DOI:
10.1039/D1RA04758B
(Paper)
RSC Adv., 2021,
11, 29441-29452
New pyridine and chromene scaffolds as potent vasorelaxant and anticancer agents†
Received
19th June 2021
, Accepted 23rd August 2021
First published on 2nd September 2021
Abstract
Based on studies that have reported the association between cancer and cardiovascular diseases, new series of pyridine- (3a–o) and/or chromene- (4a–e) carbonitrile analogous were designed, synthesized and screened for their vasodilation and cytotoxic properties. The majority of the new chemical entities demonstrated significant vasodilation efficacies, compounds 3a, 3h, 3j, 3m, 3o, 4d and 4e exhibited the most promising potency with IC50 = 437.9, 481.0, 484.5, 444.8, 312.1, 427.6 and 417.2 μM, respectively, exceeding prazosin hydrochloride (IC50 = 487.3 μM). Compounds 3b–e, 3k and 3l also, revealed moderate vasodilation activity with IC50 values ranging from 489.7 to 584.5 μM. In addition, the anti-proliferative activity evaluation of the experimental compounds at 10 μM on the MCF-7 and MDA-MB 231 breast cancer cell lines illustrated the excellent anti-proliferative properties of derivatives 3d, 3g and 3i. Compound 3d was the most potent analogue with IC50 = 4.55 ± 0.88 and 9.87 ± 0.89 μM against MCF-7 and MDA-MB 231, respectively. Moreover, compound 3d stimulated apoptosis and cell cycle arrest at the S phase in MCF-7 cells in addition to its capability in accumulation of cells in pre-G1 phase and activating caspase-3. Furthermore, the molecular docking of 3d was performed to discover the binding modes within the active site of caspase-3. 3d, as the only common bi-functional agent among the tested hits, demonstrated that new pyridine-3-carbonitrile derivatives bearing cycloheptyl ring systems offer potential as new therapeutic candidates with combined vasodilation and anticancer properties.
1. Introduction
Two major leading causes of mortality worldwide are cardiovascular disturbances and cancer. For example, hypertension is one of the main causes of diseases that lead to human angio-cardiopathy death.1 Currently, based on statistics from the World Health Organization, 1.13 billion people worldwide are diagnosed with hypertension (WHO, 2020)2 which can contribute to the development of diabetes, renal failure, and heart diseases.2,3 Also, cancer was responsible for 10 million deaths in 2020 and despite the vast leap in cancer therapy, one from six deaths is due to cancer.4
Interestingly, multiple studies have recently hypothesized an association between hypertension, and cancer incidence and cancer mortality.5–8 For example, an epidemiological survey revealed that patients with cardiovascular disease have higher risks of developing cancer compared with the other population. Moreover, a meta-analysis of 13 prospective studies demonstrated that elevated blood pressure was related to a 7% higher breast cancer risk.9,10 As both diseases share common risk factors such as obesity, diabetes mellitus and alcoholism, this may clarify their synchronous occurrence.11 Indeed, this association termed as reverse cardio-oncology (cancer risk in patients with cardiovascular disease) is now an area of focus for both clinicians and basic scientists.12 Of particular relevance to our work is emerging evidence that cancer and cardiovascular diseases might share some biological pathways.5 However, to date, only few studies have focused on developing therapeutic agents that may offer dual potential for treating both cardiovascular disease and cancer, and hence addressing the therapeutic challenges of treating reverse cardio-oncology. Within this programme, we therefore aimed to identify, design and develop appropriate small molecules containing pharmacophores of potential interest for the treatment of cardiovascular disease and cancer, and hence for reverse cardio-oncology. At the same time, challenges associated with treating the individual diseases would also be addressed by identifying new lead compounds for development for application for each condition.
Numerous antihypertensive candidates have been developed to control high blood pressure such as potassium channel activators, calcium channel blockers, and angiotensin-converting enzyme inhibitors (ACEI). However, despite the progress in the newly developed targeted therapies, these treatment strategies mainly depend on vasodilation to reduce blood pressure.13 As a result, the drawbacks on systemic hypotension and limited efficiency are still challenging clinical problems, leading to poor prognosis.14 Thus, new antihypertensive therapeutics acting on a range of therapeutic targets are urgently needed to control hypertension more effectively and minimize adverse side-effects that are often associated with conventional agents.
A literature survey has reported that many pyridine derivatives play a vital role in vasorelaxation therapeutics.15 For example, milrinone and amrinone are positive inotropic agents with vasodilator properties; nifedipine is a peripheral and coronary vasodilator drug of the calcium channel blockers.15–17 Furthermore, different pyridine-3-carbonitrile analogs I, II and different nicotinate esters such as micinicate, hepronicate and inositol nicotinate have emerged as significant vasodilating agents (Fig. 1).18–22
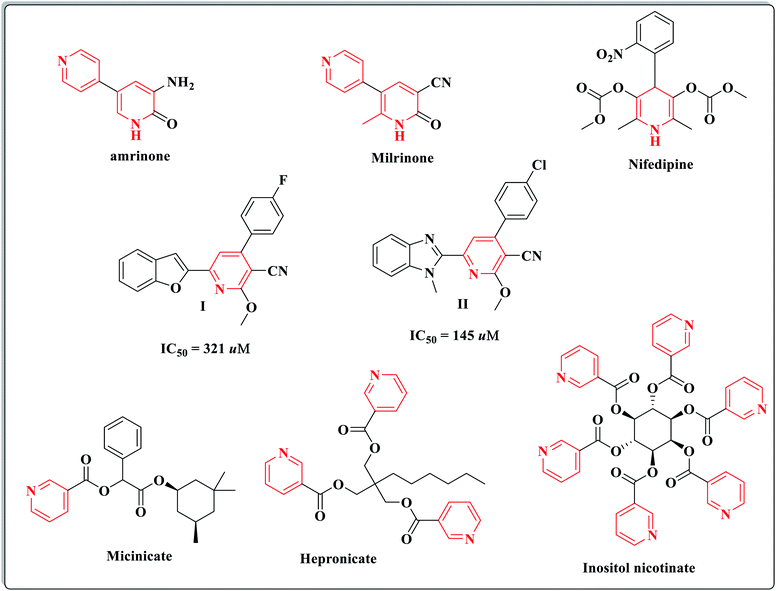 |
| Fig. 1 Different pyridine-based derivatives of potent vasodilation activity. | |
Oxygen heterocyclic compounds are frequently developed as potential therapeutic targets by medicinal chemists owing to their relatively low inherent toxicity.23 For example, different pyrano analogues such as compounds III, IV
24–27 as well as structural hybridization of scopoletin, a benzopyran-2-one derivative, with a benzopyran (chromene) nucleus, have led to the formation of substituted chromeno-coumarin hybrids of effective vasorelaxant activity. Moreover, glabridin bearing pyranobenzopyran core is a known vasorelaxing agent23 (Fig. 2).
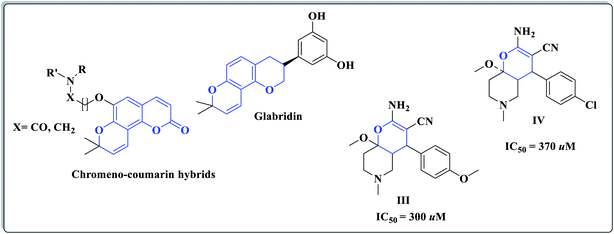 |
| Fig. 2 Various pyrano and chromeno derivatives of potent vasodilation activity. | |
In addition to their previously displayed vasodilation properties, pyridine- and/or chromene-based heterocycles have also demonstrated anticancer properties and potential as apoptotic inducers through caspase-3 activation28–32 (Fig. 3).
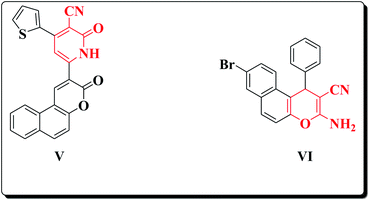 |
| Fig. 3 Pyridine and chromene based compounds as anticancer agents. | |
Encouraged by the observed effective vasorelaxant and anticancer activities of various analogues bearing pyridine as well as pyran and chromene rings, novel 2-methoxy-pyridine-3-carbonitriles and/or 2-aminopyran ring systems bearing various aromatic or heterocyclic moieties at C-4 and fused with different cycloalkane ring systems were identified for exploration in this study. The new chemical entities 3a–o and 4a–e were therefore identified as targets of interest herein for their potential as new and effective candidates for reverse cardio-oncology due to their dual vasodilation and anticancer functions.
2. Results and discussion
2.1. Chemistry
The reaction of ylidenemalononitriles 2a–m with cyclohexanone and/or cycloheptanone in the presence of sodium methoxide was utilized to accomplish a regioselective Michael addition reaction of the active methylene of 4-cycloalkanone at the β-carbon of arylidinemalononitriles 2a–m. The latter derivatives underwent subsequent cyclization (due to the methoxide attack at one of the nitrile groups), dehydration and then dehydrogenation to afford the corresponding 2-methoxy-4-aryl-6,7-dihydro-5H-cycloalkyl[b]pyridine-3-carbonitrile derivatives (3a–o). An alternative pathway has also been hypothesized to occur through a nucleophilic attack of the active methylene of cyclohexanone at the β-carbon of the unsaturated dinitrile function in the presence of the basic catalyst. Cyclization occurred as a result of the methoxide nucleophilic attack at 4-cyclohexanone carbonyl function, followed by interaction with the neighboring nitrile group.33 In this way, the corresponding 2-amino-4-(4-aryl)-8a-methoxy-4a,5,6,7,8,8a-hexahydro-4H-chromene-3-carbonitrile analogs (4a–e) were also afforded for analysis, as shown in Scheme 1.
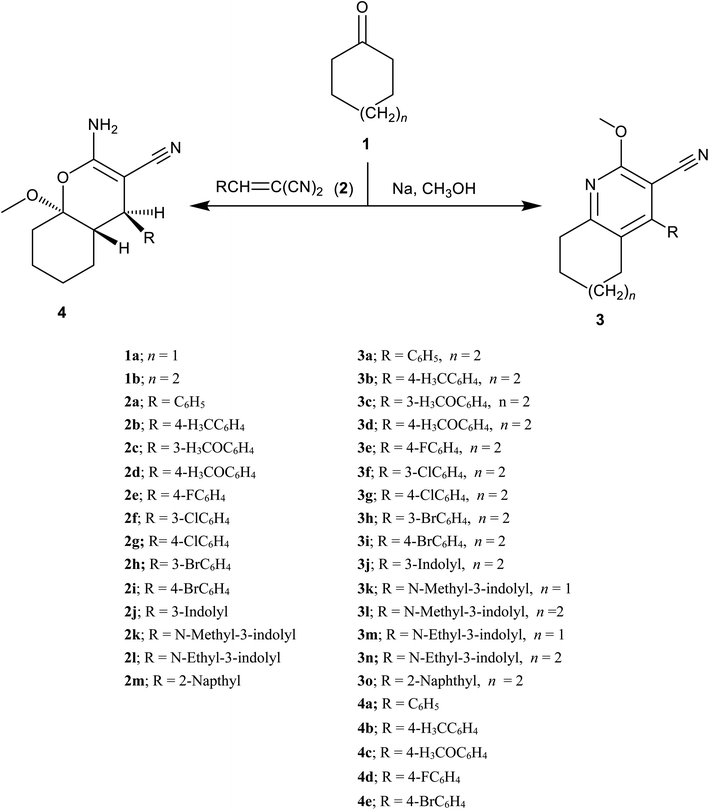 |
| Scheme 1 Synthetic routes for accessing the synthesized fused cycloalkane-pyridine-3-carbonitrile scaffolds 3a–o and 4a–e. | |
The derivatives (3a–o) and (4a–e) were characterized using a range of spectroscopic methods. For instance, compound 3a which is a representative member of the pyridine-3-carbonitrile series 3a–o, showed a strong stretching IR band at ν = 2220 cm−1 for (C
N) with no detection of the band representative of the carbonyl stretching vibration. The 1H-NMR spectrum also indicated the methylene protons H2C-6, H2C-7 and H2C-8 as multiplet at δH = 1.49–1.87 ppm while H2C-5 and H2C-9 were present as two triplets at δH = 2.53 and 3.04 ppm, respectively. The methoxy signal was resonating at δH = 4.05 ppm. The 13C-NMR spectrum for compound 3a showed the pyridinyl C-3 at δC = 93.73 ppm as well as the methoxy and nitrile carbons at δC = 54.13 and 115.57, respectively. EI mass spectrometric analysis demonstrated the parent ion peak m/z (%) 278 (M+, 66), 277 (100).
Considering compound 4a as a representative example for the chromene-3-carbonitrile analogs 4a–e, strong stretching vibrational bands were evident at ν = 3449, 3348 cm−1 for (NH2), and at ν = 2187 cm−1 for (C
N). Moreover, the 1H-NMR spectrum revealed the methoxy group at δH = 3.32 ppm, while the characteristic NH2 protons were assigned at δH = 4.36 ppm, the methylene functions H2C-5, H2C-6, H2C-7 and H2C-8 were resonating at δH = 1.07–1.87 ppm, as well as the methine protons HC-4a and HC-4 were observed as a multiplet at δH = 2.30–2.39 ppm and a broad singlet at δH = 3.29 ppm, respectively. The 13C-NMR spectrum for the same compound revealed the methylene carbons H2C-5, H2C-6, H2C-7 and H2C-8 at δC = 22.34, 25.06, 26.50, 30.86 ppm, respectively, the methine carbons HC-4 and HC-4a were detected at δC = 40.80 and 47.13 ppm, respectively. Also, the methoxy and the nitrile carbons were detected at δC = 48.65 and δC = 120.81 ppm, respectively, along with the characteristic quaternary carbon C-8a at δC = 102.76 ppm. EI mass spectrometric analysis revealed the relative intensity value of the parent ion peak m/z (%) 284 (M, 4.6), 112 (100). The established chemical structures of all of the new chemical entities were proven via spectral data (IR, 1H NMR, 13C NMR, MS) and exhibited similar features to those discussed for 3a and 4a (cf. Experimental section), the spectral charts (IR, 1H-, 13C-NMR and MS) of the new synthesized compounds are available in the ESI (Fig. S1–S79†).
2.2. Biological activity
2.2.1. Vasodilation properties. The novel substituted pyridine-/chromene-carbonitriles 3a–o and 4a–e, respectively, were tested for their vasodilation properties following a well-documented procedure using isolated thoracic aortic rings of mice pre-contracted with norepinephrine HCl. Prazosin hydrochloride was also included as a standard drug for comparison purposes. The observed results are displayed as IC50 values (Table 1) and dose response curves (Table S1 of ESI†). The obtained data shows that compounds 3a, 3h, 3j, 3m, 3o, 4d and 4e represented notable vasodilation efficiency with IC50 = 437.9, 481.0, 484.5, 444.8, 312.1, 427.6 and 417.2 μM, respectively, exceeding that of prazosin hydrochloride (IC50 = 487.3 μM). However, the tested analogous 3b–e, 3k and 3l exhibited modest vasodilation activity with IC50 values extent from 489.7 to 584.5 μM. On the other hand, derivatives 3f–g, 3i, 3n and 4a–c revealed weak vasodilation activity with IC50 range lies from 608.6 to 758.6 μM. It is obvious that the vasodilation activity is enhanced when cycloheptapyridine-3-carbonitrile scaffold is attached with 2-napthyl more than phenyl, substituted phenyl and/or 3-indolyl rings as shown in 3o, 3a, 3b, 3h and 3j, respectively. Likewise, the conjugation of chromene-3-carbonitrile group with phenyl rings of electron withdrawing substituents resulted in significant vasodilation efficiency as 4d and 4e. Thus the cycloalkane-pyridine-3-carbonitrile scaffolds incorporated with substituted aryl and/or heterocyclic rings might be developed into potent vasodilation candidates.
Table 1 Vasodilatory activity (IC50 μM) in rat thoracic aortic rings
Entry |
Compound |
R |
n |
Potency IC50 μM ± SDa |
SEM (n = 6) |
SD = standard deviation. |
1 |
3a |
C6H5 |
2 |
437.9 ± 8.57 |
3.50 |
2 |
3b |
4-H3CC6H4 |
2 |
489.7 ± 9.67 |
3.95 |
3 |
3c |
3-H3COC6H4 |
2 |
532.8 ± 10.45 |
4.27 |
4 |
3d |
4-H3COC6H4 |
2 |
524.1 ± 8.25 |
3.37 |
5 |
3e |
4-FC6H4 |
2 |
558.6 ± 12.88 |
5.26 |
6 |
3f |
3-ClC6H4 |
2 |
608.6 ± 16.65 |
6.80 |
7 |
3g |
4-ClC6H4 |
2 |
698.3 ± 11.65 |
4.75 |
8 |
3h |
3-BrC6H4 |
2 |
481.0 ± 13.39 |
5.47 |
9 |
3i |
4-BrC6H4 |
2 |
758.6 ± 13.00 |
5.31 |
10 |
3j |
3-Indolyl |
2 |
484.5 ± 15.02 |
6.13 |
11 |
3k |
N-Methyl-3-indolyl |
1 |
584.5 ± 12.70 |
5.19 |
12 |
3l |
N-Methyl-3-indolyl |
2 |
558.6 ± 11.20 |
4.57 |
13 |
3m |
N-Ethyl-3-indolyl |
1 |
444.8 ± 13.79 |
5.63 |
14 |
3n |
N-Ethyl-3-indolyl |
2 |
739.7 ± 11.67 |
4.76 |
15 |
3o |
2-Naphthyl |
2 |
312.1 ± 9.05 |
3.70 |
16 |
4a |
C6H5 |
1 |
710.2 ± 12.02 |
4.91 |
17 |
4b |
4-H3CC6H4 |
1 |
674.1 ± 11.57 |
4.72 |
18 |
4c |
4-H3COC6H4 |
1 |
639.7 ± 10.55 |
4.31 |
19 |
4d |
4-FC6H4 |
1 |
427.6 ± 8.26 |
3.37 |
20 |
4e |
4-BrC6H4 |
1 |
417.2 ± 9.35 |
3.82 |
21 |
Prazosin HCl |
— |
487.3 ± 10.13 |
4.14 |
2.2.2. Cytotoxic data of experimental compounds against cancer cells. The experimental compounds were also screened for their anti-proliferative activity at 10 μM against the MCF-7 and MDA-MB 231 breast cancer cell lines using the MTT assay. The results shown in Fig. 4 and 5 illustrate that whilst the majority of compounds appeared to have little to no effect on cell viability at this concentration, others such as 3d, 3g and 3i exhibited relatively good cytotoxic activity, with inhibition percentage around 50–60%. After initial screening, it was clearly noticed that the investigated derivatives demonstrated higher cytotoxic efficiency towards the MCF-7 cell line than the MDA-MB 231 cells. For example, compounds 3d, 3g and 3i revealed excellent cytotoxic efficiency against the MCF-7 cell line, with compound 3d being the most effective against the MDA-MB 231 cell line. The most active derivatives were selected for further study, in order to determine their IC50 values. As depicted in Table 2, compound 3d showed excellent cytotoxic efficacy on MCF-7 with IC50 = 4.55 ± 0.88 μM which illustrates greater activity than 5-fluouracil which is drug often used in chemotherapy regimens for breast cancer (IC50 = 7.10 ± 0.90 μM). Furthermore, compounds 3g and 3i also exhibited promising efficiency with IC50 = 11.52 ± 2.60 and 11.23 ± 3.27 μM, respectively. Moreover, compound 3d showed remarkable cytotoxic efficiency against MDA-MB 231 cells with IC50 = 9.87 ± 0.89 μM exceeding that of 5-fluouracil (IC50 = 15.10 ± 0.90 μM). It is noteworthy that compound 3d displayed significant activity against each of the two breast cancer cell lines. Hence, it was concluded that the inclusion of 4-methoxy-phenyl with cycloheptapyridine-3-carbonitrile fused system was beneficial for the cytotoxic efficiency against both types of breast cancer cell lines (Fig. 2 and 3 in ESI†). Alternatively, compounds 3d, 3g and 3i represented moderate vasodilation activity. More structural optimization is needed to reach to new candidates possessing dual vasodilation and anticancer activities.
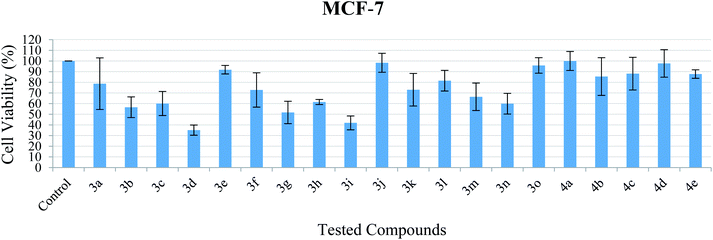 |
| Fig. 4 Cell viability of MCF-7 after exposure to the compounds at 10 μM. Data indicate mean ± SEM, n ≥ 3. | |
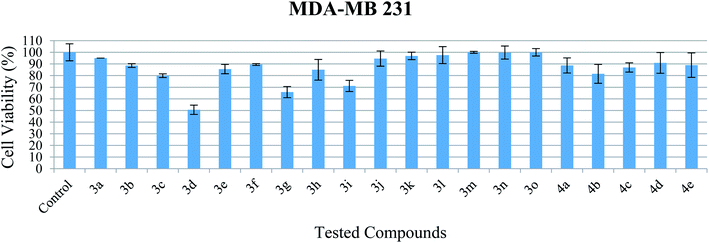 |
| Fig. 5 Cell viability of MDA-MB 231 after exposure to the compounds at 10 μM. Data indicate mean ± SEM, n ≥ 3. | |
Table 2 IC50 of tested compounds on MCF-7 and MDA-MB 231 cell lines, Data indicate mean IC50 ± SEM
Compound |
MCF-7, IC50 (μM) |
MDA-MB 231, IC50 (μM) |
3d |
4.55 ± 0.88 |
9.87 ± 0.89 |
3g |
11.52 ± 2.60 |
— |
3i |
11.23 ± 3.27 |
— |
5-FU |
7.10 ± 0.90 |
15.10 ± 0.90 |
2.2.3. Cellular mechanism of action.
2.2.3.1. Cell cycle arrest. Based on the prominent cytotoxic efficacy of compound 3d, it was elected for more in-depth investigation to study its influence on the cell cycle development and apoptosis induction in MCF-7 cells. Flow cytometry data revealed that subjecting MCF-7 cells to compound 3d at 4.55 μM for 24 h resulted in disruption of the normal cell cycle development. It was noticed that compound 3d caused a remarkable increment in the percentage of cells at the pre-G1 phase from 1.74 to 26.97% in addition to an increase in the cell percentages at the S phase from 39.27 to 49.33%. On the other hand, a reduction in cell percentages at the G1 phase (from 52.35 to 42.71%) and the G2-M phase (from 8.38 to 7.96%) were observed compared to the untreated MCF-7 cells (Fig. 6), Therefore, it could be hypothesized that compound 3d may suppress the proliferation of MCF-7 cells via induction of pre-G1 apoptosis and cell growth arrest at S phase.
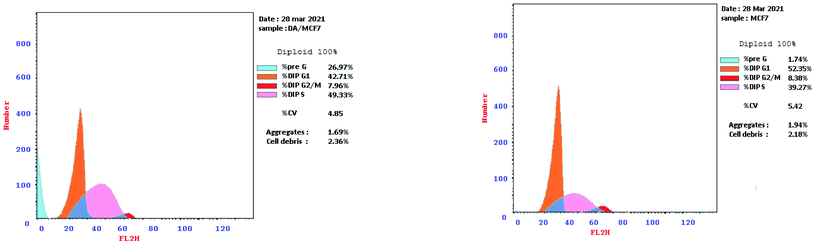 |
| Fig. 6 The impact of compound 3d on cell cycle progression of MCF-7 cells. | |
2.2.3.2. Detection of apoptosis via annexin-V-FITC assay. Annexin V-FITC/propidium iodide (PI) dual staining of MCF-7 cells was also performed to assure and quantify the apoptosis percentage prompted by compound 3d. A significant increase of apoptotic cells (late apoptosis) was detected upon treatment of MCF-7 cells with compound 3d from 0.22 to 13.73%, additionally, considerable increase in the early apoptosis was noticed from 0.57 to 3.89% compared to the untreated MCF-7 cells. Furthermore, there was a pronounced increment of the percentage of necrotic cells from 0.95 to 9.35% (Fig. 7). Thus the anti-proliferative activity of compound 3d was exerted via induction of apoptosis and necrosis.
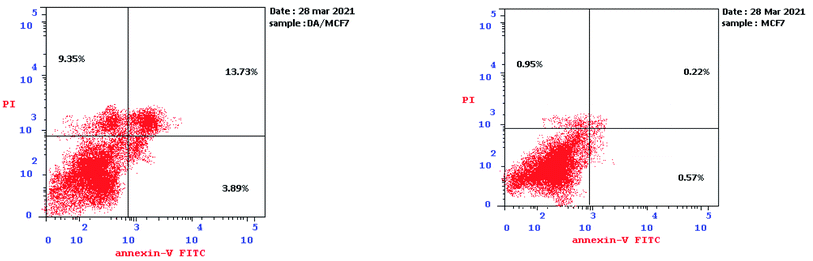 |
| Fig. 7 The influence of compound 3d in induction of apoptosis/necrosis of MCF-7 cells. | |
2.2.3.3. Upregulation of caspase-3. It has been reported that caspase cascade events are essential mediators in the initiation of apoptosis through intrinsic or extrinsic pathways.34,35 Therefore, the activation of caspase 3 has a crucial role in apoptotic cell death.36 A significant increase by 3.77 fold was noticed in the induction of caspase-3 upon treating of MCF-7 cells with compound 3d relative to the untreated MCF-7 cells (Table 3). Accordingly, it is hypothesized that the apoptotic process might be stimulated via activation of caspase-3.
Table 3 The influence of compound 3d on caspase-3 activity in MCF-7 cells
Compound |
Conc. (μM) |
RT-PCR, caspase-3, FLD |
3d |
4.55 |
3.77 |
Cont. MCF-7 |
— |
1 |
3. Molecular docking study on caspase-3
The above results illustrate the significant apoptotic activity of the target compound 3d that could be a result of activation of caspase-3. This was further probed through a molecular docking approach within the active binding site of caspase-3 kinase using Molecular Operating Environment (MOE-Dock) software version 2014.0901.37,38 Firstly, the crystal structure of the key enzyme caspase-3 (PDB code: 2J30)39 was retrieved from the RCSB Protein Data Bank and prepared for the docking process.
Docking of the promising activator 3d gave a perfect energy score −12.40 kcal mol−1 as illustrated in Fig. 8. The nitrogen of the cyano group formed H-bond acceptors with the sidechains of the key amino acids Arg64 and His121 (distance: 3.20 and 2.52 Å, respectively). Furthermore, the centroid of the pyridine scaffold linked to Arg207 through an arene–cation interaction. Interestingly, the cyclohepta[b]pyridine core fitted well within the binding site through hydrophobic interactions with the essential amino acids Tyr204, Ser205, Trp206, Ser120, Gln161, Cys163 and Phe256.
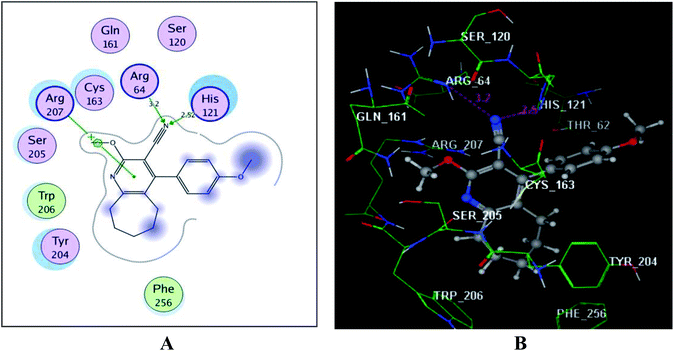 |
| Fig. 8 (A) & (B) diagrams illustrate 2D and 3D binding modes of the new derivative, 3d within the active site of caspase-3 (PDB: 2J30). | |
4. Conclusion
In this programme, we aimed to identify novel molecules with dual vasodilation and anti-cancer properties, in order to develop new opportunities for treating cardiovascular disease, cancer and reverse cardio-oncology. Reaction of ylidenemalononitriles 2a–m with cyclohexanone and/or cycloheptanone in the presence of sodium methoxide was carried out regioselectively due to a Michael addition reaction to afford the corresponding pyridine- (3a–o) and chromene- (4a–e) carbonitrile analogous, respectively. The new chemical entities were scanned for their vasodilation and cytotoxic properties. The majority of the tested analogous revealed significant vasodilation efficacy, compound 3o in particular was the most potent among the examined series with IC50 = 312.1 μM, which is superior to prazosin hydrochloride (IC50 = 487.3 μM). In addition, the anti-proliferative results at 10 μM on MCF-7 and MDA-MB 231 breast cancer cell lines, indicated excellent activity of the derivatives 3d, 3g and 3i. Compound 3d was the most active against the MCF-7 and MDA-MB 231 cell lines with IC50 = 4.55 ± 0.88 and 9.87 ± 0.89 μM, respectively. Furthermore, flow cytometry data revealed that compound 3d stimulated apoptosis of MCF-7 cancer cells via activation of caspase-3 and arrested the cell cycle at the S phase along with its ability in accumulation of cells at the pre-G1 phase. Moreover, molecular docking for compound 3d identified the interactions with key amino acids within the active binding site of caspase-3. Interestingly 3d exhibited both vasodilation and anti-proliferative activities, demonstrating that pyridine-3-carbonitrile scaffolds bearing cycloheptyl ring systems are important functionalized precursors for further development of new vasodilation and anticancer dual active hits.
5. Experimental
5.1. Chemistry
Melting points were recorded on a Stuart SMP30 melting point apparatus. IR spectra (KBr) were recorded on a JASCO 6100 spectrophotometer. NMR spectra were recorded on a JEOL AS 500 (1H: 500 MHz, 13C: 125 MHz) and a BRUKER 400 (1H: 400, 13C: 100 MHz) spectrometers. Mass spectra were recorded on a Shimadzu GCMS-QP 1000 EX (EI, 70 eV) spectrometer, Elemental microanalyses were recorded on a Vario El Elementar analyzer, ylidenemalononitrile 2a–m were prepared according to previously reported procedure.19–21
5.1.1. General procedure for synthesis of compounds. A mixture of equimolar amounts of 1a,b and the corresponding ylidenemalononitrile 2a–m (10 mmol) in methanol (25 mL) containing sodium (0.46 g, 20 mmol) was stirred at room temperature (20–25 °C) for 24 h. The separated solid was collected, washed with water and crystallized from an appropriate solvent affording 3a–o and/or 4a–e.
5.1.1.1. 2-Methoxy-4-phenyl-6,7,8,9-tetrahydro-5H-cyclohepta[b]pyridine-3-carbonitrile (3a). Colorless microcrystals from DMF; yield 1.65 g (59%); mp 214–215 °C; IR: νmax/cm−1 2220 (C
N), 1558 (C
N). 1H-NMR (400 MHz, CDCl3): δ 1.49–1.87 (m, 6H), 2.53 (t, J = 5.4, 2H), 3.04 (t, J = 5.4, 2H), 4.05 (s, 3H), 7.21–7.26 (m, 2H), 7.40–7.49 (m, 3H). 13C-NMR (100 MHz, CDCl3): δ 26.07, 27.63, 29.07, 32.07, 39.57, 54.13, 93.73, 115.57, 128.42, 128.62, 128.76, 129.23, 136.36, 154.97, 161.99, 166.60. MS: m/z (%) 278.09 (M+, 66), 277.03 (100). Anal. calcd for C18H18N2O (278.35): C, 77.67; H, 6.52; N, 10.06. Found: C, 77.80; H, 6.63; N, 10.16.
5.1.1.2. 2-Methoxy-4-p-tolyl-6,7,8,9-tetrahydro-5H-cyclohepta[b]pyridine-3-carbonitrile (3b). Colorless microcrystals from n-butanol; yield 1.67 g (57%); mp 228–230 °C; IR: νmax/cm−1 2221 (C
N), 1587 (C
N). 1H-NMR (400 MHz, CDCl3): δ 1.49–1.87 (m, 6H), 2.42 (s, 3H), 2.55 (t, J = 5.6, 2H), 3.03 (t, J = 5.4, 2H), 4.04 (s, 3H), 7.10 (d, J = 8 Hz, 2H), 7.26 (d, J = 8.8 Hz, 2H). 13C-NMR (100 MHz, CDCl3): δ 21.32, 26.09, 27.65, 29.05, 32.09, 39.57, 54.08, 93.83, 115.76, 128.35, 129.29, 129.30, 133.38, 138.65, 155.15, 162.00, 166.47. MS: m/z (%) 292.10 (M+, 75), 290.87 (100). Anal. calcd for C19H20N2O (292.37): C, 78.05; H, 6.89; N, 9.58. Found: C, 78.17; H, 6.93; N, 9.70.
5.1.1.3. 2-Methoxy-4-(3-methoxy-phenyl)-6,7,8,9-tetrahydro-5H-cyclohepta[b]pyridine-3-carbonitrile (3c). Pale yellow microcrystals from n-butanol; yield 1.82 g (59%); mp 171–172 °C; IR: νmax/cm−1 2219 (C
N), 1635 (C
N). 1H-NMR (400 MHz, CDCl3): δ 1.49–1.87 (m, 6H), 2.53 (t, J = 5.2 Hz, 2H), 3.02 (t, J = 5.8 Hz, 2H), 3.86 (s, 3H), 4.05 (s, 3H), 6.73–6.80 (m, 2H), 6.96 (d, J = 10.8 Hz, 1H), 7.36 (t, J = 8 Hz, 1H). 13C-NMR (100 MHz, CDCl3): δ 26.07, 27.66, 29.10, 32.07, 39.55, 54.13, 55.30, 93.65, 114.08, 114.30, 115.50, 120.71, 129.19, 129.79, 137.61, 154.79, 159.53, 161.96, 166.58. MS: m/z (%) 308.08 (M+, 100). Anal. calcd for C19H20N2O2 (308.37): C, 74.00; H, 6.54; N, 9.08. Found: C, 74.24; H, 6.40; N, 8.90.
5.1.1.4. 2-Methoxy-4-(4-methoxy-phenyl)-6,7,8,9-tetrahydro-5H-cyclohepta[b]pyridine-3-carbonitrile (3d). Colorless microcrystals from ethanol; yield 1.92 g (62%); mp 167–168 °C; IR: νmax/cm−1 2217 (C
N), 1608 (C
N). 1H-NMR (400 MHz, CDCl3): δ 1.50–1.87 (m, 6H), 2.56 (t, J = 5.4 Hz, 2H), 3.03 (t, J = 5.6 Hz, 2H), 3.85 (s, 3H), 4.04 (s, 3H), 6.98 (d, J = 8.4 Hz, 2H), 7.15 (d, J = 8.4 Hz, 2H). 13C-NMR (100 MHz, CDCl3): δ 26.09, 27.66, 29.02, 32.09, 39.58, 54.08, 55.28, 93.92, 114.05, 114.38, 115.86, 128.48, 129.48, 129.89, 130.20, 154.82, 159.89, 162.05, 166.46. MS: m/z (%) 308.11 (M+, 100). Anal. calcd for C19H20N2O2 (308.37): C, 74.00; H, 6.54; N, 9.08. Found: C, 74.17; H, 6.63; N, 9.00.
5.1.1.5. 4-(4-Fluoro-phenyl)-2-methoxy-6,7,8,9-tetrahydro-5H-cyclohepta[b]pyridine-3-carbonitrile (3e). Colorless microcrystals from n-butanol; yield 1.78 g (60%); mp 235–237 °C; IR: νmax/cm−1 2223 (C
N), 1560 (C
N). 1H-NMR (400 MHz, CDCl3): δ 1.49–1.88 (m, 6H), 2.52 (t, J = 5.6 Hz, 2H), 3.04 (t, J = 5.6 Hz, 2H), 4.05 (s, 3H), 7.14–7.26 (m, 4H). 13C-NMR (100 MHz, CDCl3): δ 26.03, 27.59, 29.04, 32.03, 39.57, 54.17, 93.80, 115.50, 115.94, 129.33, 130.33, 130.41, 132.23, 132.27, 153.87, 162.02, 164.14, 166.79. MS: m/z (%) 296.10 (M+, 68), 295.03 (100). Anal. calcd for C18H17FN2O (296.34): C, 72.95; H, 5.78; N, 9.45. Found: C, 72.80; H, 5.89; N, 9.60.
5.1.1.6. 4-(3-Chloro-phenyl)-2-methoxy-6,7,8,9-tetrahydro-5H-cyclohepta[b]pyridine-3-carbonitrile (3f). Colorless microcrystals from n-butanol; yield 1.88 g (60%); mp 174–176 °C; IR: νmax/cm−1 2219 (C
N), 1633 (C
N). 1H-NMR (400 MHz, CDCl3): δ 1.49–1.88 (m, 6H), 2.52 (t, J = 5.6 Hz, 2H), 3.04 (t, J = 5.6 Hz, 2H), 4.05 (s, 3H), 7.11 (d, J = 10 Hz, 1H), 7.21 (s, 1H), 7.39–7.44 (m, 2H). 13C-NMR (100 MHz, CDCl3): δ 26.01, 27.56, 29.13, 31.99, 39.57, 54.22, 93.52, 115.22, 126.69, 128.47, 129.03, 129.11, 130.06, 134.62, 138.05, 153.23, 161.99, 166.95. MS: m/z (%) 312.04 (M+, 59), 311.01 (100). Anal. calcd for C18H17ClN2O (312.79): C, 69.12; H, 5.48; N, 8.96. Found: C, 69.00; H, 5.30; N, 9.10.
5.1.1.7. 4-(4-Chloro-phenyl)-2-methoxy-6,7,8,9-tetrahydro-5H-cyclohepta[b]pyridine-3-carbonitrile (3g). Colorless microcrystals from n-butanol; yield 1.94 g (62%); mp 229–231 °C; IR: νmax/cm−1 2221 (C
N), 1557 (C
N). 1H-NMR (400 MHz, CDCl3): δ 1.48–1.88 (m, 6H), 2.52 (t, J = 5.6 Hz, 2H), 3.04 (t, J = 5.8 Hz, 2H), 4.05 (s, 3H), 7.16 (d, J = 8.4 Hz, 2H), 7.44 (d, J = 8.4 Hz, 2H). 13C-NMR (100 MHz, CDCl3): δ 26.02, 27.58, 29.07, 32.01, 39.57, 54.20, 93.57, 115.41, 129.00, 129.16, 129.87, 134.71, 135.04, 153.62, 162.03, 166.90. MS: m/z (%) 312.11 (M+, 79), 311.06 (100). Anal. calcd for C18H17ClN2O (312.79): C, 69.12; H, 5.48; N, 8.96. Found: C, 69.30; H, 5.59; N, 8.90.
5.1.1.8. 4-(3-Bromo-phenyl)-2-methoxy-6,7,8,9-tetrahydro-5H-cyclohepta[b]pyridine-3-carbonitrile (3h). Colorless microcrystals from n-butanol; yield 2.22 g (62%); mp 167–168 °C; IR: νmax/cm−1 2221 (C
N), 1635 (C
N). 1H-NMR (400 MHz, CDCl3): δ 1.51–1.88 (m, 6H), 2.52 (t, J = 5.6 Hz, 2H), 3.04 (t, J = 5.4 Hz, 2H), 4.05 (s, 3H), 7.16 (d, J = 8.8 Hz, 1H), 7.34–7.38 (m, 2H), 7.57 (d, J = 8.8 Hz, 1H). 13C-NMR (100 MHz, CDCl3): δ 26.01, 27.54, 29.14, 31.99, 39.57, 54.22, 93.54, 115.22, 122.68, 127.13, 129.10, 130.28, 131.30, 131.96, 138.30, 153.12, 162.00, 166.94. MS: m/z (%) 356.94 (M+, 100). Anal. calcd for C18H17BrN2O (357.24): C, 60.52; H, 4.80; N, 7.84. Found: C, 60.74; H, 4.63; N, 7.76.
5.1.1.9. 4-(4-Bromo-phenyl)-2-methoxy-6,7,8,9-tetrahydro-5H-cyclohepta[b]pyridine-3-carbonitrile (3i). Colorless microcrystals from n-butanol; yield 2.29 g (64%); mp 235–237 °C; IR: νmax/cm−1 2222 (C
N), 1557 (C
N). 1H-NMR (400 MHz, CDCl3): δ 1.48–1.88 (m, 6H), 2.52 (t, J = 5.6 Hz, 2H), 3.04 (t, J = 5.8 Hz, 2H), 4.05 (s, 3H), 7.09 (d, J = 8.8 Hz, 2H), 7.60 (d, J = 8.8 Hz, 2H). 13C-NMR (100 MHz, CDCl3): δ 26.02, 27.58, 29.08, 32.01, 39.56, 54.21, 93.49, 115.39, 123.27, 129.08, 130.13, 131.95, 135.19, 153.61, 162.03, 166.92. MS: m/z (%) 356.93 (M+, 100). Anal. calcd for C18H17BrN2O (357.24): C, 60.52; H, 4.80; N, 7.84. Found: C, 60.41; H, 4.93; N, 7.96.
5.1.1.10. 4-(1H-Indol-3-yl)-2-methoxy-6,7,8,9-tetrahydro-5H-cyclohepta[b]pyridine-3-carbonitrile (3j). Colorless microcrystals from n-butanol; yield 2.00 g (63%); mp 244–246 °C; IR: νmax/cm−1 3438 (NH), 2217 (C
N), 1635 (C
N). 1H-NMR (400 MHz, CDCl3): δ 1.38–1.84 (m, 6H), 2.66 (t, J = 3.6 Hz, 2H), 3.01–3.13 (m, 2H), 4.07 (s, 3H), 7.13 (t, J = 7.2 Hz, 1H), 7.24–7.34 (m, 3H), 7.44 (d, J = 7.6 Hz, 1H), 8.48 (s, 1H). 13C-NMR (100 MHz, CDCl3): δ 26.09, 27.90, 29.49, 32.21, 39.81, 54.06, 94.86, 111.58, 112.13, 116.42, 119.56, 120.59, 122.78, 124.47, 126.39, 130.96, 135.82, 148.44, 162.39, 166.13. MS: m/z (%) 317.24 (M+, 100). Anal. calcd for C20H19N3O (317.38): C, 75.69; H, 6.03; N, 13.24. Found: C, 75.51; H, 6.20; N, 13.30.
5.1.1.11. 2-Methoxy-4-(1-methyl-1H-indol-3-yl)-5,6,7,8-tetrahydro-quinoline-3-carbonitrile (3k). Yellow microcrystals from ethanol; yield 1.94 g (61%); mp 214–216 °C; IR: νmax/cm−1 2215 (C
N), 1632 (C
N). 1H-NMR (500 MHz, CDCl3): δ 1.51–2.94 (m, 8H), 3.81 (s, 3H), 4.04 (s, 3H), 7.09–7.42 (m, 5H). 13C-NMR (125 MHz, CDCl3): δ 22.75, 22.90, 26.90, 33.25, 33.52, 54.17, 95.02, 109.94, 110.02, 116.44, 120.24, 122.39, 125.22, 126.35, 128.89, 137.02, 150.10, 159.76, 162.36. MS: m/z (%) 317.16 (M+, 100). Anal. calcd for C20H19N3O (317.38): C, 75.69; H, 6.03; N, 13.24. Found: C, 75.84; H, 6.19; N, 13.15.
5.1.1.12. 2-Methoxy-4-(1-methyl-1H-indol-3-yl)-6,7,8,9-tetrahydro-5H-cyclohepta[b]pyridine-3-carbonitrile (3l). Yellow microcrystals from n-butanol; yield 2.09 g (63%); mp 217–219 °C; IR: νmax/cm−1 2221 (C
N), 1614 (C
N). 1H-NMR (400 MHz, CDCl3): δ 1.59–1.85 (m, 6H), 2.67–2.73 (m, 2H), 3.00–3.13 (m, 2H), 3.87 (s, 3H), 4.06 (s, 3H), 7.13–7.17 (m, 2H), 7.26–7.30 (m, 2H), 7.38 (d, J = 8.8 Hz, 1H). 13C-NMR (100 MHz, CDCl3): δ 26.10, 27.94, 29.49, 32.22, 33.14, 39.83, 54.03, 94.75, 109.73, 110.46, 116.45, 119.75, 120.18, 122.29, 126.91, 128.94, 130.82, 136.78, 148.43, 162.42, 166.00. MS: m/z (%) 331.24 (M+, 100). Anal. calcd for C21H21N3O (331.41): C, 76.11; H, 6.39; N, 12.68. Found: C, 76.00; H, 6.57; N, 12.56.
5.1.1.13. 4-(1-Ethyl-1H-indol-3-yl)-2-methoxy-5,6,7,8-tetrahydro-quinoline-3-carbonitrile (3m). Colorless microcrystals from ethanol; yield 2.13 g (64%); mp 204–206 °C; IR: νmax/cm−1 2217 (C
N), 1617 (C
N). 1H-NMR (500 MHz, CDCl3): δ 1.53 (t, J = 7.2 Hz, 3H), 1.73–2.95 (m, 8H), 4.04 (s, 3H), 4.22 (q, J = 7.3 Hz, 2H), 7.10–7.41 (m, 5H). 13C-NMR (125 MHz, CDCl3): δ 15.57, 22.77, 22.93, 26.92, 33.52, 41.43, 54.16, 95.02, 110.03, 110.10, 116.22, 120.20, 120.38, 122.23, 125.20, 126.52, 127.38, 136.10, 150.27, 159.71, 162.38. MS: m/z (%) 331.11 (M+, 100). Anal. calcd for C21H21N3O (331.41): C, 76.11; H, 6.39; N, 12.68. Found: C, 76.30; H, 6.29; N, 12.76.
5.1.1.14. 4-(1-Ethyl-1H-indol-3-yl)-2-methoxy-6,7,8,9-tetrahydro-5H-cyclohepta[b]pyridine-3-carbonitrile (3n). Colorless microcrystals from n-butanol; yield 2.25 g (65%); mp 192–194 °C; IR: νmax/cm−1 2221 (C
N),1560 (C
N). 1H-NMR (400 MHz, CDCl3): δ 1.52 (t, J = 7.2 Hz, 3H), 1.60–1.85 (m, 6H), 2.68–2.71 (m, 2H), 2.99–3.12 (m, 2H), 4.06 (s, 3H), 4.22 (q, J = 7.7 Hz, 2H), 7.12–7.16 (m, 1H), 7.25–7.31 (m, 3H), 7.40 (d, J = 8.4 Hz, 1H). 13C-NMR (100 MHz, CDCl3): δ 15.45, 26.11, 27.96, 29.53, 32.22, 39.84, 41.30, 54.03, 94.77, 109.81, 110.53, 116.44, 119.88, 120.12, 122.12, 127.07, 127.46, 130.77, 135.84, 148.60, 162.43, 165.95. MS: m/z (%) 345.23 (M+, 100). Anal. calcd for C22H23N3O (345.44): C, 76.49; H, 6.71; N, 12.16. Found: C, 76.70; H, 6.63; N, 12.06.
5.1.1.15. 2-Methoxy-4-naphthalen-1-yl-6,7,8,9-tetrahydro-5H-cyclohepta[b]pyridine-3-carbonitrile (3o). Colorless microcrystals from n-butanol; yield 2.20 g (67%); mp 182–184 °C; IR: νmax/cm−1 2223 (C
N), 1633 (C
N). 1H-NMR (400 MHz, CDCl3): δ 1.25–1.80 (m, 6H), 2.31 (t, J = 5.6 Hz, 2H), 3.08 (t, J = 7.6 Hz, 2H), 4.09 (s, 3H), 7.26–7.58 (m, 5H), 7.91 (t, J = 8.2 Hz, 2H). 13C-NMR (100 MHz, CDCl3): δ 26.09, 27.47, 29.40, 32.09, 39.70, 54.17, 94.73, 115.19, 124.89, 125.32, 126.30, 126.33, 126.75, 128.57, 129.24, 130.45, 130.84, 133.48, 133.98, 153.76, 162.09, 166.45. MS: m/z (%) 328.06 (M+, 100). Anal. calcd for C22H20N2O (328.41): C, 80.46; H, 6.14; N, 8.53. Found: C, 80.60; H, 6.03; N, 8.48.
5.1.1.16. 2-Amino-8a-methoxy-4-phenyl-4a,5,6,7,8,8a-hexahydro-4H-chromene-3-carbonitrile (4a). Colorless microcrystals from ethanol; yield 1.54 g (54%); mp 188–190 °C; IR: νmax/cm−1 3449, 3348 (NH2), 2187 (C
N). 1H-NMR (400 MHz, CDCl3): δ 1.07–1.87 (m, 8H), 2.30–2.39 (m, 1H), 3.29 (br. s, 1H), 3.32 (s, 3H), 4.36 (s, 2H), 7.19–7.49 (m, 5H). 13C-NMR (100 MHz, CDCl3): δ 22.34, 25.06, 26.50, 30.86, 40.80, 47.13, 48.65, 62.03, 102.76, 120.81, 127.10, 128.09, 128.48, 128.69, 128.90, 141.36, 160.69. MS: m/z (%) 284.10 (M+, 4.6), 112.02 (100). Anal. calcd for C17H20N2O2 (284.35): C, 71.81; H, 7.09; N, 9.85. Found: C, 71.99; H, 7.25; N, 9.77.
5.1.1.17. 2-Amino-8a-methoxy-4-p-tolyl-4a,5,6,7,8,8a-hexahydro-4H-chromene-3-carbonitrile (4b). Pale yellow microcrystals from ethanol; yield 1.65 g (55%); mp 201–203 °C; IR: νmax/cm−1 3449, 3348 (NH2), 2184 (C
N). 1H-NMR (400 MHz, CDCl3): δ 1.09–1.89 (m, 8H), 2.16 (d, J = 11.2 Hz, 1H), 2.34 (s, 3H), 3.24 (br. s, 1H), 3.32 (s, 3H), 4.40 (s, 2H), 7.07–7.52 (m, 4H). 13C-NMR (100 MHz, CDCl3): δ 21.09, 22.36, 25.09, 26.52, 30.87, 40.35, 47.12, 48.63, 62.15, 102.76, 120.94, 128.42, 129.20, 136.55, 138.27, 160.64. MS: m/z (%) 298.10 (M+, 14), 112.03 (100). Anal. calcd for C18H22N2O2 (298.38): C, 72.46; H, 7.43; N, 9.39. Found: C, 72.30; H, 7.58; N, 9.20.
5.1.1.18. 2-Amino-8a-methoxy-4-(4-methoxy-phenyl)-4a,5,6,7,8,8a-hexahydro-4H-chromene-3-carbonitrile (4c). Yellow microcrystals from ethanol; yield 1.89 g (60%); mp 203–204 °C; IR: νmax/cm−1 3446, 3348 (NH2), 2183 (C
N). 1H-NMR (500 MHz, CDCl3): 1.05–1.67 (m, 8H), 2.28 (d, J = 10.5 Hz, 1H), 3.23 (d, J = 10.5 Hz, 1H), 3.30 (s, 3H), 3.78 (s, 3H), 4.37 (s, 2H), 6.83 (d, J = 8.6 Hz, 2H), 7.09 (d, J = 8.6 Hz, 2H). 13C-NMR (125 MHz, CDCl3): δ 22.46, 25.18, 26.59, 30.94, 40.03, 47.30, 48.74, 55.28, 62.20, 102.87, 113.98, 121.15, 129.56, 133.42, 158.67, 160.77. MS: m/z (%) 314.25 (M+, 15), 112.12 (100). Anal. calcd for C18H22N2O3 (314.38): C, 68.77; H, 7.05; N, 8.91. Found: C, 68.60; H, 7.18; N, 8.80.
5.1.1.19. 2-Amino-4-(4-fluoro-phenyl)-8a-methoxy-4a,5,6,7,8,8a-hexahydro-4H-chromene-3-carbonitrile (4d). Pale yellow microcrystals from ethanol; yield 1.88 g (62%); mp 208–210 °C; IR: νmax/cm−1 3442, 3338, (NH2), 2186 (C
N). 1H-NMR (500 MHz, CDCl3): 1.07–1.65 (m, 8H), 2.29 (d, J = 11.4 Hz, 1H), 3.31 (br s, 4H), 4.38 (d, J = 10.5 Hz, 2H), 6.90–7.30 (m, 4H). 13C-NMR (125 MHz, CDCl3): δ 22.41, 25.12, 26.55, 30.92, 40.24, 47.33, 48.77, 61.68, 102.84, 115.36, 115.54, 115.93, 116.10, 120.91, 130.06, 137.18, 160.91. MS: m/z (%) 302.07 (M+, 38), 112.05 (100). Anal. calcd for C17H19FN2O2 (302.34): C, 67.53; H, 6.33; N, 9.27. Found: C, 67.40; H, 6.49; N, 9.17.
5.1.1.20. 2-Amino-4-(4-bromo-phenyl)-8a-methoxy-4a,5,6,7,8,8a-hexahydro-4H-chromene-3-carbonitrile (4e). Yellow microcrystals from ethanol; yield 2.00 g (55%); mp 205–207 °C; IR: νmax/cm−1 3448, 3319 (NH2), 2188 (C
N). 1H-NMR (400 MHz, CDCl3): 1.05–1.65 (m, 8H), 2.30 (d, J = 11.4 Hz, 1H), 3.27 (d, J = 10.8 Hz, 1H), 3.30 (s, 3H), 4.45 (s, 2H), 7.06 (d, J = 8.4 Hz, 2H), 7.42 (d, J = 8.4 Hz, 2H). 13C-NMR (100 MHz, CDCl3): δ 22.28, 25.01, 26.44, 30.81, 40.43, 47.09, 48.70, 61.21, 102.73, 120.68, 120.89, 130.29, 131.64, 140.56, 160.90. MS: m/z (%) 363.16 (M+, 6), 112.08 (100). Anal. calcd for C17H19BrN2O2 (363.25): C, 56.21; H, 5.27; N, 7.71. Found: C, 56.40; H, 5.19; N, 7.87.
5.2. Vasodilation activity screening
Vasodilation properties were assessed using well documented techniques19–21 such that the effects of the newly synthesized pyridine-3-carbonitriles (3a–o) and chromene-3-carbonitrile (4a–e) were determined on isolated thoracic aortic rings of male Wister rats (250–350 g). Further details are available in the ESI.†
5.3. In vitro antitumor screening
Compounds 3a–o and 4a–e were screened against two human cancer cell lines for anti-tumor activity using a cell based approach. The cell line MCF-7 (breast cancer cell line) was obtained from Tenovus centre for cancer research (Cardiff, UK), MDA-MB 231 (epithelial, human breast cancer cell line) was purchased from the European collection of cell cultures (ECACC). The cell culture medium EMEM, fetal bovine serum (FBS), L-glutamine (200 mM), non-essential amino acid (NEAA) [10X], and trypsin EDTA were purchased from Lonza (UK). The cell culture medium RPMI 1640 (with L-glutamine), DMEM (without L-glutamine), DMEM (low glucose, with glutamax), and dimethyl sulfoxide (DMSO) were purchased from Fisher scientific (UK).
3-(4,5-Dimethylthiazol-2-yl)-2,5-diphenyltetrazolium bromide salt was purchased from Sigma-Aldrich (UK).
The cell culture method and MTT cell viability assay used is briefly displayed in ESI.†
5.4. Cell cycle analysis and apoptosis detection
The cell cycle analysis and apoptosis investigations were carried out by flow cytometry.40 MCF-7 cells were seeded at 8 × 104 and incubated at 37 °C, 5% CO2 overnight. After treatment with the tested compound, for 24 h, cell pellets were collected and centrifuged (300g, 5 min). For cell cycle analysis, cell pellets were fixed with 70% ethanol on ice for 15 min and collected again. The collected pellets were incubated with propidium iodide (PI) staining solution (50 mg mL−1 PI, 0.1 mg mL−1 RNaseA, 0.05% Triton X-100) at room temperature for 1 hand analyzed by Gallios flow cytometer (Beckman Coulter, Brea, CA, USA). Apoptosis detection was performed by FITC Annexin V/PI commercial kit (Becton Dickenson, Franklin Lakes, NJ, USA) following the manufacture protocol. The samples were analyzed by fluorescence-activated cell sorting (FACS) with a Gallios flow cytometer (Beckman Coulter, Brea, CA, USA) within 1 h after staining. Data were analyzed using Kaluzav 1.2 (Beckman Coulter).
5.5. Caspase-3 activation assay
The activity of caspase-3 was determined using MaxDiscovery™ caspase-3 colorimetric detection kit, Bioo Scientific Corporation (BIOO), USA. Briefly, MCF-7 cells (5 × 105 cells per well) in a 6-well plate were treated with IC50 concentrations of compound 3d for 24 h. The cell culture medium was gently removed from the control and the treated culture wells, and then the cells were lysed by adding cell lysis buffer (1000 μL) to each culture well. The plate was gently shaken for 10 min to facilitate cell lysis and sample homogenization. Caspase-3 substrate (100 μL) was diluted into 10 mL of reaction buffer. In the reaction microplate, 100 μL caspase-3 reaction buffer was added to each well, followed by 100 μL of cell lysate. The absorbance was measured using a plate reader for the increase in absorbance at 405 nm in 30 min. Caspase-3 activity was expressed as the change of the activity in treated cancer cells compared to the untreated controls.41,42
5.6. Molecular docking study
The docking studies were performed using Molecular Operating Environment (MOE-Dock) software version 2014.0901.37,38 The co-crystallized structure of caspase-3 (PDB code: 2J30)39 was downloaded from the RCSB Protein Data Bank. All minimizations for the structure were performed with MOE until an RMSD gradient of 0.05 kcal mol−1 Å−1 with MMFF94x force field and the partial charges were automatically detected. Preparation of caspase-3 kinase structure was achieved using Protonate 3D protocol in MOE with the default options. The docking protocol was achieved using London dG scoring function and Triangle Matcher placement method.
Conflicts of interest
The authors declare no conflicts of interest.
References
- L. Hu, L. Li, Q. Chang, S. Fu, J. Qin, Z. Chen, X. Li, Q. Liu, G. Hu and Q. Li, Discovery of Novel Pyrazolo[3,4-b] pyridine Derivatives with Dual Activities of Vascular Remodeling Inhibition and Vasodilation for the Treatment of Pulmonary Arterial Hypertension, J. Med. Chem., 2020, 63, 11215–11234 CrossRef CAS PubMed.
- S. Estrada-Soto, M. E. González-Trujano, P. Rendón-Vallejo, L. Arias-Durán, G. Avila-Villarreal and R. Villalobos-Molina, Antihypertensive and vasorelaxant mode of action of the ethanol-soluble extract from Tagetes lucida Cav. aerial parts and its main bioactive metabolites, J. Ethnopharmacol., 2021, 266, 113399 CrossRef CAS PubMed.
- L. Li, W. Zhang, F. Lin, X. Lu, W. Chen, X. Li, X. Zhou, R. Su, L. Wang, Z. Zheng and S. Li, Synthesis and biological evaluation of pyrazolo[3,4-b]pyridine-3-yl pyrimidine derivatives as sGC stimulators for the treatment of pulmonary hypertension, Eur. J. Med. Chem., 2019, 173, 107–116 CrossRef CAS PubMed.
- J. Ferlay, M. Laversanne, M. Ervik, F. Lam, M. Colombet, L. Mery, M. Pineros, A. Znaor, I. Soerjomataram and F. Bray, Global Cancer Observatory: Cancer Today, International Agency for Research on Cancer, Lyon, 2020 Search PubMed.
- J. P. Aboumsallem, J. Moslehi and R. A. de Boer, Reverse Cardio-Oncology: Cancer Development in Patients with Cardiovascular Disease, J. Am. Heart Assoc., 2020, 9, e013754 Search PubMed.
- T. Stocks, M. Van Hemelrijck, J. Manjer, T. Bjorge, H. Ulmer, G. Hallmans, B. Lindkvist, R. Selmer, G. Nagel, S. Tretli, H. Concin, A. Engeland, H. Jonsson and P. Stattin, Blood pressure and risk of cancer incidence and mortality in the Metabolic Syndrome and Cancer Project, Hypertension, 2012, 59, 802–810 CrossRef CAS PubMed.
- T. Hasin, Z. Iakobishvili and G. Weisz, Associated risk of malignancy in patients with cardiovascular disease: evidence and possible mechanism, Am. J. Med., 2017, 130, 780–785 CrossRef PubMed.
- J. J. Moslehi, Cardiovascular toxic effects of targeted cancer therapies, N. Engl. J. Med., 2016, 375, 1457–1467 CrossRef CAS PubMed.
- A. Seretis, S. Cividini, G. Markozannes, X. Tseretopoulou, D. S. Lopez, E. E. Ntzani and K. K. Tsilidis, Association between blood pressure and risk of cancer development: a systematic review and meta-analysis of observational studies, Sci. Rep., 2019, 9, 8565 CrossRef PubMed.
- H. Han, W. Guo, W. Shi, Y. Yu, Y. Zhang, X. Ye and J. He, Hypertension and breast cancer risk: a systematic review and meta-analysis, Sci. Rep., 2017, 7, 44877 CrossRef CAS PubMed.
- W. C. Meijers and R. A. de Boer, Common risk factors for heart failure and cancer, Cardiovasc. Res., 2019, 115, 844–853 CrossRef CAS PubMed.
- J. S. Coviello, Cardiovascular and cancer risk: the role of cardio-oncology, J. Adv. Pract. Oncol., 2018, 9, 160–176 Search PubMed.
- M. Zakaria Stiti, M. Belghobsi, T. Habila, E. Goffin, P. d Tullio, B. Pirotte, G. Faury and S. Khelili, Synthesis and vasodilator activity of new 1,4-dihyropyridines bearing sulfonylurea, urea and thiourea moieties, Chem. Pap., 2020, 74, 915–928 CrossRef.
- T. Nakai, N. R. Perl, T. C. Barden, A. Carvalho, A. Fretzen, P. Germano, G. Y. Im, H. Jin, C. Kim, T. W. Lee, L. Long, J. Moore, J. M. Rohde, R. Sarno, C. Segal, E. O. Solberg, J. Tobin, D. P. Zimmer and M. G. Currie, Discovery of IWP-051, a novel orally bioavailable sGC stimulator with once-daily dosing potential in humans, ACS Med. Chem. Lett., 2016, 7, 465–469 CrossRef CAS PubMed.
- G. S. Masaret, Synthesis, Docking and Antihypertensive Activity of Pyridone Derivatives, ChemistrySelect, 2020, 5, 13995–14003 CrossRef CAS.
- A. S. Mehanna and J. Y. Kim, Design, synthesis, and biological testing of thiosalicylamides as a novel class of calcium channel blockers, Bioorg. Med. Chem., 2005, 13, 4323–4331 CrossRef CAS PubMed.
- F. Hernández, A. Sánchez, P. Rendón-Vallejo, C. Millán-Pacheco, Y. Alcaraz, F. Delgado, M. A. Vázquez and S. Estrada-Soto, Synthesis, ex vivo and in silico studies of 3-cyano-2-pyridone derivatives with vasorelaxant activity, Eur. J. Med. Chem., 2013, 70, 669–676 CrossRef PubMed.
- A. S. Girgis, A. Kalmouch and M. Ellithey, Synthesis of novel vasodilatory active nicotinate esters with amino acid function, Bioorg. Med. Chem., 2006, 14, 8488–8494 CrossRef CAS PubMed.
- Z. M. Nofal, A. M. Srour, W. I. El-Eraky, D. O. Saleh and A. S. Girgis, Rational design, synthesis and QSAR study of vasorelaxant active 3-pyridinecarbonitriles incorporating 1H-benzimidazol-2-yl function, Eur. J. Med. Chem., 2013, 63, 14–21 CrossRef CAS PubMed.
- A. M. Srour, S. S. Abd El-Karim, D. O. Saleh, W. I. El-Eraky and Z. M. Nofal, Rational design, synthesis and 2D-QSAR study of novel vasorelaxant active benzofuran-pyridine hybrids, Bioorg. Med. Chem. Lett., 2016, 26, 2557–2561 CrossRef CAS PubMed.
- N. M. Khalifa, A. M. Srour, S. S. AbdEl-Karim, D. O. Saleh and M. A. Al-Omar, Synthesis and 2D-QSAR Study of Active Benzofuran-Based Vasodilators, Molecules, 2017, 22, 1820 CrossRef PubMed.
- A. M. Srour, D. H. Dawood and D. O. Saleh, Synthesis, 3D-pharmacophore modelling and 2D-QSAR study of new pyridine-3-carbonitriles as vasorelaxant active agents, New J. Chem., 2021, 45, 7731–7740 RSC.
- S. Singh, K. Agarwal, H. Iqbal, P. Yadav, D. Yadav, D. Chanda, S. Tandon, F. Khan, A. K. Gupta and A. Gupta, Synthesis and evaluation of substituted 8,8-dimethyl-8H-pyrano[2,3-f]chromen-2-one derivatives as vasorelaxing agents, Bioorg. Med. Chem. Lett., 2020, 30, 126759 CrossRef CAS PubMed.
- T. Yamanaka, M. Yasumoto, T. Nakajima and O. Yaoka, Chem. Abstr., 1993, 118, 169097 Search PubMed , Jpn. Kokai Tokkyo Koho JP 04,282,353 (92,282,353) (Cl. C07C239/20), 7 Oct. 1992..
- M. Baumgarth, R. Gericke, I. Lues, J. De Peyer and R. Bergmann, Chem. Abstr., 1989, 111, 97213 Search PubMed , Eur. Pat. Appl. EP 308,792 (Cl. C07D491/04), 29 Mar. 1989..
- J. M. Evans, G. Stemp and F. Cassidy, Chem. Abstr., 1987, 106, 213921 Search PubMed , Eur. Pat. Appl. EP 205,292 (Cl. C07D491/04), 17 Dec. 1986..
- A. S. Girgis, N. S. M. Ismail and H. Farag, Facile synthesis, vasorelaxant properties and molecular modeling studies of 2-amino-8a-methoxy-4H-pyrano[3,2-c]pyridine-3-carbonitriles, Eur. J. Med. Chem., 2011, 46, 2397–2407 CrossRef CAS PubMed.
- A. Malki, M. Mohsen, H. Aziz, O. Rizk, O. Shaaban, M. El-Sayed, Z. A. Sherif and H. Ashour, New 3-Cyano-2-Substituted Pyridines Induce Apoptosis in MCF 7 Breast Cancer Cells, Molecules, 2016, 21, 230 CrossRef PubMed.
- K. M. Abouzid, G. H. Al-Ansary and A. M. El-Naggar, Eco-friendly synthesis of novel cyanopyridine derivatives and their anticancer and PIM-1 kinase inhibitory activities, Eur. J. Med. Chem., 2017, 134, 357–365 CrossRef CAS PubMed.
- R. Sabour, M. F. Harras, O. M. Al Kamaly and N. Altwaijry, Discovery of Novel 3-Cyanopyridines as Survivin Modulators and Apoptosis Inducers, Molecules, 2020, 25, 4892 CrossRef CAS PubMed.
- G. Gudipudi, S. R. Sagurthi, S. Perugu, G. Achaiah and G.
L. David Krupadanam, Rational design and synthesis of novel 2-(substituted-2H-chromen-3-yl)-5-aryl-1H-imidazole derivatives as an anti-angiogenesis and anti-cancer agent, RSC Adv., 2014, 4, 56489–56501 RSC.
- H. E. A. Ahmed, M. A. A. El-Nassag, A. H. Hassan, R. M. Okasha, S. Ihmaid, A. M. Fouda, T. H. Afifi, A. Aljuhani and A. M. El-Agrody, Introducing novel potent anticancer agents of 1H-benzo[f]chromene scaffolds, targeting c-Src kinaseenzyme with MDA-MB-231 cell line anti-invasioneffect, J. Enzyme Inhib. Med. Chem., 2018, 33, 1074–1088 CrossRef CAS PubMed.
- A. S. Girgis, D. O. Saleh, R. F. George, A. M. Srour, G. G. Pillai, C. S. Panda and A. R. Katritzky, Synthesis, bioassay, and QSAR study of bronchodilatory active 4H-pyrano[3,2-c]pyridine-3-carbonitriles, Eur. J. Med. Chem., 2015, 89, 835–843 CrossRef CAS PubMed.
- T. Rudel, Caspase inhibitors in prevention of apoptosis, Herz, 1999, 24, 236–241 CrossRef CAS PubMed.
- P. D. Mace, S. J. Ried and G. S. Salvesen, Chapter seven-caspase enzymology and activation mechanisms, Methods Enzymol., 2014, 544, 161–178 CAS.
- T. M. Thornton and M. Rincon, Non-Classical P38 Map Kinase Functions: Cell Cycle Checkpoints and Survival, Int. J. Biol. Sci., 2009, 5, 44–52 CrossRef CAS PubMed.
- A. E. G. E. Amr, E. A. Elsayed, M. A. Al-Omar, H. O. Badr Eldin, E. S. Nossier and M. M. Abdallah, Design, synthesis, anticancer evaluation and molecular modeling of novel estrogen derivatives, Molecules, 2019, 24(3), 416 CrossRef PubMed.
- E. S. Nossier, S. M. El-Hallouty and E. R. Zaki, Synthesis, anticancer evaluation and molecular modeling of some substituted thiazolidinonyl and thiazolyl pyrazole derivatives, Int. J. Pharm. Sci., 2015, 7, 353–359 CAS.
- B. Feeney, C. Pop, P. Swartz, C. Mattos and A. C. Clark, Role of loop bundle hydrogen bonds in the maturation and activity of (Pro) caspase-3, Biochemistry, 2006, 45(44), 13249–13263 CrossRef CAS PubMed.
- S. Diab, T. Teo, M. Kumarasiri, P. Li, M. Yu, F. Lam, S. K. C. Basnet, M. J. Sykes, H. Albrecht, R. Milne and S. Wang, Discovery of 5-(2-(phenylamino) pyrimidin-4yl)thiazol-2(3H)-one derivatives as potent Mnk2 inhibitors: synthesis, SAR analysis and biological evaluation, ChemMedChem, 2014, 9, 962–972 CrossRef CAS PubMed.
- V. Gurtu, S. R. Kain and G. Zhang, Fluorometric and Colorimetric Detection of Caspase Activity Associated with Apoptosis, Anal. Biochem., 1997, 251, 98–102 CrossRef CAS PubMed.
- N. Dong, X. Liu, T. Zhao, L. Wang, H. Li, S. Zhang, X. Li, X. Bai, Y. Zhang and B. Yang, Apoptosis-inducing effects and growth inhibitory of a novel chalcone, in human hepatic cancer cells and lung cancer cells, Biomed. Pharmacother., 2018, 105, 195–203 CrossRef CAS PubMed.
Footnote |
† Electronic supplementary information (ESI) available. See DOI: 10.1039/d1ra04758b |
|
This journal is © The Royal Society of Chemistry 2021 |
Click here to see how this site uses Cookies. View our privacy policy here.