DOI:
10.1039/D1RA04525C
(Paper)
RSC Adv., 2021,
11, 24416-24423
Combined first-principles calculations and experimental study on the photocatalytic mechanism of natural dolomite
Received
11th June 2021
, Accepted 6th July 2021
First published on 12th July 2021
Abstract
Mineral-based photocatalysts have received great attention due to their low cost. In this study, the photocatalytic activity of natural dolomite and its mechanism were investigated based on designed experiments and first-principles calculations. The kinetic study showed that natural dolomite showed notable photocatalytic activity for the degradation of target compounds including methylene blue, diphenhydramine, and tetracycline. The EPR analysis demonstrated that O2−˙, ˙OH, and 1O2 were produced in the dolomite system under simulated sunlight irradiation. The first-principles calculations indicated that the isomorphous substitution of Fe2+ for Mg2+ in the dolomite lattice led to the impurity levels appearing in the forbidden band, which caused a significant decrease of the band gap from 5.02 to 1.63 eV. As a result, natural dolomite could act as a semiconductor photocatalyst in photochemical reactions due to the substitution of Mg2+ by Fe2+. Under simulated sunlight irradiation, photogenerated electron–hole pairs in the natural dolomite were separated and transferred to the surface, and then formed reactive radicals through further reactions, thereby enhancing the degradation of target compounds. This research may contribute to the understanding of the photocatalytic activity of natural minerals.
1. Introduction
Natural minerals are widely distributed at the Earth's surface. As important raw materials, they are extensively used in the different branches of industry. In the field of heterogeneous photocatalysis, it has been widely recognized that natural minerals may play an important role as photocatalysts or substrates of photocatalysts due to their low cost.1–7 So far, a large number of studies have been carried out to investigate the photocatalytic activity of natural minerals, and to further develop mineral-based photocatalytic applications. For example, the photocatalytic activity and mechanisms of natural semiconducting minerals, including rutile,7,8 iron oxides,9 sphalerite,7,10 and manganese oxides,11 have been widely studied via experiments and theoretical calculations, and their applications as photocatalysts in the degradation of toxic and hazardous pollutants are also conducted. A recent study reported the application of natural wolfremite as a novel visible-light photocatalyst for organics degradation.12 In addition, an excellent review by Lu provides overviews of various natural minerals as potential photocatalysts.13 Besides semiconductor minerals, several clay minerals such as montmorillonite14,15 and kaolinite,16 were demonstrated to have photocatalytic activity under simulated sunlight irradiation. Moreover, many studies reported that natural minerals were used as substrate materials to synthesize photocatalysts for the enhancement of photocatalytic activity.17
A comprehensive understanding of the photocatalytic properties of natural minerals is important to develop mineral-based photocatalytic applications. However, most studies center on natural semiconductor minerals, and few studies focus on the photocatalytic properties of natural carbonate minerals. Dolomite is a double-carbonate mineral with chemical formula CaMg(CO3)2, and its theoretical compositions consist of 30.41% CaO, 21.86% MgO, and 47.73% CO2. In natural dolomite, a isomorphous substitution of Mg2+ by Fe2+ is often observed.18 As an inexpensive material, dolomite is widely used in different industrial fields, such as building construction, ceramic and plastic production, iron and steel making, soil remediation, and effluent treatment.19–21 Besides these traditional applications, the dolomite is also applied in the synthesis of composite catalysts. Some studies reported the applications of dolomite based catalyst in the removal of acidic gaseous pollutants and the production of biodiesel and glycerol carbonate.22,23
To the best of our knowledge, there is no studies on the photocatalytic properties of natural dolomite. If natural dolomite has photocatalytic activity under sunlight irradiation, it may be used as a novel low-cost photocatalyst, or used to synthesize dolomite-based photocatalyst. Based on this idea, we planned to investigate the photocatalytic properties of natural dolomite, and further discussed its photocatalytic mechanism under sunlight based on the first-principles calculations. This research will contribute to the understanding of the photocatalytic activity and mechanism of natural minerals.
2. Materials and methods
2.1 Materials
The natural dolomite sample used in this work was obtained from Jinyuan Co., Ltd (Zhengzhou, China). Methylene blue (MB), diphenhydramine hydrochloride (DP), and tetracycline hydrochloride (TC) with purity >98% were purchased from Sinopharm Chemical Reagent Co., Ltd (Beijing, China). Chemical reagents including acetonitrile, acetic acid, methanol, and triethylamine were high-performance liquid chromatography (HPLC) grade, and purchased from J&K Chemical Co., Ltd (Beijing, China). The spin-trapping reagents 2,2,6,6-tetramethylpiperidine (TEMP) and 5,5-dimethyl-1-pyrroline N-oxide (DMPO) used for electron paramagnetic resonance (EPR) experiments were supplied by Tokyo Chemical Industry Co., Ltd (Tokyo, Japan). Milli-Q water (18 MΩ cm−1) was used to prepare all solutions.
2.2 Pre-treatment and characterization of the natural dolomite sample
The natural dolomite was first passed through a 100-mesh sieve to obtain a uniform particle size. Then, the obtained sample was cleaned by Milli-Q water for four times, and dried in room temperature for 24 h. No chemical treatments were conducted on the sample in order to preserve its natural attributes. The morphology of the dolomite sample was recorded by a JEOL, JSM-7610F scanning electron microscope (SEM). Powder X-ray diffraction (XRD) pattern was obtained with a Philips X'Pert-Pro diffractometer equipped with Cu-Kα radiation, monitoring in the range from 2θ = 5 to 80°, and the collected data were processed using MDI Jade software (version 6.5). The elemental composition of the dolomite sample was quantified using a S4 Pioneer X-ray fluorescence (XRF) spectrometer (Bruker AXS, Germany). The UV-Vis diffuse reflectance spectra (DRS) of the natural dolomite were measured by a UV-Vis spectrophotometer (U4100, Hitachi) with an integration sphere.
2.3 Photocurrent measurement
The photocurrent measurements were performed on a CHI-660C electrochemistry workstation (Chenhua, China) with a three-electrode system: the dolomite-coated FTO glass (effective area is 1.0 cm2) as the working electrode, a saturated calomel electrode (SCE) as the reference electrode, and a Pt wire as the counter electrode. The supporting electrolyte was a 0.5 M Na2SO4 aqueous solution. A 150 W xenon light resource with a reflector (300–800 nm) was used as the irradiation source. The photocurrent response was carried out at a constant potential of +0.4 V to the working photoanode. A manual shutter controlled the exposure time of the sample to the light with a interval of 20 s.
2.4 Photoactivity evaluation
Photocatalytic degradation was performed in a PR22-25 photochemical reactor (PerfectLight, China), which is equipped with a PLS-SXE300UV xenon light resource and a DC-0506 water-cooling system (HengPing, Shanghai). The output wavelength range of xenon lamp is in the range between 300 and 800 nm, and the light power input into the reactor was about 120 mW cm−2. During irradiation, the mixture was stirred constantly, and the temperature in the reactor was maintained at 4 °C through circulating water. For each experiment, 0.5 g dolomite sample was dispersed in 100 mL MB (10 mg L−1), DP (20 mg L−1), or TC (20 mg L−1) solution in the 250 mL reactor. And then, the solution containing dolomite was magnetically stirred for 1 h to establish the adsorption equilibrium. At the end of equilibrium, the suspension began to be irradiated. A 2 mL aliquot of the suspension was collected at 15 min intervals, filtered through a 0.22 μm membrane, and analyzed by HPLC or UV-Vis spectrophotometer. All experiments were performed in duplicate for each condition.
2.5 Analytical determination and detection of reactive species
The concentration of MB was measured by a UV-vis spectrophotometer (Shimadzu, UV 2450) at the maximum absorption wavelength of 664 nm. The concentrations of DP and TC were determined with a Shimadzu HPLC (LC-20) equipped with a C18 ODS reversed phase column (4.6 mm × 150 mm, 5 μm) and a SPD20 UV detector. For the determination of DP, the mobile phase consisted of 25 mM acetic acid and acetonitrile (60
:
40, v/v), with pH adjusted to 6.0 ± 0.1 using triethylamine. While for the determination of TC, the mobile phase was composed of 0.01 M oxalic acid, acetonitrile, and methanol (65
:
25
:
15, v/v). The detector wavelengths for DP and TC determination were set to 220 and 355 nm, respectively. Isocratic elution was performed at a flow rate 0.8 mL min−1. The injection volume was 20 μL, and oven temperature was kept at 35 °C.
EPR signals of reactive radicals trapped by TEMP and DMPO were detected on a Bruker EMXplus EPR spectrometer equipped with an situ irradiation source (a Quanta-Ray Nd:YAG laser system with λ = 355 nm). The operating parameters were set as follows: center field 3227.67 G; microwave frequency 9054.62 MHz; power 0.998 mW.
2.6 Method of calculation
To probe the photocatalytic mechanism of natural dolomite, the first-principles calculations based on the density functional theory (DFT) were carried out using the Vienna Ab initio Simulation Package (VASP).24 The generalized gradient approximation (GGA) with the Perdew–Burke–Ernzerhof (PBE) parametrization were employed to deal with the exchange–correlation functional.25,26 The energy cutoff for plane-wave basis was set at 500 eV, and a 4 × 4 × 1 Monkhorst–Pack k-point mesh was used for the Brillouin zone integration.27,28 For each calculation, the convergence thresholds for the maximum energy change and force were 1.0 × 10−5 eV per atom and 0.01 eV Å−1 per atom, respectively. As shown in Fig. 7(a), a 2 × 2 × 1 supercell (including 6 C, 18 O, 3 Mg, and 3 Ca atoms) was built to model the natural dolomite, and the lattice parameters of unit cell were a = b = 4.81 Å, c = 16.01 Å.29 To simulate the isomorphous substitution of Fe2+ for Mg2+ in natural dolomite, the Mg2+ ions in the unit cell are randomly replaced by Fe2+ ions, with the number of Fe2+ ions varying from 1 to 9. The random substitution of 3 Fe2+ for 3 Mg2+ in the unit cell is shown in Fig. 7(b).
3. Results and discussion
3.1 Characterization results
SEM images of the natural dolomite are depicted in Fig. 1, which indicated a smooth surface morphology, characteristic of dolomite. Such a microstructure was attributed to the deposition environment of carbonates, and generally led to low internal porosity and surface area.
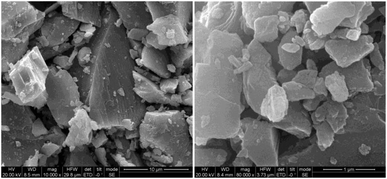 |
| Fig. 1 SEM image of the natural dolomite at different magnifications. | |
The XRD pattern of the sample showed that characteristic reflections of dolomite (Fig. 2). Main peaks of the dolomite sample located at 31.0, 33.5, 37.3, 41.1, 44.9, and 50.5°, which was consistent with that reported by Sdiri.30 No obvious peaks of impurities was observed, indicative of a high purity dolomite.
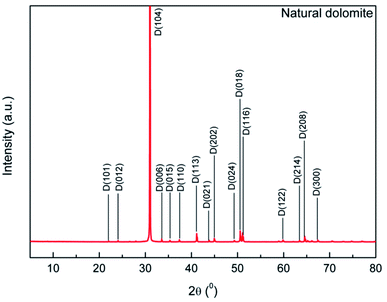 |
| Fig. 2 XRD pattern of the natural dolomite and analysis with MDI Jade 6.5 software. | |
Chemical compositions of the dolomite sample determined by XRF analysis showed high amounts of CaO and MgO (Table 1), indicating a high purity dolomite. Besides, the dolomite sample also contained a small amount of impurities, such as SiO2, Al2O3, and Fe2O3. Among of them, the content of Fe2O3 reached 1.84%, which could be ascribed to the isomorphous substitution of Mg2+ by Fe2+ in the dolomite lattice.
Table 1 Elemental compositions of natural dolomite determined by X-ray fluorescence spectrometer
Composition (%) |
CO2 |
MgO |
Al2O3 |
SiO2 |
P2O5 |
SO3 |
K2O |
CaO |
Fe2O3 |
52.6 |
23.8 |
0.153 |
0.135 |
0.008 |
0.048 |
0.014 |
21.4 |
1.84 |
The UV-vis DRS spectra of the sample is shown in Fig. 3. It indicated that the natural dolomite exhibited significant absorption in the wavelength range of 200–600 nm, with a absorption maximum at 228 nm (Fig. 3(a)). Based on this spectra, the energy band gap of the natural dolomite obtained from extrapolation of linear portion of the absorption edge was estimated to be 1.28 eV,31 suggesting a semiconducting nature (Fig. 3(b)).
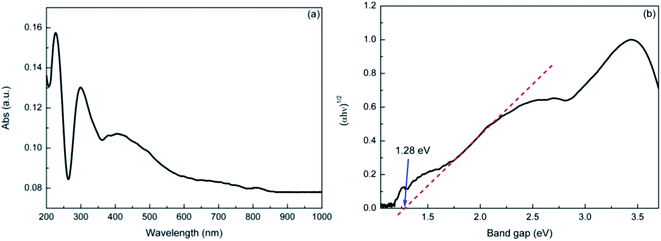 |
| Fig. 3 The UV-Vis diffuse reflectance spectra of natural dolomite (a), and the estimated band gap (b). | |
3.2 Photocatalytic degradation of MB, DP and TC by natural dolomite
To examine the photocatalytic activity of natural dolomite, the MB, DP, and TC were selected as target compounds to model organic pollutants. MB is a dye, a compound widely used in evaluating the catalytic performance of photocatalyst. DP and TC are two pharmaceuticals, which belong to emerging pollutants. Fig. 4 displays the photodegradation of MB, DP, and TC versus irradiation time in the absence and presence of natural dolomite, as well as the dark controls with dolomite. The degradation rates for MB, DP, and TC in pure aqueous phase were 53%, 12%, and 7%, respectively, and the photolysis kinetics obeyed the pseudo-first order kinetics, with rate constants 0.46 × 10−2, 0.16 × 10−2, and 0.09 × 10−2 min−1 (Table 2). When the natural dolomite was added to the solution, the degradation rates for MB, DP and TC increased to 79%, 62% and 80%, respectively, with the corresponding rate constants increased to 0.87 × 10−2, 1.29 × 10−2, and 2.13 × 10−2 min−1 (Table 2). The dark controls were performed to determine the adsorption of target compounds on the dolomite during irradiation. The results showed that the loss of MB, DP, and TC caused by adsorption were about 11%, 5%, and 9%, respectively. Apparently, the degradation rates calculated after deducting the amount of adsorption significantly increased in the presence of natural dolomite. This result suggested that the natural dolomite had notable photocatalytic activity, and could be use as a potential photocatalyst. In addition, from an environmental perspective, the natural dolomite may also play an important role in environmental self-purification.
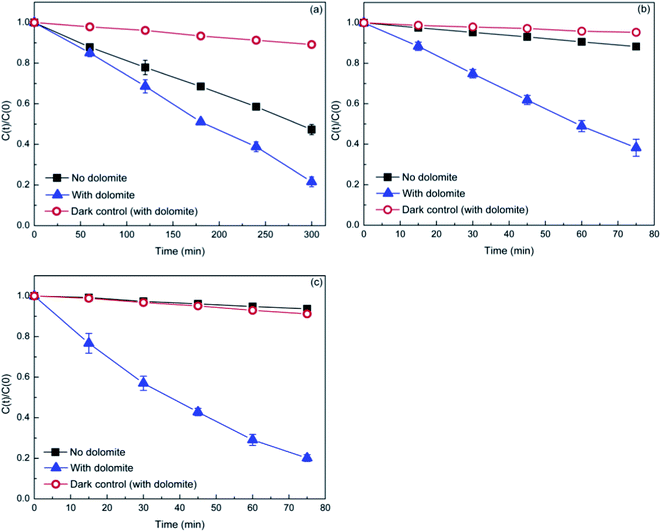 |
| Fig. 4 Photocatalytic degradation of MB (a), DP (b), and TC (c) in the presence of the natural dolomite (0.5 g). The initial concentrations of MB, DP, and TC are 10, 20, and 20 mg L−1, respectively. | |
Table 2 Fitted parameters for MB, DP, and TC photodegradation in the absence and presence of natural dolomite by pseudo-first-order model
Reaction condition |
KDP (min−1) |
R2 |
MB in water |
(0.46 ± 0.02) × 10−2 |
0.994 |
MB + dolomite |
(0.87 ± 0.07) × 10−2 |
0.967 |
DP in water |
(0.16 ± 0.02) × 10−2 |
0.998 |
DP + dolomite |
(1.29 ± 0.08) × 10−2 |
0.982 |
TC in water |
(0.091 ± 0.004) × 10−2 |
0.991 |
TC + dolomite |
(2.13 ± 0.08) × 10−2 |
0.993 |
In order to evaluate the recyclability and stability of the natural dolomite in the photocatalytic applications, cycling experiments were performed. The results illustrated that the degradation rate for DP, MB, and TC was 62%, 78%, and 79% in the first cycle, respectively, but significantly decreased to 30%, 46%, and 66% in the second cycle (Fig. 5). At the end of 3 cycles, the photocatalytic activity of the natural dolomite further decreased, suggesting that deactivation of the dolomite could occurred after repeated applications.
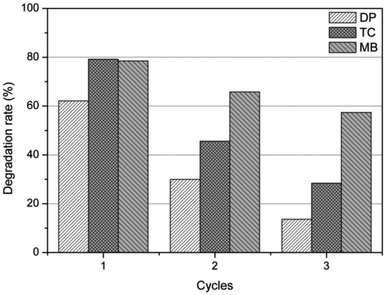 |
| Fig. 5 Photocatalytic activity of the natural dolomite after repeated use for 3 times. | |
3.3 Detection of reactive oxygen species
Usually, photogenerated reactive oxygen species (ROS), including hydroxyl radical (˙OH), superoxide anion radical (O2−˙), and singlet oxygen (1O2) are often considered to play a crucial role in photocatalytic reactions. Therefore, it is reasonable to speculate that the enhanced degradation of target compounds may also be attributed to the photogenerated ROS in the dolomite system. As such, the EPR spin-trapping experiments was performed to determine the ROS produced in the irradiated dolomite system. As shown in Fig. 6, no obvious signal was detected in the dark condition, but after irradiation, the characteristic peaks of TEMP–1O2, DMPO–O2−˙, and DMPO–OH adducts appeared, and gradually strengthened with the irradiation time. This result clearly demonstrated the generation of 1O2, O2−˙, and ˙OH in the dolomite system under simulated sunlight irradiation. Furthermore, the strong signals indicated that the radicals generated in the dolomite system was considerable. These reactive radicals further reacted with target compounds, resulting in their enhanced degradation.
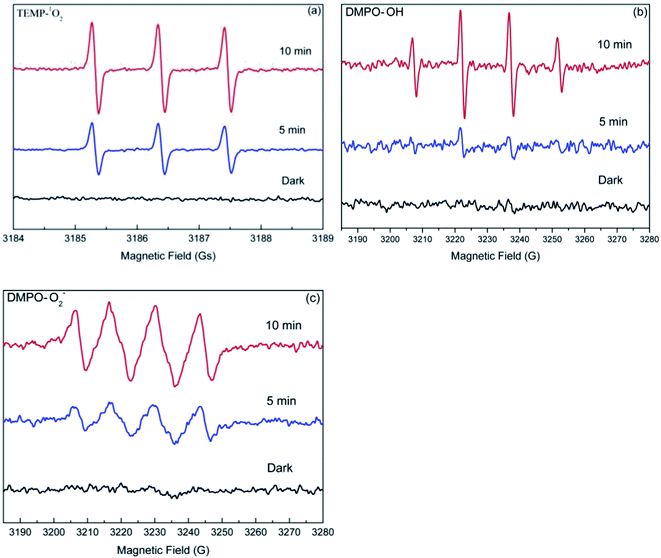 |
| Fig. 6 EPR spectra of the TEMP–1O2 (a), DMPO–˙OH (b), and DMPO–O2−˙ (c) adducts generated in the natural dolomite system after simulated sunlight irradiation. | |
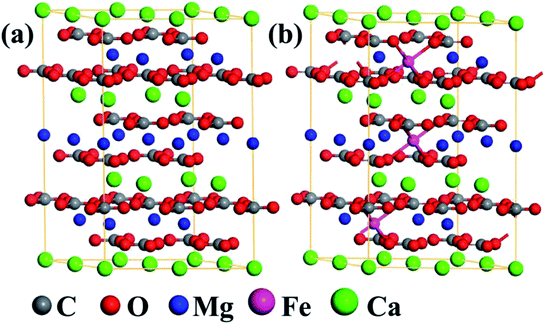 |
| Fig. 7 The optimized structures of (a) the 2 × 2 × 1 dolomite supercell, and (b) the supercell with substitution of 3 Fe2+ for 3 Mg2+. | |
3.4 Photocatalytic mechanisms of the natural dolomite
Density functional theory calculations were performed to further probe the photocatalytic mechanism. Fig. 8 displays the electronic band structure of pristine and Fe2+-substituted dolomite. For the pristine dolomite, the band gap energy is calculated to be 5.02 eV (Fig. 8(a)), which is consistent with the result previously reported by Hossain et al.32 With the substitution of 3 Mg2+ ions by 3 Fe2+ ions, there are two impurity levels appearing in the forbidden band, causing the maximum band gap to be reduced to 2.04 eV (Fig. 8(b)). As more Mg2+ are substituted by Fe2+ ions, more impurity levels arise in the forbidden band, and the impurity bands widen, thereby causing a further decrease of the band gap. When 9 Mg2+ ions are replaced, the maximum band gap decreases to 1.63 eV (Fig. 8(d)), which is close to the band gap (1.28 eV) estimated from the UV-Vis reflectance spectra of the natural dolomite (Fig. 3(b)). The decrease of band gap from 5.02 to 1.63 eV suggests that the natural dolomite could exhibit the characteristics of a semiconductor photocatalyst after partial substitution of Mg2+ by Fe2+.
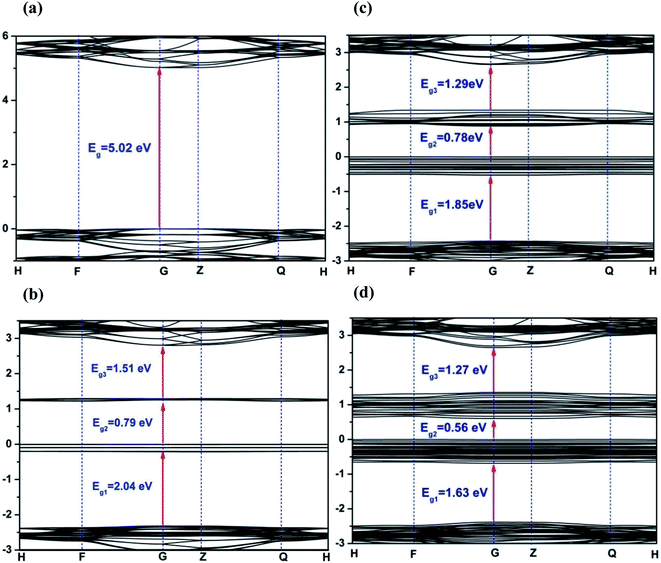 |
| Fig. 8 Band structures of dolomite supercell with 0 (a), 3 (b), 6 (c), and 9 (d) Fe2+ substitution. The Fermi level is set to 0. | |
Densities of states (DOS) for pristine and Fe2+-substituted dolomite is presented in Fig. 9. According to the DOS, the valence band is mainly composed of O 2p state, and the conduction band consists of O 2p, C 2p, Mg 3p, and Ca 3d states, but the role of C 2p state is major (Fig. 9(a)). The two impurity bands appearing in the forbidden gap are predominantly contributed by Fe 3d and Ca 3d states (Fig. 9(b)), and show more delocalization with the increasing concentration of Fe2+.
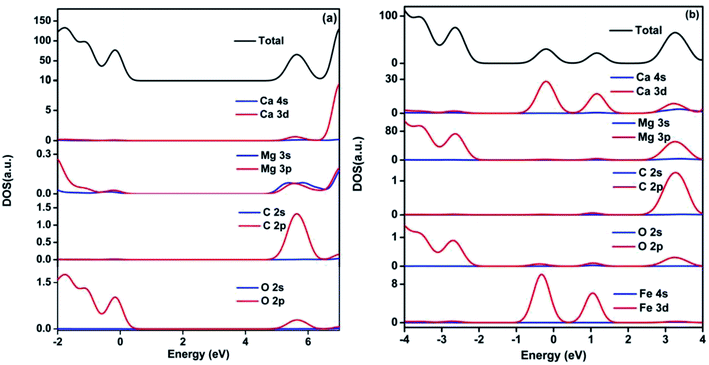 |
| Fig. 9 Density of state of pristine (a) and 3 Fe2+-substituted (b) dolomite. The Fermi level is set to 0. | |
In summary, the results obtained from theoretical calculations demonstrated that the substitution of Fe2+ for Mg2+ in the dolomite lattice resulted in the decrease of band gap, which caused the natural dolomite to exhibit photocatalytic activity. To further verify the photocatalytic mechanism, photocurrent was measured for the natural dolomite (Fig. 10). It was clear that obvious photocurrent response were observed, indicating a photoinduced electrons and holes separation.
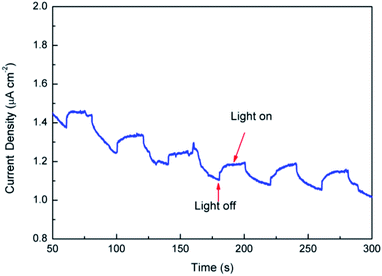 |
| Fig. 10 Photocurrent of the natural dolomite under simulated sunlight irradiation. | |
On the basis of the above results, the photocatalytic mechanism for the natural dolomite was proposed. Under simulated sunlight irradiation, photoinduced electrons and holes separate and migrate to the dolomite surface (eqn (1)). Then, further reactions according to eqn (2)–(6) led to the formation of ˙OH and O2−˙. As to the generation of 1O2, it might be ascribed to the energy transfer between excited dolomite and dissolved oxygen in aqueous solution (eqn (7)).
|
Dolomite + hν → h+ + e−
| (1) |
|
H2O2 + O2−˙ → ˙OH + OH− + O2
| (6) |
|
Dolomite* + O2 → 1O2 + dolomite
| (7) |
Besides, considering the release of trace Fe ions into the solution during irradiation, it could be speculated that the photo-Fenton-like reaction induced by released Fe ions might also contribute to the degradation of target compounds.
4. Conclusion
For the first time, the photocatalytic activity of natural dolomite was investigated using MB, DP, and TC as target compounds, and the mechanism was further discussed based on EPR analysis and the first-principles calculations. The results indicated that the natural dolomite had notable photocatalytic activity for the degradation of the target compounds. The EPR analysis demonstrated that 1O2, O2−˙, and ˙OH generated in the irradiated dolomite system, and are responsible for the accelerated degradation of target compounds. The first-principles calculations indicated that the partial substitution of Fe2+ for Mg2+ in the dolomite lattice led to the impurity bands appearing in the forbidden gap, causing a significant decrease of band gap. Therefore, the natural dolomite could exhibit the properties of semiconductor photocatalyst in photoreactions. Based on these results, the mechanism of natural dolomite photocatalysis was proposed. First, the dolomite excited by simulated sunlight generates electrons and holes, which subsequently migrate to the dolomite surface, and react to form ROS via further reactions. Second, the ROS react with target compounds to cause their degradation.
Conflicts of interest
There are no conflicts to declare.
Acknowledgements
This work was supported by the National Natural Science Foundation of China (Grant 41403083), the Key Project of Science and Technology of Hubei Provincial Department of Education (Grant D20141305), Natural Science of Foundation of Hubei Province, China (Grant 2019CFB225), and the Science Foundation of Education Commission of Hubei Province of China (Grant T2020008).
References
- D. Papoulis, Appl. Clay Sci., 2019, 168, 164–174 CrossRef CAS.
- B. Szczepanik, Appl. Clay Sci., 2017, 141, 227–239 CrossRef CAS.
- Y. Zhang, H. Gan and G. Zhang, Chem. Eng. J., 2011, 172, 936–943 CrossRef CAS.
- O. Kozák, P. Praus, K. Kočí and M. Klementová, J. Colloid Interface Sci., 2010, 352, 244–251 CrossRef.
- T. Liu, X. Chen, Y. Dai, L. Zhou, J. Guo and S. Ai, J. Alloys Compd., 2015, 649, 244–253 CrossRef CAS.
- Y. Li, C. Ding, Y. Liu, Y. Li, A. Lu, C. Wang, and H. Ding, Visible light photocatalysis of natural semiconducting minerals, in Advances in Photocatalytic Disinfection. Green Chemistry and Sustainable Technology, ed. T An, T Zhao, and P Wong, Springer, Berlin, Heidelberg, 2017 Search PubMed.
- M. A. A. Schoonen, Y. Xu and D. R. Strongin, J. Geochem. Explor., 1998, 62, 201–215 CrossRef CAS.
- R. I. Bickley, G. Munuera and F. S. Stone, J. Catal., 1973, 31, 398–407 CrossRef CAS.
- S. Belaidi, N. Setifi, L. Mammeri, W. Remache, K. Benhamouda, T. Sehili and K. Djebbar, Desalin. Water Treat., 2018, 113, 171–178 CrossRef CAS.
- Y. Li, A. Lu and C. Wang, Acta Geol. Sin. (Engl. Ed.), 2009, 3, 633–639 CrossRef.
- S. Ristig, N. Cibura and J. Strunk, Green, 2015, 5, 1–6 Search PubMed.
- L. Li, Y. Li, Y. Li, A. Lu, H. Ding, P. K. Wong, H. Sun and J. Shi, Catal. Today, 2019, 358, 177–183 CrossRef.
- A. Lu, Acta Petrol. Mineral., 2003, 22, 323–331 CAS.
- Y. Liu, X. Zhang and F. Wu, Appl. Clay Sci., 2010, 49, 182–186 CrossRef CAS.
- Q. Wu, Z. Que, Z. Li, S. Chen, W. Zhang, K. Yin and H. Hong, J. Hazard. Mater., 2018, 359, 414–420 CrossRef CAS PubMed.
- D. Kibanova, M. Trejo, H. Destaillats and J. Cervini-Silva, Catal. Commun., 2011, 12, 698–702 CrossRef CAS.
- H. Van Damme, F. Bergaya, and D. Challal, Photocatalysis over clay supports, in Homogeneous and heterogeneous photocatalysis, ed. E. Pelizzetti and N. Serpone, Springer, Dordrecht, 1986 Search PubMed.
- R. J. Reeder and W. A. Dollase, Am. Mineral., 1989, 74, 1159–1167 CAS.
- O. Sivrikaya, Ironmaking Steelmaking, 2018, 45, 764–772 CrossRef CAS.
- R. Marouf, N. Khelifa, K. Marouf-Khelifa, J. Schott and A. Khelifa, J. Colloid Interface Sci., 2006, 297, 45–53 CrossRef CAS.
- M. Shaaban, Q. Peng, S. Lin, Y. Wu, M. S. Khalid, L. Wu, Y. Mo and R. Hu, Catena, 2016, 140, 9–14 CrossRef CAS.
- L. M. Correia, N. D. S. Campelo, D. S. Novaes, C. L. Cavalcante, J. A. Cecilia, C. E. Rodríguez and R. S. Vieira, Chem. Eng. J., 2015, 269, 35–43 CrossRef CAS.
- Y. T. Algoufi, G. Kabir and B. H. Hameed, J. Taiwan Inst. Chem. Eng., 2017, 70, 179–187 CrossRef CAS.
- G. Kresse and J. Furthmüller, Comput. Mater. Sci., 1996, 6, 15–50 CrossRef CAS.
- B. Hammer, L. B. Hansen and J. K. Norskov, Phys. Rev. B: Condens. Matter Mater. Phys., 1999, 59, 7413–7421 CrossRef.
- W. Zhang and Y. Xiao, Energy Fuels, 2020, 34, 2425–2434 CrossRef CAS.
- Q. Wang, Z. Q. Liu, D. M. Liu, W. Wang, Z. W. Zhao, F. Y. Cui and G. B. Li, Chem. Eng. J., 2019, 360, 838–847 CrossRef CAS.
- W. Zhang and Y. Xiao, Energy Fuels, 2020, 34, 2425–2434 CrossRef CAS.
- J. Warren, Earth-Sci. Rev., 2000, 52, 1–81 CrossRef CAS.
- A. Sdiri, Environ. Prog. Sustainable Energy, 2018, 37, 1–8 CrossRef.
- Y. Xu, W. Wen and J.-M. Wu, J. Hazard. Mater., 2018, 343, 285–297 CrossRef CAS PubMed.
- F. M. Hossain, G. E. Murch, I. V. Belova and B. D. Turner, Solid State Commun., 2009, 149, 1201–1203 CrossRef CAS.
|
This journal is © The Royal Society of Chemistry 2021 |