DOI:
10.1039/D1RA04772H
(Paper)
RSC Adv., 2021,
11, 27583-27588
Removal of toluene as a toxic VOC from methane gas using a non-thermal plasma dielectric barrier discharge reactor
Received
20th June 2021
, Accepted 2nd August 2021
First published on 12th August 2021
Abstract
Methane is the main component of biogas, which could be used as a renewable energy source for electricity, source of heat, and biofuel production after upgrading from biogas. It also contains toxic compounds which cause environmental and human health problems. Therefore, in this work, the removal of a toxic compound (toluene) from methane gas was studied using a dielectric barrier discharge (DBD) reactor. It was observed that the removal of the toxic compound could be achieved from methane carrier gas using a dielectric barrier discharge reactor, and it depends on plasma input power. The maximum removal of the toxic compound was 85.9% at 40 W and 2.86 s. The major gaseous products were H2 and lower hydrocarbons (LHC) and the yield of these products also increases with input power. In the current study, the yield of gaseous products depends on the decomposition of toxic compounds and methane, because the decomposition of methane also produces H2 and lower hydrocarbons. The percentage yield of H2 increases from 0.43–4.74%. Similarly, the yield of LHC increases from 0.56–7.54% under the same reaction conditions. Hence, input power promoted the decomposition of the toxic compound and enhanced the yield of gaseous products.
1. Introduction
In recent years, non-thermal plasma (NTP) has received significant attention in gas cleaning and removal of VOCs due to its simple operation and the ability to handle high and low concentrations of VOCs.1–11 NTP is considered as non-equilibrium plasma due to a very high-temperature difference between electrons and heavy species. Electrons are produced and accelerated by the electric field, which collide with the molecules and atoms passing through the plasma reactor. Some electrons have sufficient energy to ionize these gas atoms or molecules upon collision. The energy required to dissociate/ionize the background gases is in the range of 5–25 eV.12 It was observed that a sufficient number of high energy electrons are present in a Maxwellian distribution to dissociate/ionize background gas molecules.13 The molecules, ions, and gas atoms remain at modest temperature, whereas electrons in comparison are very hot. It was reported that the input power absorbed by the electrons was very high (i.e. 102–103 times) compared to the heavy particles.14 However, the energy transferred from electrons to heavy particles is small due to the lower mass of the electrons.15
The treatment of pollutants via NTP is mostly carried using a dielectric barrier discharge (DBD) due to its ease of operation and the availability of an efficient and economical power supply.16–23 Due to the combined effect of non-equilibrium plasma properties and ease of operation at ambient pressure, DBD reactors are being used for industrial applications.24,25 The ability of the DBD reactor to simple scaling up is also a well-known feature for the commercial applicability of this process.26,27 Moreover, cost-effective and reliable power supplies are also easily available to generate NTP in DBD reactors. The frequency range (1 kHz to 10 MHz) and the pressure range (10–500 kPa) are normally preferred for industrial applications of the DBD reactor.28 Currently, DBD reactors are widely used for a range of industrial applications such as the treatment of pollutants causing anthropogenic emissions into the atmosphere, ozone generation, surface treatment, ultraviolet lamps, mercury-free fluorescent lamps, and large-area flat plasma displays.28,29
Recently, many researchers focused on the removal of VOCs using air as a carrier gas.30–34 However, in biogas, the major component of gas is CH4, which could be up to 75% depending on the type of feedstock and operating condition of the digester.35 To the best of our knowledge, the removal of the toxic compound using CH4 as a carrier gas in a DBD reactor has not been studied so far. Therefore, it was very important to investigate the removal of toluene using CH4 as a carrier gas to evaluate the performance of NTP. Herein, toluene was chosen as a model compound due to its presence in abundance in biogas. Toluene is also used in many industrial processes.36 The concentration of VOCs was kept higher (33 g Nm−3) than the amount of VOCs present in biogas, leaving operational margin for scaling up into industrial practice.37
2. Experimental setup
Fig. 1 shows the schematic diagram of the DBD reactor. The NTP coaxial DBD reactor was used to investigate the decomposition of toluene. Stainless steel (SS) external electrode (30 mm) was wrapped outside the external quartz tube with a 15 mm inner diameter. The inner SS electrode was placed inside the inner quartz tube having an outer diameter of 12 mm. The plasma was produced between the annular spaces of the coaxial quartz tubes. A variac was connected to the plasma generator to control the discharge power to the DBD reactor. In this study, the input power was varied from 5 to 40 W and measured using an energy meter. The length of the discharge zone depends upon the outer electrode (shortest electrode).38
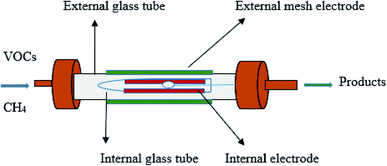 |
| Fig. 1 Schematic diagram of the DBD reactor. | |
The flow rate of the natural gas was controlled by using a computer-controlled mass flow controller, connected to the gas cylinder (BOC, UK). The natural gas was passed through a bubbler filled with toluene to add the vapours.
The gaseous products were measured by Varian 450-GC provided with a TCD (thermal conductivity detector) to monitor CH4 and H2, and FID (flame ionization detector) to detect lower hydrocarbons (LHC).
2.1 Definitions
The decomposition of toluene is defined as:
The yield of different product was defined as follows:
where
m > 1
The energy efficiency and specific input energy (SIE) are calculated using the following formulas:39
3. Results and discussion
3.1 Decomposition of VOC with changing the input power
Fig. 2 shows the removal of toluene using the DBD reactor. It can be observed that the removal of toluene increases with increasing input power. It is well known that increasing plasma input power increases the number of high-energy electrons.40,41 These high-energy electrons are responsible to increase the decomposition of VOCs, whereas they can also produce reactive species upon collision with background gas,42–44 which can also contribute to the high conversion of VOCs. Therefore, at high power combined effect of energetic electrons and reactive species may contribute to enhance the decomposition of target compounds. The bond dissociation energy of the –CH3 group in toluene is 3.7 eV,45 which is lesser than the C–C bond between the methyl group and aromatic ring, and bonds present in the aromatic ring.46 Hence, the decomposition of toluene can proceed via the removal of H from the –CH3 group. The abstraction of H from CH3 form the benzyl radicals (eqn (1)), which can further react with reactive species/energetic electrons to produce lower hydrocarbons (eqn (7)). The reactive species have enough energy which can break the C–C bond between the aromatic ring and methyl group and produce phenyl radicals. These radicals have a tendency to agglomerate with benzyl radicals and produce undesirable solid residues (eqn (6)). However, there are enough highly energetic electron and reactive species which can rupture the aromatic ring directly and produce lower hydrocarbons. The decomposition of background gas (CH4) also produces CH3 and H radicals (eqn (3)). These reactive species may also contribute to decompose VOC (eqn (4) and (5)). Eqn (1)–(7) show some reactions involved during the decomposition of toluene:39,47 |
C7H8 + e → H + C7H7 + e
| (1) |
|
C7H8 + e → CH3 + C6H5 + e
| (2) |
|
CH4 + e → CH3 + H + e
| (3) |
|
C7H8 + CH3 → CH4 + C7H7
| (5) |
|
C6H5 + C7H7 → polymerize
| (6) |
|
Toluene/intermediate products + e or active species → LHC (C1–C6) + e
| (7) |
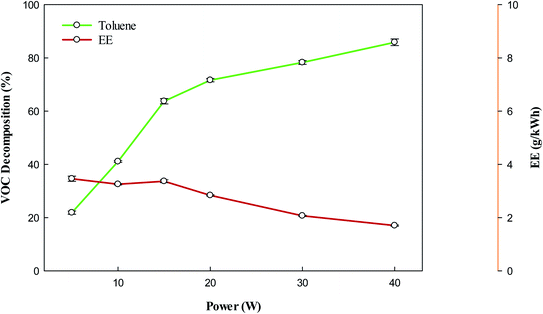 |
| Fig. 2 Effect of plasma input power on the conversion and energy efficiency of VOC. Reaction conditions: concentration, 33 g Nm−3; flow rate, 40 ml min−1; and temperature, ambient. | |
3.2 Decomposition of CH4
Fig. 3 shows that the decomposition of CH4 also increases with increasing input power due to the presence of a high number of reactive species at higher input power, which play a vital role in NTP processes.48 However, the effect of power is the same, increases with increasing input power, due to abundant reactive species at high powers. The decomposition of CH4 into CH3 and H by NTP forms a variety of products, and the composition of these products heavily depends on the plasma input power.
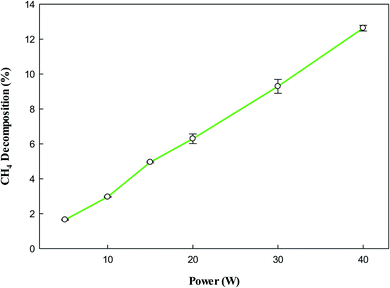 |
| Fig. 3 Effect of plasma input power on CH4 decomposition. Reaction conditions: concentration, 33 g Nm−3; flow rate, 40 ml min−1; and temperature, ambient. | |
3.3 Yield of gaseous products
Fig. 4 shows the yield of gaseous products from toluene decomposition. It was observed that the yield of all products increases with increasing input power. At higher input power energetic electrons have enough energy which can rupture the aromatic ring resulting in the formation of LHC. Secondly, it can be seen (Fig. 4) that amount of H2 increases at higher power, which can also contribute to the formation of LHC. The decomposition of CH4 could also contribute to the production of H2 and LHC. Moreover, it was observed that the impact of energetic electrons generates different radicals such as CH3, CH2, CH, and H. These radicals combine to produce different LHC via reactions (8)–(13).48–50 |
C2H6 + e → H + C2H5 + e
| (9) |
|
C3H8 + e → H + C3H7 + e
| (12) |
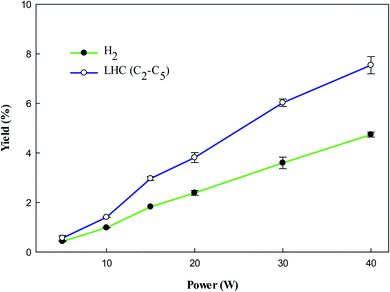 |
| Fig. 4 Effect of plasma input power on the yield of products. Reaction conditions: concentration, 33 g Nm−3; flow rate, 40 ml min−1; and temperature, ambient. | |
Fig. 5 shows the yield of individual LHC. The decomposition of VOC and background gas may contribute to the formation of LHC. In the plasma methane decomposition process the impact of electrons dissociate methane and produce CH3 (eqn (3)), which can combine to form C2 hydrocarbons (eqn (8)). Similarly, electron impact dissociation can produce a variety of radicals from different compounds (eqn (9) and (12)), which further recombine to produce >C2 hydrocarbons. Therefore, the yield of lower hydrocarbons and hydrogen collectively depends on the decomposition of VOC and methane carrier gas.
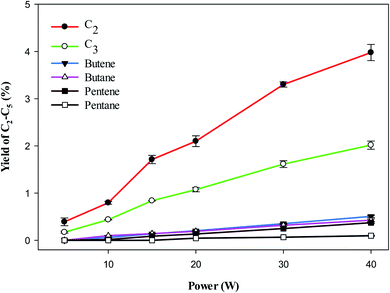 |
| Fig. 5 Effect of plasma input power on the yield of C2–C5. Reaction conditions: concentration, 33 g Nm−3; flow rate, 40 ml min−1; and temperature, ambient. | |
It has been observed that the reactions involved in NTP are power-dependent. The input power in the DBD reactor is the most important parameter. The input power plays a key role to produce active species, which produces ionization, dissociation, and excitation of background gas to start decomposition reactions. Therefore, it was reported that the reactions involved in NTP are energy-dependent.51 Fig. 6 shows the variation of ln
(C/C0)T of toluene with input power. The value of R2 for linear regression is 0.96, which shows that the decomposition of VOC compounds in the current experiment is first ordered.
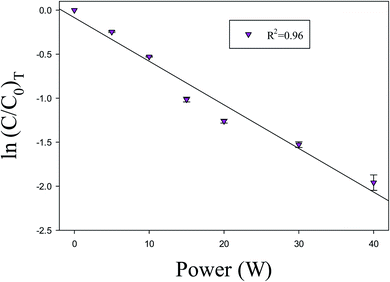 |
| Fig. 6 Power versus ln (C/C0). Reaction conditions: concentration, 33 g Nm−3; flow rate, 40 ml min−1; and temperature, ambient. | |
3.4 Effect of SIE
Fig. 7 shows the effect of specific input energy (SIE) on the decomposition of CH4 and products yield. It can be observed that the decomposition of methane and products yield increase linearly with increasing SIE. The SIE depends on input power and flow rate; however, the flow rate was kept constant in the current study. Due to this reason, SIE is closely related to plasma input power. Previous studies showed that increasing SIE enhanced the decomposition of methane and product yield.52 Overall, the major contributing factor is the input power because SIE only depends on power in this study.
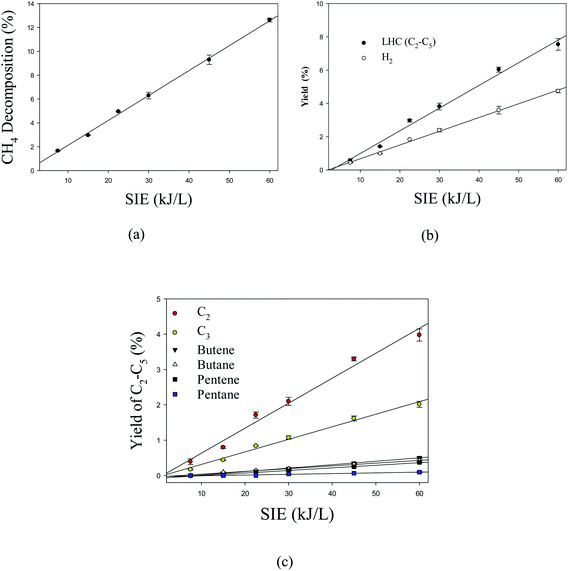 |
| Fig. 7 Effect of SIE on (a) the decomposition of CH4 (b) the product yield and (c) the yield of individual lower hydrocarbons. Reaction conditions: concentration, 33 g Nm−3; flow rate, 40 ml min−1; and temperature, ambient. | |
4. Conclusions
The decomposition of toxic VOC (toluene) has been studied in CH4 gas, which is the major component of biogas using the DBD reactor. The results show that the removal of VOC could be achieved from CH4 gas by using NTP dielectric barrier discharged reactor. However, the removal of VOC depends on input power. The highest decomposition of toluene at 40 W and 2.86 s was 85.9%. The major gaseous products were H2 and LHC. The effect of input power was the same on the yield of the products as in the case of decomposition efficiency. The decomposition of background gas may be affected due to the formation of methane through the decomposition of toluene, which may occur due to the recombination of CH3 and H radicals. However, it is difficult to measure such an effect due to the presence of methane as the carrier gas. Hence, the NTP dielectric barrier discharged reactor could be employed to remove VOCs from the methane gas.
Conflicts of interest
There are no conflicts to declare.
References
- F. Saleem, A. Harvey and K. Zhang, Fuel, 2019, 248, 258–261 CrossRef CAS.
- F. Saleem, K. Zhang and A. Harvey, Energy Fuels, 2019, 33, 2598–2601 CrossRef CAS.
- F. Saleem, K. Zhang and A. Harvey, Energy Fuels, 2019, 33, 389–396 CrossRef CAS.
- R. H. Rad, M. R. Khani, H. Mehdikia, M. Shafiei and B. Shokri, J. Environ. Health Sci. Eng., 2019, 17, 549–560 CrossRef CAS PubMed.
- B. Wang, C. Chi, M. Xu, C. Wang and D. Meng, Chem. Eng. J., 2017, 322, 679–692 CrossRef CAS.
- M. F. Mustafa, X. Fu, Y. Liu, Y. Abbas, H. Wang and W. Lu, J. Hazard. Mater., 2018, 347, 317–324 CrossRef CAS PubMed.
- F. Ghorbani Shahna, A. Bahrami, I. Alimohammadi, R. Yarahmadi, B. Jaleh, M. Gandomi, H. Ebrahimi and K. Ad-Din Abedi, J. Hazard. Mater., 2017, 324, 544–553 CrossRef CAS PubMed.
- X. Feng, C. Chen, C. He, S. Chai, Y. Yu and J. Cheng, J. Hazard. Mater., 2020, 383, 121143 CrossRef CAS PubMed.
- K. H. Hama Aziz, A. Mahyar, H. Miessner, S. Mueller, D. Kalass, D. Moeller, I. Khorshid and M. A. M. Rashid, Process Saf. Environ. Prot., 2018, 113, 319–329 CrossRef CAS.
- K. H. Hama Aziz, H. Miessner, S. Mueller, A. Mahyar, D. Kalass, D. Moeller, I. Khorshid and M. A. M. Rashid, J. Hazard. Mater., 2018, 343, 107–115 CrossRef CAS PubMed.
- K. H. Hama Aziz, H. Miessner, S. Mueller, D. Kalass, D. Moeller, I. Khorshid and M. A. M. Rashid, Chem. Eng. J., 2017, 313, 1033–1041 CrossRef CAS.
- E. W. McDaniel, J. B. A. Mitchell and M. E. Rudd, Atomic Collisions: Heavy Particle Projectiles, John Wiley and Sons, New York, 1993 Search PubMed.
- M. A. Lieberman and A. J. Lichtenberg, MRS Bull., 1994, 30, 899–901 Search PubMed.
- U. R. Kortshagen, R. M. Sankaran, R. N. Pereira, S. L. Girshick, J. J. Wu and E. S. Aydil, Chem. Rev., 2016, 116, 11061–11127 CrossRef CAS PubMed.
- V. E. E. Golant, A. P. Zhilinsky and I. E. E. Sakharov, Fundamentals of plasma physics, John Wiley & Sons, 1980 Search PubMed.
- H. Zhang, K. Li, C. Shu, Z. Lou, T. Sun and J. Jia, Chem. Eng. J., 2014, 256, 107–118 CrossRef CAS.
- H. Zhang, K. Li, T. Sun, J. Jia, Z. Lou and L. Feng, Chem. Eng. J., 2014, 241, 92–102 CrossRef CAS.
- Q. Jin, B. Jiang, J. Han and S. Yao, Chem. Eng. J., 2016, 286, 300–310 CrossRef CAS.
- C. Subrahmanyam, A. Renken and L. Kiwi-Minsker, Chem. Eng. J., 2010, 160, 677–682 CrossRef CAS.
- X. Yao, J. Zhang, X. Liang and C. Long, Chemosphere, 2018, 208, 922–930 CrossRef CAS PubMed.
- X. Yao, J. Zhang, X. Liang and C. Long, Chemosphere, 2019, 230, 479–487 CrossRef CAS PubMed.
- R. Huang, M. Lu, P. Wang, Y. Chen, J. Wu, M. Fu, L. Chen and D. Ye, RSC Adv., 2015, 5, 72113–72120 RSC.
- K. H. Hama Aziz, H. Miessner, A. Mahyar, S. Mueller, D. Kalass, D. Moeller and K. M. Omer, Sep. Purif. Technol., 2019, 216, 51–57 CrossRef CAS.
- D. Kuvshinov, J. Lozano-Parada, A. Siswanto and W. Zimmerman, World Acad. Sci. Eng. Technol. Int. J. Chem. Mol. Nucl. Mater. Metall. Eng., 2014, 8, 80–83 Search PubMed.
- Q. Wang, B. Spasova, V. Hessel and G. Kolb, Chem. Eng. J., 2015, 262, 766–774 CrossRef CAS.
- N. Singh, Scale-up of a dielectric-barrier-discharge plasma reactor and toluene removal efficiency, Oklahoma State University, 2007 Search PubMed.
- S. Tang, N. Lu, J. Li and Y. Wu, Sep. Purif. Technol., 2012, 95, 73–79 CrossRef CAS.
- U. Kogelschatz, Plasma Chem. Plasma Process., 2003, 23, 1–46 CrossRef CAS.
- X. Xu, Thin Solid Films, 2001, 390, 237–242 CrossRef CAS.
- O. Karatum and M. A. Deshusses, Chem. Eng. J., 2016, 294, 308–315 CrossRef CAS.
- H. Song, Y. Peng, S. Liu, S. Bai, X. Hong and J. Li, Plasma Chem. Plasma Process., 2019, 39, 1469–1482 CrossRef CAS.
- B. Zhu, L.-Y. Zhang, M. Li, Y. Yan, X.-M. Zhang and Y.-M. Zhu, Chem. Eng. J., 2020, 381, 122599 CrossRef CAS.
- L. Mao, Z. Chen, X. Wu, X. Tang, S. Yao, X. Zhang, B. Jiang, J. Han, Z. Wu, H. Lu and T. Nozaki, J. Hazard. Mater., 2018, 347, 150–159 CrossRef CAS PubMed.
- J. Chen, Z. Xie, J. Tang, J. Zhou, X. Lu and H. Zhao, Chem. Eng. J., 2016, 284, 166–173 CrossRef CAS.
- K. C. Surendra, D. Takara, A. G. Hashimoto and S. K. Khanal, Renewable Sustainable Energy Rev., 2014, 31, 846–859 CrossRef.
- B. Wang, C. Chi, M. Xu, C. Wang and D. Meng, Chem. Eng. J., 2017, 322, 679–692 CrossRef CAS.
- J. Sun, Q. Wang, W. Wang, Z. Song, X. Zhao, Y. Mao and C. Ma, Fuel, 2017, 207, 121–125 CrossRef CAS.
- A. H. Khoja, M. Tahir and N. A. S. Amin, Energy Convers. Manage., 2017, 144, 262–274 CrossRef CAS.
- F. Zhu, X. Li, H. Zhang, A. Wu, J. Yan, M. Ni, H. Zhang and A. Buekens, Fuel, 2016, 176, 78–85 CrossRef CAS.
- U. Kortshagen, Plasma Chem. Plasma Process., 2016, 36, 73–84 CrossRef CAS.
- J. Wu, Q. Xia, H. Wang and Z. Li, Appl. Catal., B, 2014, 156–157, 265–272 CrossRef CAS.
- F. Saleem, A. H. Khoja, J. Umer, F. Ahmad, S. Z. Abbas, K. Zhang and A. Harvey, J. Energy Inst., 2021, 96, 97–105 CrossRef CAS.
- F. Saleem, A. Rehman, A. Abbas, A. Hussain Khoja, F. Ahmad, L. Liu, K. Zhang and A. Harvey, J. Energy Inst., 2021, 97, 161–168 CrossRef CAS.
- U. H. Dahiru, F. Saleem, K. Zhang and A. P. Harvey, J. Environ. Chem. Eng., 2021, 9, 105023 CrossRef CAS.
- B. D. Darwent, NSRDS-NBS No. 31, U. S. Dept. Commerce, Washington, D. C, Jan 1970, p. 48.
- S. Y. Liu, D. H. Mei, M. A. Nahil, S. Gadkari, S. Gu, P. T. Williams and X. Tu, Fuel Process. Technol., 2017, 166, 269–275 CrossRef CAS.
- S. Liu, D. Mei, L. Wang and X. Tu, Chem. Eng. J., 2017, 307, 793–802 CrossRef CAS.
- F. Saleem, J. Umer, A. Rehman, K. Zhang and A. Harvey, Energy Fuels, 2020, 34, 1744–1749 CrossRef CAS.
- E. Chiremba, K. Zhang, C. Kazak and G. Akay, AIChE J., 2017, 63, 4418–4429 CrossRef CAS.
- C. Xu and X. Tu, J. Energy Chem., 2013, 22, 420–425 CrossRef CAS.
- Q. Yu, M. Kong, T. Liu, J. Fei and X. Zheng, Plasma Chem. Plasma Process., 2012, 32, 153–163 CrossRef CAS.
- F. Saleem, J. Kennedy, U. H. Dahiru, K. Zhang and A. Harvey, Chem. Eng. Process., 2019, 142, 107557 CrossRef CAS.
|
This journal is © The Royal Society of Chemistry 2021 |