR Responsive Xylenol Orange Functionalized Gold Nanoparticles- Optical Sensor for Estimation of Al3+ in Water, Spectrochim. Acta, Part A, 2020, 228, 117701–117711 CrossRef CAS PubMed.
- D. Böhme, N. Düpre, D. A. Megger and J. Müller, Conformational Change Induced by Metal-Ion-Binding to DNA Containing the Artificial 1,2,4-Triazole Nucleoside, Inorg. Chem., 2007, 46, 10114–10119 CrossRef PubMed.
- Y. C. Chen, I. L. Lee, Y. M. Sung and S. P. Wu, Triazole Functionalized Gold Nanoparticles for Colorimetric Cr3+ Sensing, Sens. Actuators, B, 2013, 188, 354–359 CrossRef CAS.
- M. Tiwari, S. Gupta and R. Prakash, One Pot Synthesis of Coordination Polymer 2,5-Dimercapto-1,3,4-Thiadiazole–Gold and its Application in Voltammetric Sensing of Resorcinol, RSC Adv., 2014, 4, 25675–25682 RSC.
- V. N. Mehta, R. K. Singhal and S. K. Kailasa, A Molecular Assembly of Piperidine Carboxylic Acid Dithiocarbamate on Gold Nanoparticles for the Selective and Sensitive Detection of Al3+ Ion in Water Samples, RSC
Adv., 2015, 5, 33468–33477 RSC.
- R. Klajn, J. F. Stoddart and B. A. Grzybowski, Nanoparticles Functionalised with Reversible Molecular and Supramolecular Switches, Chem. Soc. Rev., 2010, 39, 2203–2237 RSC.
- X. H. Ji, X. N. Song, J. Li, Y. B. Bai, W. S. Yang and X. G. Peng, Size Control of Gold Nanocrystals in Citrate Reduction: the Third Role of Citrate, J. Am. Chem. Soc., 2007, 129, 13939–13948 CrossRef CAS PubMed.
- E. Priyadarshini and N. Pradhan, Gold Nanoparticles as Efficient Sensors in Colorimetric Detection of Toxic Metal Ions: A Review, Sens. Actuators, B, 2017, 238, 888–902 CrossRef CAS.
- D. Y. Long and H. L. Yu, A Synergistic Coordination Strategy for Colorimetric Sensing of Chromium(III) Ions Using Gold Nanoparticles, Anal. Bioanal. Chem., 2016, 408, 8551–8557 CrossRef CAS PubMed.
Footnote |
† Electronic supplementary information (ESI) available. See DOI: 10.1039/d1ra04834a |
|
This journal is © The Royal Society of Chemistry 2021 |
Click here to see how this site uses Cookies. View our privacy policy here.