DOI:
10.1039/D1RA04022G
(Paper)
RSC Adv., 2021,
11, 29728-29740
The preparation, characterization and catalytic activity of Ni NPs supported on porous alginate-g-poly(p-styrene sulfonamide-co-acrylamide)†
Received
23rd May 2021
, Accepted 23rd August 2021
First published on 6th September 2021
Abstract
Herein, we report the synthesis of nickel nanoparticles under mild conditions using porous alginate-g-poly(p-styrene sulfonamide-co-acrylamide) as a protecting/stabilizing agent and sodium borohydride as a reducing agent. The porous cross-linked polymeric support was prepared via combining the use of sol–gel, nanocasting, and crosslinking techniques, in which the p-styrene sulfonamide monomer (PSSA) and N,N′-methylene-bis (acrylamide) (MBA) cross-linker underwent copolymerization on the surface of sodium alginate in the presence of a SiO2 nanoparticle (NP) template (Alg–PSSA-co-ACA). The prepared catalyst (Alg–PSSA-co-ACA@Ni) showed high catalytic activity for the one-step synthesis of 1,3,4-oxadiazoles from the reaction of hydrazides and aryl iodides through isocyanide insertion/cyclization.
1. Introduction
Oxadiazole and its fused derivatives represent a wide range of biological and pharmacological activities. In particular, 1,3,4-oxadiazoles are one of the most important antitumor drugs in clinical use today.1 They represent the core structure of several antitumor and anti-HIV drugs on the market, including zibotentan,2 and raltegravir.3 Hence, some methods have recently been reported for the synthesis of 1,3,4-oxadiazoles, such as the annulation of methyl ketones or acyl chlorides with hydrazides,4 the cyclization of 1,2-diacylhydrazines,5 the cyclization of acylhydrazones,6 imine C–H bond activation of N-arylidenearoylhydrazide,7 arylation of the 1,3,4-oxadiazoles,8 intramolecular decarboxylation,9 and the isocyanide insertion reaction.10 However, some of these require harsh reaction conditions, and expensive noble and precious metal-based catalyst systems which results in increased production costs owing to the difficultly in separating the desired products. Thus, it is of great significance to develop a novel catalytic system, which can afford the functionalized 1,3,4-oxadiazoles in excellent yields under mild reaction conditions using a heterogeneous support.
Porous polymers have been widely studied as solid supports owing to their high specific surface area and excellent thermal stability. Porous polymers with an appropriate chemical composition allow the fabrication of materials that are both biocompatible and have a uniform porosity.11–14 In order to precisely control the pore size, the synthesis of mesoporous polymers from silica nanoparticles (NPs) using the nanocasting route is a practical and efficient technique.15,16 Although the synthesis of porous polymers has been widely investigated, there is still a lack of practical procedures to prepare mesoporous natural polysaccharides with high chemical and thermal stabilities. Here, a sodium alginate-based polymer with a mesoporous structure was synthesized as a heterogeneous support for the immobilization of Ni NPs.
Sodium alginate is a member of the natural polysaccharides. Owing to its potential to transform into different forms (such as gels, spheres, and fibres), and its non-toxic and eco-friendly nature, it is used as a powerful heterogeneous catalyst. Other factors that play an important role in the catalytic performance of sodium alginate are its biodegradable, biocompatible and abundant nature.17–22 In order to improve the catalytic activity of sodium alginate, specific methods have been proposed, such as blending polymers,23 compounding with natural and synthetic chemical derivatives,24 and grafting copolymerization with vinyl monomers.25–27
Sulfonamide scaffolds have attracted remarkable attention owing to their potential application in medicinal,28 and catalytic activity.29 The results obtained from the catalytic application of sulfonamides indicate that sulfonamides play a vital role in heterogeneous substrates and catalysts.29 Owing to the biomedical and catalytic application of sulfonamides, p-styrene sulfonamide can be considered as a promising candidate for the synthesis of sodium alginate based catalysts using graft copolymerization. Similarly, by grafting of PSSA and crosslinking of N,N′-methylene-bis(acrylamide) with alginate through the nanocasting route a novel type of porous polymer was synthesized. By modifying the chemically active sites and mesoporous structure of Alg–PSSA-co-ACA, it can be used as a novel amphiphilic support for the immobilization of Ni NPs (Scheme 1).
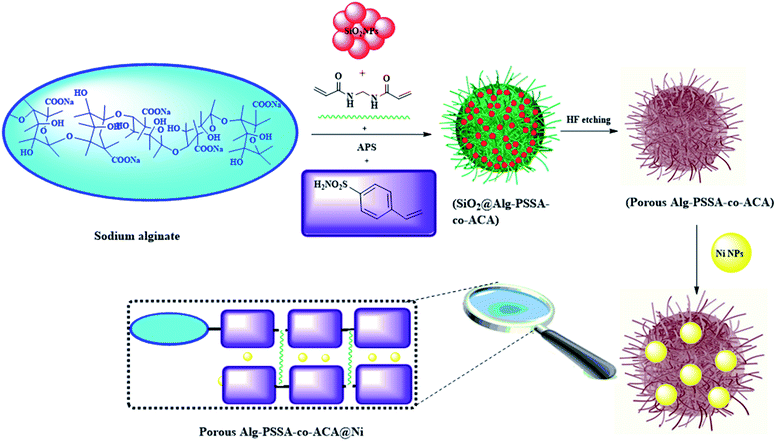 |
| Scheme 1 A schematic diagram of the preparation of SiO2@Alg–PSSA-co-ACA, porous Alg–PSSA-co-ACA, and porous Alg–PSSA-co-ACA@Ni (APS: ammonium persulfate). | |
Therefore, some specific methods used to improve the catalytic performance of porous alginate-g-poly (p-styrene sulfonamide-co-acrylamide) are: (i) creating a mesoporous structure in the alginate-g-poly (p-styrene sulfonamide-co-acrylamide). This mesoporous structure allows the reactants and Ni NPs to rapidly diffuse through the mesopores, and enables the formation of a large surface area; (ii) the addition of PSSA in the catalyst formulation could have promising advantages, including: (a) a high chemical and thermal stability under the reaction conditions,30 and (b) the amphiphilic properties of the catalyst;29d and (iii) the interaction of Ni NPs with PSSA can decrease the self-aggregation and leaching of Ni NPs.31
Following on from our recent work on the design and application of a multifunctional sulfonamide-based catalyst,29,32 and the synthesis of functionalized oxadiazole,29d in this article, we herein report the green synthesis of 1,3,4-oxadiazoles from the reaction of hydrazides, aryl iodides and tert-butyl isocyanide in the presence of an Alg–PSSA-co-ACA@Ni catalyst (Scheme 2). The prepared porous nanocomposite revealed an excellent catalytic functionality for the synthesis of different 1,3,4-oxadiazoles with high yields.
 |
| Scheme 2 The synthesis of 1,3,4-oxadiazole derivatives using Alg–PSSA-co-ACA@Ni in the presence of H2O : EtOH and KHCO3. | |
2. Experimental
2.1. Synthesis of silica alginate-g-poly(p-styrene sulfonamide-co-acrylamide) (SiO2@Alg–PSSA-co-ACA)
The silica NPs were synthesized using the Stöber method.33 Then, the mixture of p-styrene sulfonamide (1.5 g), MBA (0.2 g) and SiO2 NPs (0.05 g) was gradually added to the sodium alginate solution (0.5 g in 20 mL distilled water). Finally, ammonium persulfate (APS) (0.6 g) was added and the mixture was stirred at 60 °C for 2 h. The synthesized SiO2@Alg–PSSA-co-ACA nanocomposite, in the form of a gel, was dried at room temperature (weight: 5 g).
2.2. Synthesis of porous alginate-g-poly(styrene sulfonamide-co-acrylamide) (Alg–PSSA-co-ACA)
In order to synthesize the mesoporous structure, SiO2 NPs of SiO2@Alg–PSSA-co-ACA were selectively removed through etching the silica NPs by mixing a HF solution (5 mL, 10 wt%) with a solution of SiO2@Alg–PSSA-co-ACA (1 g in 20 mL deionized water) in a plastic tube. The prepared solution was stirred for 3 h. The resultant porous Alg–PSSA-co-ACA was separated by filtration, washed with water, and dried (weight: 0.85 g).
2.3. Nickel NPs immobilized on porous alginate-g-poly(p-styrene sulfonamide-co-acrylamide) (Alg–PSSA-co-ACA@Ni)
To synthesize the Alg–PSSA-co-ACA@Ni, the obtained porous Alg–PSSA-co-ACA (0.1 g) in a solution of NiCl2·6H2O (1 M, 5 mL) was stirred at room temperature for 2 h, then the solution of NaBH4 (10 mmol in 5 mL ethanol) was slowly added and stirred at room temperature for 60 min. The functionalized Alg–PSSA-co-ACA@Ni was separated using centrifugation, washed with distilled water (3 × 20 mL), and dried under a vacuum at room temperature for 12 h (weight: 0.3 g) (Scheme 1).34 The amount of Ni incorporated in the support was 1.6 mmol g−1, as corroborated using inductively coupled plasma optical emission spectroscopy (ICP-OES).
2.4. General procedure for the synthesis of the 1,3,4-oxadiazoles
A mixture of substituted benzohydrazide (1 mmol), substituted aryl iodide (1 mmol), KHCO3 (1 mmol), tert-butyl isocyanide (1.5 mmol), and Alg–PSSA-co-ACA@Ni (1.5 mol%, 9 mg) was refluxed in H2O
:
EtOH (2 mL, 1
:
1). After completion of the reaction (monitored using TLC, n-hexane/ethyl acetate, 10
:
4), the catalyst was separated from the reaction mixture by centrifugation and washed with ethanol (10 mL). Finally, the solvent was evaporated and the solid obtained was crystallized from ethanol (10 mL). In some cases, the residue was purified using a short silica gel column using n-hexane/ethylacetate (10
:
4).
2.5. Spectral data for the compounds
2-(2-Bromophenyl)-5-phenyl-1,3,4-oxadiazole. Mp 145–147 °C, 1H NMR (500 MHz, CDCl3-d3) δ 6.83 (d, 2H, J = 8 Hz), 6.94 (t, 1H, J = 7.2 Hz), 7.30 (dd, 3H, J = 7.6, 5.6 Hz), 7.45 (t, 1H, J = 7.6 Hz), 7.69 (d, 1H, J = 7.6 Hz), 7.80 (dd, 1H, J = 6.4, 1.2 Hz). 13C NMR (125 MHz, DMSO-d6) δ 164.7, 163.2, 135.1, 132.6, 132.4, 132.1, 129.8, 129.4, 127.2, 127.1, 126.1, 123.6, 122.9. MS m/z: 299.1.
2.5.2 2-(2-Chlorophenyl)-5-(pyridin-4-yl)-1,3,4-oxadiazole. Mp 128–130 °C, 1H NMR (500 MHz, DMSO-d6) δ 8.85 (d, J = 4.8 Hz, 1H), 8.16 (d, J = 2.8 Hz, 1H), 8.12–8.03 (m, 2H), 7.87 (d, J = 5.5 Hz, 1H), 7.72 (dd, J = 8.1, 2.4 Hz, 1H), 7.65 (qd, J = 8.0, 7.5, 2.7 Hz, 1H), 7.51 (td, J = 8.0, 3.1 Hz, 1H). 13C NMR (125 MHz, DMSO-d6) δ 164.1, 163.3, 151.3, 134.6, 133.7, 133.3, 132.6, 131.9, 131.0, 130.7, 129.2, 128.3, 126.9, 126.0, 125.4, 120.8, MS m/z: 257.1.
2-(5-Phenyl-1,3,4-oxadiazol-2-yl) benzaldehyde. Mp 180–181 °C; 1H NMR (500 MHz, DMSO-d6) δ 6.85 (d, 2H, J = 8.4 Hz), 7.19 (t, 2H, J = 7.6 Hz), 7.44 (d, 1H, 4.8 Hz), 7.49 (dd, 2H, J = 7.6, 7.2 Hz), 7.61 (d, 2H, J = 7.2 Hz); 8.88 (s, 1H). 13C NMR (125 MHz, DMSO-d6) δ, 169.2, 160.1, 147.4, 146.8, 138.7, 134.8, 134.7, 134.1, 132.3, 132.2, 130.8, 130.4, 129.5, 128.0, 127.6, 127.2, 125.8, 125.0, 124.8, 124.5, 124.4, 124.1, 122.7. MS m/z: 250.07.
2-(3-Chlorophenyl)-5-(pyridin-4-yl)-1,3,4-oxadiazole. Mp 143–145 °C; 1H NMR (250 MHz, DMSO-d6) δ 8.85 (s, 2H), 8.19 (s, 1H), 8.10 (s, 3H), 7.69 (dq, J = 14.7, 7.7 Hz, 2H). 13C NMR (63 MHz, DMSO-d6) δ 164.1, 163.2, 151.3, 134.5, 132.6, 131.9, 130.7, 126.9, 126.0, 125.3, 121.0. MS m/z: 257.6.
2-Benzyl-5-phenyl-1,3,4-oxadiazole. Mp 102–103 °C; 1H NMR (250 MHz, DMSO-d6) δ 7.93 (d, J = 6.9 Hz, 2H), 7.56 (d, J = 6.2 Hz, 4H), 7.36 (d, J = 4.4 Hz, 4H), 4.34 (s, 2H). 13C NMR (63 MHz, DMSO-d6) δ 165.9, 164.6, 134.8, 132.2, 129.7, 129.2, 127.6, 126.7, 123.7, 31.2. MS m/z: 236.2.
2-(4-Bromophenyl)-5-phenyl-1,3,4-oxadiazole. Mp 165–166 °C; 1H NMR (250 MHz, DMSO-d6) δ 8.18–7.97 (m, 3H), 7.83 (d, J = 7.2 Hz, 3H), 7.71–7.55 (m, 3H). 13C NMR (63 MHz, DMSO-d6) δ 164.8, 163.8, 132.9, 132.5, 129.8, 129.0, 127.7, 126.1, 123.6, 122.9.
4-(5-Phenyl-1,3,4-oxadiazol-2-yl) aniline. Mp 191–193 °C; 1H NMR (250 MHz, DMSO-d6) δ 8.18–7.98 (m, 2H), 7.75 (d, J = 8.2 Hz, 2H), 7.58 (s, 3H), 6.69 (d, J = 8.2 Hz, 2H), 5.98 (s, 1H). 13C NMR (63 MHz, DMSO) δ 165.2, 163.0, 152.9, 131.9, 129.7, 128.9, 128.6, 127.9, 126.7, 124.1, 114.0, 110.0. MS m/z: 237.2.
2-Benzyl-5-(p-tolyl)-1,3,4-oxadiazole. Mp 93–94 °C; 1H NMR (250 MHz, DMSO-d6) δ 7.79 (d, J = 7.8 Hz, 2H), 7.61–6.96 (m, 7H), 4.31 (s, 2H), 2.48 (s, 3H). 13C NMR (63 MHz, DMSO-d6) δ 165.6, 164.7, 142.3, 136.2, 134.9, 130.3, 129.7, 129.5, 129.3, 129.1, 128.6, 127.9, 127.6, 126.9, 126.7, 121.0, 31.2, 21.4.
2-Phenyl-5-(pyridin-4-yl)-1,3,4-oxadiazole. Mp 139–140 °C; 1H NMR (250 MHz, DMSO-d6) δ 8.83 (s, 2H), 8.13 (d, J = 6.5 Hz, 2H), 8.03 (d, J = 5.0 Hz, 2H), 7.62 (d, J = 9.2 Hz, 3H). 13C NMR (63 MHz, DMSO-d6) δ 165.2, 162.9, 151.3, 132.8, 130.9, 129.9, 127.3, 123.4, 120.7. MS m/z: 223.2.
2-(4-Chlorophenyl)-5-(pyridin-4-yl)-1,3,4-oxadiazole. Mp 140–143 °C; 1H NMR (250 MHz, DMSO-d6) δ 8.14 (d, J = 8.8 Hz, 4H), 7.70 (d, J = 7.5 Hz, 2H), 7.62–7.48 (d, J = 8 Hz, 2H), 2.42 (s, 3H). MS m/z: 257.04.
2-(Pyridin-4-yl)-5-(p-tolyl)-1,3,4-oxadiazole. Mp 140–142 °C; 1H NMR (500 MHz, DMSO-d6) δ 8.13 (d, J = 5 Hz, 2H), 8.04–8.00 (d, J = 2.5 Hz, 2H), 7.64 (d, J = 5 Hz, 2H), 7.44 (d, J = 7.9 Hz, 2H), 2.42 (s, 3H). 13C NMR (125 MHz, DMSO-d6) δ 164.5, 164.2, 142.6, 132.4, 130.4, 130.4, 129.8, 127.1, 127.0, 123.8, 121.0, 21.6. MS m/z: 237.2.
2-(2-Chlorophenyl)-5-phenyl-1,3,4-oxadiazole. Mp 96–98 °C; 1H NMR (250 MHz, DMSO-d6) δ 8.10 (ddt, J = 7.9, 4.6, 1.4 Hz, 3H), 7.73 (dt, J = 7.9, 1.3 Hz, 1H), 7.70–7.52 (m, 5H). 13C NMR (125 MHz, DMSO-d6) δ 13C NMR (63 MHz, DMSO) δ 133.7, 132.7, 131.8, 131.6, 129.9, 128.3, 127.2, 123.5. MS m/z: 257.6.
4-(5-Phenyl-1,3,4-oxadiazol-2-yl) benzoic acid. Mp 217–218 °C; 1H NMR (500 MHz, DMSO-d6) δ 12.6 (s, 1H), 8.34 (s, 1H), 8.24 (d, J = 8.0 Hz, 1H), 8.19–8.09 (m, 4H), 7.64 (d, J = 10 Hz, 3H). 13C NMR (126 MHz, DMSO-d6) δ 166.9, 164.8, 163.9, 134.0, 132.6, 132.5, 130.6, 129.9, 129.8, 129.6, 128.0, 127.4, 127.3, 127.2, 127.1, 123.6. MS m/z: 266.6.
2-(4-Bromophenyl)-5-(3-methoxyphenyl)-1,3,4-oxadiazole. Mp 152–153 °C, 1H NMR (400 MHz, DMSO-d6) δ 8.06 (d, J = 8.0 Hz, 2H), 7.82 (d, J = 8.0 Hz, 2H), 7.70 (d, J = 8.0 Hz, 1H), 7.60 (s, 1H), 7.54 (t, J = 7.7 Hz, 1H), 7.21 (dd, J = 8, 4 Hz, 1H), 3.89 (s, 3H). 13C NMR (100 MHz, DMSO-d6) δ 164.5, 164.4, 160.0, 132.6, 131.2, 129.8, 127.1, 124.6, 123.4, 119.4, 118.5, 111.9, 111.8, 55.8. MS m/z: 330.0.
2,5-Diphenyl-1,3,4-oxadiazole. Mp 132–133 °C; 1H NMR (400 MHz, DMSO-d6) δ 8.12 (dd, J = 8, 4 Hz, 4H), 7.63 (m, 6H), 13C NMR (101 MHz, DMSO) δ 164.6, 132.6, 129.9, 127.1, 123.5.
2-(4-Bromophenyl)-5-(p-tolyl)-1,3,4-oxadiazole. Mp 200–202 °C; 1H NMR (400 MHz, DMSO-d6) δ 8.06 (d, J = 8.4 Hz, 2H), 8.01 (d, J = 8 Hz, 1H), 7.83 (d, J = 6 Hz, 2H), 7.65 (d, J = 8.4 Hz, 1H), 7.44 (d, J = 8 Hz, 2H), 2.49 (s, 3H). MS m/z: 315.0.
2-Phenyl-5-(p-tolyl)-1,3,4-oxadiazole. Mp 122–124 °C; 1H NMR (400 MHz, DMSO-d6) δ 8.13–8.10 (m, 3H), 8.06 (d, J = 9.6 Hz, 1H), 7.82 (d, J = 8 Hz, 1H), 7.64 (t, J = 6.4 Hz, 4H), 2.51 (s, 3H). 13C NMR (100 MHz, DMSO-d6) δ 164.6, 163.8, 132.9, 132.0, 129.8, 129.0, 127.1, 126.1, 123.7, 123.0, 23.2. m/z: 236.
2-(3-Methoxyphenyl)-5-phenyl-1,3,4-oxadiazole. Mp 148–149 °C; 1H NMR (400 MHz, DMSO-d6) δ 8.09 (dd, 3.2, 1.2 Hz, 2H), 7.66 (dd, J = 7.7, 1.3 Hz, 1H), 7.64–7.59 (m, 3H), 7.56 (t, J = 1.6 Hz, 1H), 7.51 (t, J = 8.0 Hz, 1H), 7.21 (dd, 4.0, 2.8 Hz, 1H), 3.82 (s, 3H). 13C NMR (100 MHz, DMSO-d6) δ 164.5, 164.4, 160.0, 132.6, 131.2, 129.8, 127.1, 124.6, 123.4, 119.4, 118.5, 111.9, 111.8, 55.8. m/z: 252.2.
2-(4-Bromophenyl)-5-(pyridin-4-yl)-1,3,4-oxadiazole. Mp 180 °C;1H NMR (400 MHz, DMSO-d6) δ 8.83 (d, J = 5.8 Hz, 2H), 8.08 (dd, J = 10.0, 7.3 Hz, 4H), 7.84 (d, J = 8.5 Hz, 2H). 13C NMR (101 MHz, DMSO-d6) δ 151.2, 133.0, 129.3, 120.9. m/z: 300.1.
2-(2,3-Dichlorophenyl)-5-phenyl-1,3,4-oxadiazole. Mp 146–147 °C; 1H NMR (400 MHz, DMSO-d6) δ 8.51 (ddd, J = 7.8, 6.7, 1.4 Hz, 2H), 8.29 (t, J = 7.9 Hz, 2H), 7.96 (t, J = 7.8 Hz, 2H), 7.57 (dd, J = 2, 1.2 Hz, 2H), 7.49 (t, J = 7.6 Hz, 1H). 13C NMR (100 MHz, DMSO) δ 155.5, 134.6, 132.6, 131.2, 129.1, 128.7, 127.9, 118.6, 118.5, 118.3.
2-(2-Methoxyphenyl)-5-phenyl-1,3,4-oxadiazole. Mp 118–120 °C; 1H NMR (400 MHz, DMSO-d6) δ 7.60 (d, J = 6.4 Hz, 2H), 7.44 (t, J = 7.8 Hz, 2H), 7.20 (t, J = 7.8 Hz, 2H), 7.13 (d, J = 8.3 Hz, 1H), 7.07 (t, J = 7.5 Hz, 1H), 6.74 (t, J = 7.3 Hz, 1H), 3.87 (s, 3H). 13C NMR (101 MHz, DMSO) δ 156.4, 145.9, 130.5, 129.0, 128.4, 120.6, 119.3, 118.1, 113.6, 113.6, 111.5, 55.7.
2-(4-Fluorophenyl)-5-phenyl-1,3,4-oxadiazole. Mp 150–151 °C; 1H NMR (400 MHz, DMSO-d6) δ 8.64 (t, J = 5.6 Hz, 2H), 8.33 (t, J = 8.7 Hz, 2H), 8.19 (t, J = 7.7 Hz, 2H), 7.84 (d, J = 7.9 Hz, 1H), 7.75 (t, J = 7.3 Hz, 1H), 7.70 (d, J = 9.6 Hz, 1H). 13C NMR (101 MHz, DMSO-d6) δ 163.3, 160.9, 145.7, 129.0, 119.4, 116.4, 115.9, 115.6, 113.8.
3. Results and discussion
3.1. Characterization of the catalyst
The synthesized Alg–PSSA-co-ACA@Ni catalyst was fully characterized using Fourier transform infrared spectroscopy (FT-IR), thermogravimetric analysis (TGA), inductively coupled plasma mass spectrometry (ICP-MS), field emission scanning electron microscopy with energy dispersive X-ray spectroscopy (FESEM-EDX) mapping techniques and N2 isotherms. The FT-IR spectrum of sodium alginate, SiO2@Alg–PSSA-co-ACA, mesoporous Alg–PSSA-co-ACA, and Alg–PSSA-co-ACA@Ni is shown in Fig. 1. The results obtained from the FT-IR spectrum show: (i) the presence of the SiO2 NPs template; (ii) the polymerization of PSSA and crosslinking of the MBA groups; (iii) the selective removal of the SiO2 NPs from SiO2@Alg–PSSA-co-ACA; and (iv) the interaction of Ni NPs with the prepared support. The FT-IR spectrum of NaAlg displayed vibrational bands at 1642 and 1454 cm−1 (representing the vibrations of the carboxylate anions). The vibrational bands at 3412 and 2929.87 cm−1 can be attributed to the O–H and C–H stretching, respectively (Fig. 1A).17 The FT-IR spectrum of SiO2@Alg–PSSA-co-ACA displayed new bands at 3363, 3090 and 1662 cm−1 that can be attributed to the O–H, C–H and C
O amide stretching bands, respectively. The presence of these peaks confirmed the successful crosslinking of MBA. In addition, the presence of new bands at 1328 and 1159 cm−1 (owing to the O
S
O stretching) confirmed the successful polymerization of PSSA.35 Also, the peaks at 1180 cm−1 proved the presence of the SiO2 NPs (Fig. 1B).36 After the silica etching procedure, this peak was not observed in the FT-IR spectra of the porous Alg–PSSA-co-ACA and Alg–PBSA-co-ACA@Ni (Fig. 1C and D). In the FT-IR spectrum of Alg–PSSA-co-ACA@Ni (Fig. 1D), after interaction of the Ni NPs with the prepared support, the band at 3392 cm−1 (resulting from the NH2 stretching, Fig. 3C) shifted to lower wave number (3363 cm−1). In addition, the amide peaks shifted from 1633 to 1600 cm−1. Based on the strong co-ordination of the Ni NPs with the composite, the IR absorption peaks of the organo-functions are slightly shifted to the lower region.
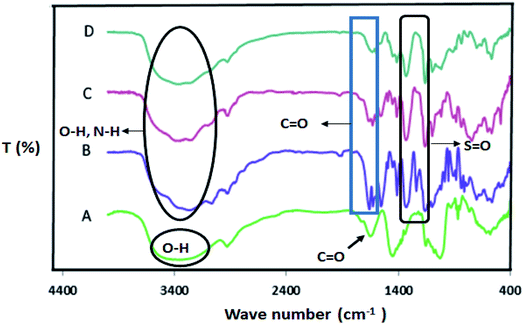 |
| Fig. 1 FT-IR spectra of NaAlg (A), SiO2@Alg–PBSA-co-ACA (B), porous Alg–PSSA-co-ACA (C), and Alg–PSSA-co-ACA@Ni (D). | |
Fig. 2 shows the X-ray diffraction pattern of the porous Alg–PSSA-co-ACA@Ni samples before (Fig. 2A) and after the reaction (Fig. 2B). Comparison of X-ray diffractometry (XRD) patterns of the Alg–PSSA-co-ACA@Ni sample shows that the structure of the prepared catalyst did not changed significantly after the reaction. The diffraction peaks appeared at 2θ = 60° and can be attributed to the Ni NPs.37 Meanwhile, in the XRD pattern of the Alg–PSSA-co-ACA@Ni nanocomposite sample, an amorphous phase is observed, which is related to the presence of amorphous polymer filaments of acrylamide (2θ = 20–30 degrees),38 and sodium alginate (2θ = 35–40 degrees),39 and polystyrene sulfonamide (2θ = 20–30 degrees).40 It is worth noting that the crystal structure of the recovered catalyst sample is quite similar to that of the original catalyst structure and the peak positions of the peaks have only changed a little, indicating the stability of the recovered catalyst. Therefore, the prepared catalyst can be reused several times after a simple recovery period.
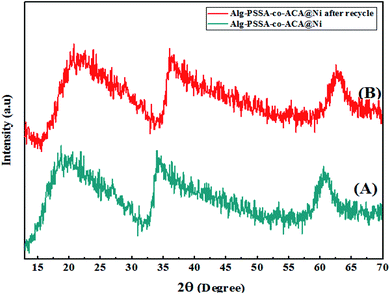 |
| Fig. 2 XRD patterns of porous Alg–PSSA-co-ACA@Ni before (A) and after (B) the reaction. | |
The morphology of the SiO2 NPs, mesoporous Alg–PSSA-co-ACA and Alg–PSSA-co-ACA@Ni was characterized using FE-SEM analysis (Fig. 3). The FE-SEM images of the spherical SiO2 NPs showed a uniform distribution (Fig. 3A). The FE-SEM image of Alg–PSSA-co-ACA obtained using the silica template method demonstrated the mesoporous structure of the Alg–PSSA-co-ACA (Fig. 3B). Fig. 3B shows the rough morphology of the surface, which results in the increased activity of the catalyst. Fig. 3C and D show the distribution of the spherically shaped Ni particles on the surface of the mesoporous Alg–PSSA-co-ACA.
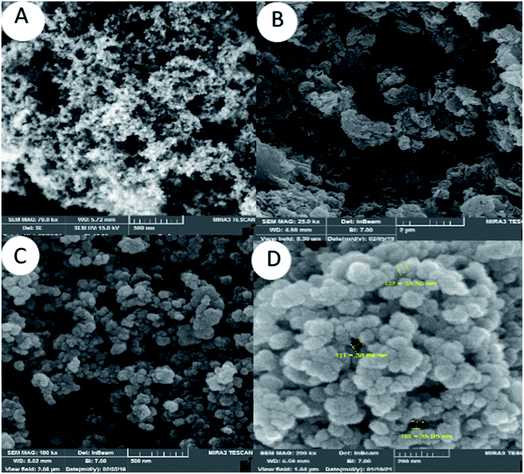 |
| Fig. 3 FE-SEM photographs of SiO2NPs (A), mesoporous Alg–PSSA-co-ACA (B), and mesoporous Alg–PSSA-co-ACA@Ni (C and D). | |
The high-resolution transmission electron microscopy (HR-TEM) images of the Alg–PSSA-co-ACA@Ni (Fig. 4A) and the recovered catalyst (Fig. 4B) are depicted in Fig. 4. The HR-TEM images showed that the sample has uniform particles that are approximately 20 nm in size. The good dispersion of the Ni species was visible at higher magnifications in the HR-TEM image, a very thin layer of the porous polymer could be detected around the Ni NPs. In the prepared catalyst, the porous Alg–PSSA-co-ACA can play the role of both reducing and capping agent. In addition, the deposition of the Ni NPs was verified using EDX analysis. The HR-TEM image demonstrated the presence of Ni NPs along with the polymer in the catalyst without any noticeable change compared with that of the original one. In comparison with the fresh particles (Fig. 4A), the number of Ni NPs distributed on the mesoporous Alg–PSSA-co-ACA substrate seems to be reduced. This may be the reason for the reduction in the catalytic performance observed after recycling seven times. To obtain further confirmation for this claim, ICP-OES was performed on a sample of the supernatant after the particles were used for the seventh time, and it was revealed that 1.55 mmol g−1 of the Ni element existed in the sample. This means that the leaching of the Ni element from the catalytic system is an inevitable event, but this partial leaching does not have any significant effect on the catalytic performance.
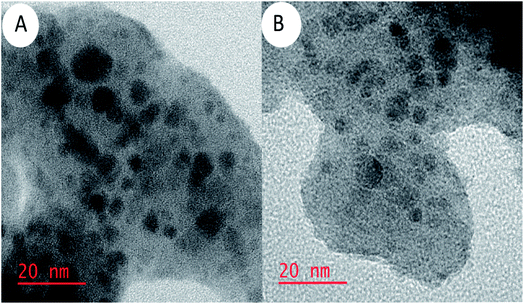 |
| Fig. 4 HR-TEM photographs of mesoporous Alg–PSSA-co-ACA@Ni before (A) and after (B) the reaction. | |
The elemental composition of the Alg–PSSA-co-ACA@Ni was determined using energy dispersive X-ray spectroscopy (EDS) analysis and the presence of Na, O, S, C, N and Ni was confirmed (Fig. 5). From the weight percentage results of the EDS analysis of the prepared catalyst (Table 1), it can be understood that the successful polymerization of para-styrene sulfonamide (S: 30.41%) has been achieved. The presence of N (9.28%) and O (26.10) also confirmed the crosslinking of MBA and the polymerization of PSSA (Table 1). In addition, the elemental mapping exhibited the uniform distribution of all the elements, as shown in Fig. 6. In addition, the proper dispersion of the Ni NPs in the composite is obvious.
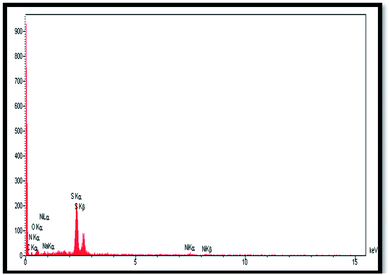 |
| Fig. 5 The EDX spectrum of Alg–PSSA-co-ACA@Ni. | |
Table 1 Elemental percentages of Alg–PSSA-co-ACA@Ni
Element |
W% |
A% |
C |
28.34 |
42.36 |
N |
9.28 |
6.76 |
O |
26.10 |
29.28 |
Na |
1.13 |
0.88 |
S |
30.41 |
19.27 |
Ni |
4.74 |
1.45 |
Total |
100.0 |
|
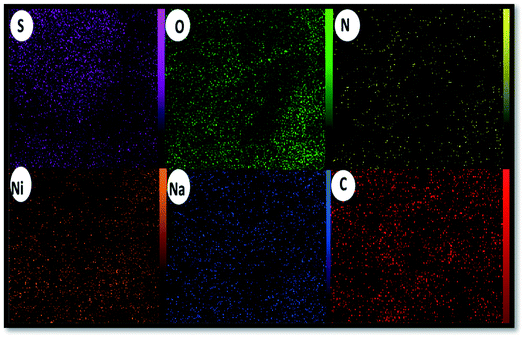 |
| Fig. 6 Elemental mapping of C, N, O, S, Ni, and Na atoms achieved from the SEM micrographs. | |
Fig. 7 shows the TGA curve of Alg–PSSA-co-ACA@Ni, in which a small weight loss from 30 to 100 °C can be observed, clearly related to the physically absorbed water. After this, the weight loss in the range of 230–320 °C clearly indicated the degradation of the Alg–PSSA-co-ACA groups.41
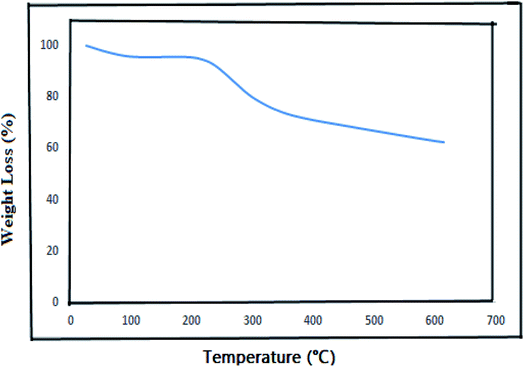 |
| Fig. 7 The TGA curve for Alg–PSSA-co-ACA@Ni. | |
As shown in Fig. 8, according to the Brunauer–Emmett–Teller (BET) analysis, Alg–PSSA-co-ACA (Fig. 8A) and Alg–PSSA-co-ACA@Ni (Fig. 8B) showed a typical type IV isotherm with type H3 hysteresis (defined by IUPAC).42 The results of the N2-adsorption and desorption isotherms of Alg–PSSA-co-ACA and Alg–PSSA-co-ACA@Ni clearly demonstrated the immobilization of Ni NPs significantly reduces the pore diameter, pore volume and specific surface area (Table 2).
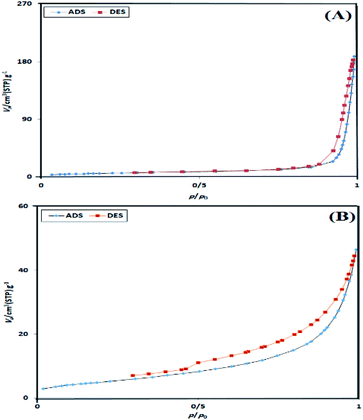 |
| Fig. 8 N2 adsorption–desorption isotherms of porous Alg–PSSA-co-ACA (A) and Alg–PSSA-co-ACA@Ni (B). | |
Table 2 Textural parameters obtained from nitrogen adsorption studies
Sample |
SBET (m2 g−1) |
Pore diameter obtained using the BJH method (nm) |
Pore volume (cm3 g−1) |
Alg–PSSA-co-ACA |
22.41 |
25.55 |
0.27 |
Alg–PSSA-co-ACA@Ni |
21.30 |
1.88 |
0.07 |
The N2 adsorption–desorption isotherms of the seventh reused catalyst (Fig. 9) were measured in order to determine the textural properties. It can be seen from Fig. 9 that the reused catalyst shows a typical type IV isotherm with type H3 hysteresis (defined by IUPAC) and is identified as a mesoporous material.42 The N2 adsorption–desorption isotherms of the reused catalyst indicate that there is no obvious change in the catalytic composition after the reaction. The changes associated with the textural properties of the catalyst when reused for the seventh time could be due to the fact that the reactants were distributed inside the Alg–PSSA-co-ACA@Ni pores during the reaction (pore volume = 0.05 cm3 g−1, SBET = 6.41 m2 g−1).
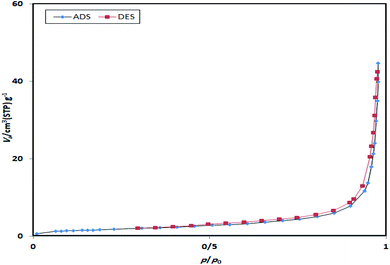 |
| Fig. 9 N2 adsorption–desorption isotherms of the recovered Alg–PSSA-co-ACA@Ni. | |
3.2. Catalytic tests
The catalytic activity of Alg–PSSA-co-ACA@Ni in the model reaction (iodobenzene, benzohydrazide and tert-butyl isocyanide) is presented in Table 3. At first, the protocol was optimized for the amount of the Alg–PSSA-co-ACA@Ni catalyst. As the amount of catalyst increased (entries 1 and 2), the yield of the product increased rapidly in 1.5 mol% of the prepared catalyst (entry 2) but after the reaction it was independent of the amount of catalyst (entry 4). Then, the reaction was checked at several different temperatures, and in the presence of bases and various solvents. According to the catalytic results in different solvents (entries 4–8), in a mixture of EtOH
:
H2O (1
:
1) the highest reactivity was observed. For optimization of the temperature (entry 9), the best result was observed under reflux conditions. Afterwards, by investigating the effect of bases on the reaction progress, it was observed that the reaction reached a 94% yield after 1 h with KHCO3. Other inorganic and organic bases also showed good yields (NaOAc, NaOH, K2CO3, KHCO3 and Et3N). However, in the absence of a base, the yield was very low (entry 10). According to Table 1, the optimized reaction conditions are Alg–PSSA-co-ACA@Ni (1.5 mol%, 9 mg) using 1 mmol of KHCO3 as the base in H2O
:
EtOH (2 mL, 1
:
1) under reflux conditions (entry 3). As shown in Table 3, to reveal the importance of the porous Alg–PSSA-co-ACA in the catalyst, the Ni NPs only were also applied under the same conditions. As can be detected in Table 3 (entry 11), the obtained reaction yield was reduced after removing the porous Alg–PSSA-co-ACA from the catalytic system. Moreover, as the Ni NPs are the main catalytic site for this catalytic system it is estimated that by removing the Ni NPs from the catalytic system, the synthesis of the desired product did not occur (entries 12 and 13). On the other hand, the influence of NiCl2 was also investigated and a low yield was obtained (entry 14). It is worth noting that a similar reaction in the presence of NiCl2 and polysulfonamide with sodium borohydride failed to proceed.
Table 3 Optimization of the reaction conditionsa
To investigate the effect of the para-styrene sulfonamide ligand, another porous support was prepared in the absence of the para-styrene sulfonamide ligand, and the Ni NPs were immobilized onto the surface of the porous Alg–ACA (Table 4). The same model reaction was performed using the Alg–ACA@Ni catalyst and the results showed that in the absence of poly styrene sulfonamide the reaction did not precede very well and only 58% of the product was obtained. This reveals that polystyrene sulfonamide on the surface of the catalyst significantly improved the catalytic activity of the immobilized Ni owing to the strong chelation of the supported ligand. Moreover, we also compared the catalytic performance of the Ni NPs immobilized on sodium alginate, porous Alg–ACA, PSSA-co-ACA and porous Alg–PSSA-co-ACA supports in both the fresh and recycled state under the same conditions (Table 4). The results show that the porous Alg–PSSA-co-ACA@Ni catalyst, in comparison with the others, is very suitable and efficient, leading to the preparation of 94% and 84% (after the 7th cycle) of the product in the fresh and recycled states, respectively, under the same conditions. In addition, as seen in Table 4, the presence of sodium alginate, acryl amide and polysulfonamide in the support matrix seems to be necessary for the further chemical stability of the catalyst compared to others in the recycled form, because after several runs, the amount of decomposition reduces, and the activity of the catalyst increases. To investigate the effect of chemical modification on the chelation between sodium alginate and Ni NPs, the loading amount of Ni was also characterized using ICP-OES (0.52 mmol g−1) and it was observed that the loading of Ni had decreased. Thus, the significant role of the sodium alginate surface modification in the effective chelation of nickel NPs to the surface was proved.
Table 4 A comparison study of the catalytic efficiency of a variety of catalysts with different surface modifications
Catalyst |
Yield of run 1 (%) |
Yield of run 7 (%) |
Time (h) |
Alg@Ni |
53 |
— |
1 |
Alg–ACA@Ni |
58 |
52 |
1 |
PSSA-co-ACA@Ni |
90 |
75 |
1 |
Alg–PSSA-co-ACA@Ni |
94 |
84 |
1 |
After stabilizing the reaction conditions, as discussed in Table 5, the next endeavour was to ascertain their scope and generality over a broad range of substrates (Tables 5 and 6). We found that a variety of functionalized 1,3,4-oxadiazoles are accessible in good to excellent yields using this method. With regard to the scope of the aryl iodides, electron-withdrawing substituted aryl iodides such as p-Br, o-Br, m-Br, p-chloro, m-chloro, o-chloro, p-F, 2,3-Cl2 and p-COOH (4b–j), electron-donating aryl iodides such as p-OCH3, o-CH3, m-OCH3, p-CH3 (4k–n) and sterically hindered aryl iodides (4c, 4f, 4i, 4k) are all good substrates. Iodobenzenes bearing electron-donating substituents gave the desired products in better yields as compared to electron-withdrawing aryl iodides. Moreover, this condition was assessed using a wide range of aryl iodides containing substituents in the ortho position (e.g., OMe, Cl, Br) (4c, 4f, 4k), leading to moderate yields (Table 5).
Table 5 The substrate scope for the preparation of 1,3,4-oxadiazoles using different iodobenzenesa
Table 6 Substrate scope for the preparation of 1,3,4-oxadiazoles using different benzhydrazides, iodobenzenes, and tert-butyl isocyanide under the optimized conditionsa
The current study was extended to benzhydrazide derivatives using the optimized reaction conditions (Table 6). It was observed that various substituted benzhydrazides, including 2-phenylacetohydrazide (5a–b), benzhydrazides carrying –NH2, –CH3, and –OCH3 (5c–f), and electron-withdrawing substituents such as pyridine, –Cl, –Br and formyl (5g–k) reacted successfully in good yields under the set reaction conditions. The yields for the benzhydrazide group are higher than those obtained for the electron-poor ones, probably owing to the stronger nucleophilicity. Interestingly, the reaction of the unreactive hydrazides with the unreactive aryl iodide led to the desired product in a good yield (5l–p). It should be noted that the corresponding products were synthesized in good yields with the isomeric electron-deficient aryliodide: o-, m-, and p-chloro iodobenzene (5n–p). The longest reaction time was observed for the o-chloro benzhydrazide because of the electron-withdrawing effect of the chloro group and also the anchimeric resistance (steric hindrance). Moreover, the desired product was synthesized in good yields using acetohydrazide, p-toluoylhydrazide and m-anisohydrazide with p-bromoiodobenzene (5q–s). It is noteworthy that the reaction is highly regioselective as no regioisomeric chloro or bromo cyclization product could be detected. The products were characterized using physical and spectral (infrared spectroscopy, NMR, and mass spectrometry) data.
As revealed from the previously published literature and according to Table 6, in order to synthesize 2,5-diphenyl-1,3,4-oxadiazole, different conditions have been investigated (entries 1–4). As shown in this table and using the previously described method, all of the requested products were obtained in high yields during short reaction times (entry 5). The catalyst is an eco-friendly material owing to the fact that it is composed of sodium alginate and poly (p-styrene sulfonamide). Moreover, as evident from Table 7, when polystyrene sulfonamide and sodium alginate are present in the support matrix, they enable the greater chemical stability of the catalyst owing to the fact that even after several runs the catalyst activity is not significantly decreased (yields of 94%, 94%, 92%, 90%, 88%, 87% and 84% are observed).
Table 7 Comparison of the present methodology with other reported catalysts for the synthesis of 2,5-diphenyl-1,3,4-oxadiazole (4a)
Entry |
Conditions |
Yield (%) [ref.] |
1 |
PdCl2 (0.0125 mmol), DPPP (0.025 mmol), NaOAc (1 mmol), DMF, 130 °C, 3 h |
76 (ref. 10) |
2 |
Cu(OTf)2 (10 mol%), CsCO3, DMF, 110 °C, N-arylidenearoylhydrazide (1 mmol), 16 h |
85 (ref. 7) |
3 |
N-acyl-N′-aryliden-hydrazines (0.5 mmol), Dess–Martin periodinane (0.5 g), CH2Cl2, 4 h |
92 (ref. 43) |
4 |
Benzaldehyde (1 mmol), benzhydrazide (1 mmol), I2 (1.2 mmol), K2CO3 (3 mmol), EtOH, reflux temperature, 3 h |
76 (ref. 6) |
5 |
Alg–PBSA-co-ACA@Ni (1.5 mol%, 9 mg), KHCO3 (1 mmol), H2O : EtOH, reflux conditions, 1 h |
94 [this work] |
A plausible reaction pathway has been suggested in Scheme 3. The oxidative addition of aryl halides to the Ni(0) catalyst facilitates the formation of the palladium complex A, followed by insertion to obtain the nickel(II) species B. Then, benzhydrazides are added to intermediate B, with the assistance of KHCO3, to form intermediate C. Finally, owing to the loss of the tert-butylamine and the cyclization of intermediate D, the desired product is produced (Scheme 3).10
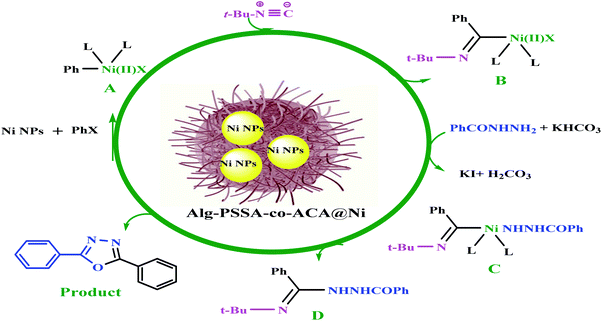 |
| Scheme 3 The suggested mechanism for the synthesis of 1,3,4-oxadiazoles using Alg–PSSA-co-ACA@Ni. | |
3.3. Reusability study
As the reusability of any catalyst is a key factor for determining its commercial applicability, the reusability and recovery of the catalyst were tested by studying the reaction of iodobenzene, benzohydrazide and tert-butyl isocyanide, as model substrates. As expected, the catalyst illustrated a desirable reusability with a negligible reduction in its activity (Fig. 10, yields of 94%, 92%, 90%, 88%, 87% and 84 are observed). It could be separated from the reaction mixture by centrifugation, and was then washed with ethanol, and reused in the forthcoming catalytic reactions. In this research, we also report the amount of Ni that has leached from the NPs in the model reaction by checking the Ni loading amount before and after the recycling of the catalyst using the ICP-OES technique. It was observed that the amount of Ni in the fresh catalyst and the recycled catalyst after recycling seven times was 1.60 and 1.55 mmol g−1, respectively. Therefore, it can be stated that the nickel species remain active in the catalytic procedure owing to the excellent complexation of the amide, sulfonamide, carboxylate and hydroxyl groups. This result also demonstrated that the amount of nickel that leached from the catalyst was low.
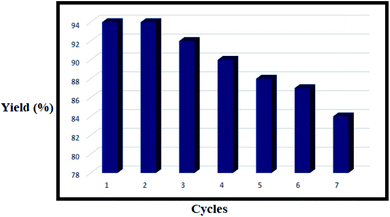 |
| Fig. 10 Recycling of Alg–PSSA-co-ACA@Ni for the synthesis of 2,5-diphenyl-1,3,4-oxadiazole (4a). | |
4. Conclusions
The purpose of the current study was to design a novel catalyst consisting of Ni decorated on a porous support containing Alg–PSSA–ACA and to evaluate its catalytic activity for the green synthesis of 1,3,4-oxadiazoles. Alg–PSSA-co-ACA@Ni has been developed as a highly active and recoverable heterogeneous catalyst for the preparation of diverse functionalized 1,3,4-oxadiazoles. The results illustrate that the amphiphilicity and high metal loading result in the high performance of the catalyst. Hence, because of these great features, we recommend Alg–PSSA-co-ACA@Ni as a robust and promising candidate for this important type of chemical conversion.
Conflicts of interest
The authors listed in this article have no conflict of interests.
Acknowledgements
The authors wish to thank Bu-Ali Sina University, Center of Excellence Developmental of Environmentally Friendly Methods for Chemical Synthesis (CEDEFMCS) and Iran National Science Foundation (INSF) for financial support in carrying out this research.
References
- H. Khalilullah, M. J. Ahsan, M. Hedaitullah, S. Khan and B. Ahmed, 1,3,4-Oxadiazole: a biologically active scaffold, Mini-Rev. Med. Chem., 2012, 12, 789–801 CrossRef CAS PubMed.
- N. D. James and J. W. Growcott, Zibotentan endothelin ETA receptor antagonist oncolytic, Drugs Future, 2009, 34, 624–633 CAS.
- V. Summa, A. Petrocchi, F. Bonelli, B. Crescenzi, M. Donghi, M. Ferrara, F. Iore, C. Gardelli, O. Gonzalez Paz, D. J. Hazuda, P. Jones, O. Kinzel, R. Laufer, E. Monteagudo, E. Muraglia, E. Nizi, F. Orvieto, P. Pace, G. Pescatore, R. Scarpelli, K. Stillmock, M. V. Witmer and M. Rowley, Discovery of raltegravir, a potent, J. Med. Chem., 2008, 51, 5843–5855 CrossRef CAS PubMed.
- Q. H. Gao, S. Liu, X. Wu, J. J. Zhang and A. X. Wu, Org. Lett., 2015, 17, 2960–2963 CrossRef CAS PubMed.
- M. Al-Talib, H. Tashtoush and N. Odeh, Synth. Commun., 1990, 20, 1811–1817 CrossRef CAS.
- G. Majji, S. K. Rout, S. Guin, A. Gogoi and B. K. Patel, RSC Adv., 2014, 4, 5357–5362 RSC.
- S. Guin, T. Ghosh, S. K. Rout, A. Banerjee and B. K. Patel, Org. Lett., 2011, 13, 5976–5979 CrossRef CAS PubMed.
- T. Kawano, T. Yoshizumi, K. Hirano, T. Satoh and M. Miura, Org. Lett., 2009, 11, 3072–3075 CrossRef CAS PubMed.
- C. Xu, F. C. Jia, Q. Cai, D. K. Li, Z. W. Zhou and A. X. Wu, Chem. Commun., 2015, 51, 6629–6632 RSC.
- X.-Y. Fan, X. Jiang, Y. Zhang, Z.-B. Chen and Y.-M. Zhu, Org. Biomol. Chem., 2015, 13, 10402–10408 RSC.
- R. Poupart, D. Grande, B. Carbonnier and B. Droumaguet, Prog. Polym. Sci., 2019, 96, 21–42 CrossRef CAS.
- Z. B. Shifrina, V. G. Matveeva and L. M. Bronstein, Chem. Rev., 2020, 120, 1350–1396 CrossRef CAS PubMed.
- S. Alizadeh and D. Nematollahi, J. Am. Chem. Soc., 2017, 139, 4753–4761 CrossRef CAS PubMed.
- S. Babaee, M. Zarei, M. A. Zolfigol, S. Khazalpour, M. Hasani, U. Rinner, R. Schirhagl, N. Norouzi and S. Rostamnia, RSC Adv., 2021, 11, 2141–2157 RSC.
- M. Zhang, L. He, T. Shi and R. Zha, Chem. Mater., 2018, 30, 7391–7412 CrossRef CAS.
- K. Kim, S. Baek, J. Rho and H. Lee, ACS Appl. Mater. Interfaces, 2019, 11, 26109–26115 CrossRef CAS PubMed.
- S. Khalid, G. Abbas, M. Hanif, S. Shah, S. Nisar, H. Shah, A. Jalil, M. Yaqoob, N. Ameer and A. Anum, Int. J. Biol. Macromol., 2020, 164, 2691–2700 CrossRef CAS PubMed.
- H. Veisi, Z. Joshani, B. Karmakar, T. Tamoradi, M. M. Heravi and J. Gholami, Int. J. Biol. Macromol., 2021, 172, 104–113 CrossRef CAS PubMed.
- R. Ghorbani-Vaghei, H. Veisi, M. HajiAliani, P. Mohammadi and B. Karmakar, J. Mol. Liq., 2020, 327, 114868–114876 CrossRef.
- F. Bayat, A. R. Karimi and T. Adimi, Int. J. Biol. Macromol., 2020, 159, 598–606 CrossRef CAS PubMed.
- A. Maleki, R. Firouzi-Haji and Z. Hajizadeh, Int. J. Biol. Macromol., 2018, 116, 320–326 CrossRef CAS PubMed.
- A. Maleki, V. Eskandarpour, J. Rahimi and N. Hamidi, Carbohydr. Polym., 2019, 208, 251–260 CrossRef CAS PubMed.
- H. V. Saether, H. K. Holme, G. Maurstad, O. SmidsrØd and B. T. Stokke, Carbohydr. Polym., 2008, 74, 813–821 CrossRef CAS.
- S. Hua and A. Wang, Carbohydr. Polym., 2009, 75, 79–84 CrossRef CAS.
- L. Yang, J. Guo, J. Wu, Y. Yang, S. Zhang, J. Song, Q. An and Y. Gong, RSC Adv., 2017, 7, 50626–50633 RSC.
- N. Işıklan and F. Kurşun, Polym. Bull., 2013, 70, 1065–1084 CrossRef.
- M. M. Soledad Lencina, Z. Iatridi, M. A. Villar and C. Tsitsilianis, Eur. Polym. J., 2014, 61, 33–44 CrossRef CAS.
- Y. Kitamura, M. Kandeel, T. Kondo, A. Tanaka, Y. Makino, N. Miyamoto, A. Shibata, M. Ikeda and Y. Kitade, Bioorg. Med. Chem. Lett., 2020, 30, 127637–127657 CrossRef CAS PubMed.
-
(a) S. Alavinia and R. Ghorbani-Vaghei, J. Phys. Chem. Solids, 2020, 146, 109573–109584 CrossRef CAS;
(b) F. Hamidi Dastjerdi, R. Ghorbani-Vaghei and S. Alavinia, Catal. Lett., 2020, 150, 3514–3522 CrossRef CAS;
(c) S. Alavinia, R. Ghorbani-Vaghei, J. Rakhtshah, J. Yousefi Seyf and I. Ali Arabian, Appl. Organomet. Chem., 2020, 34, e5449 CrossRef CAS;
(d) S. Alavinia and R. Ghorbani-Vaghei, New J. Chem., 2020, 44, 13062–13073 RSC;
(e) S. Solgi, R. Ghorbani-Vaghei and S. Alavinia, J. Porous Mater., 2021, 28, 289–298 CrossRef CAS;
(f) N. Shekarlab, R. Ghorbani-Vaghei and S. Alavinia, J. Organomet. Chem., 2021, 949, 121971 CrossRef CAS;
(g) A. Rahimi, R. Ghorbani-Vaghei and S. Alavinia, J. Porous Mater., 2021, 1–11, DOI:10.1007/s10934-021-01104-1;
(h) S. Zafari, R. Ghorbani-Vaghei and S. Alavinia, Mater. Chem. Phys., 2021, 270, 124840 CrossRef CAS;
(i) N. Shekarlab, R. Ghorbani-Vaghei and S. Alavinia, Appl. Organomet. Chem., 2020, 34, e5918 CrossRef CAS.
-
(a) K. Katagiri, T. Sakai, M. Hishikawa, H. Masu, M. Tominaga, K. Yamaguchi and I. Azumaya, Cryst. Growth Des., 2014, 14, 199–206 CrossRef CAS;
(b) M. Roca, R. L. Althaus and M. P. Molina, Food Chem., 2013, 136, 376–383 CrossRef CAS PubMed.
- R. Ghorbani-Vaghei and N. Sarmast, Can. J. Chem., 2017, 95, 1073–1084 CrossRef CAS.
-
(a) R. Ghorbani-Vaghei, S. Alavinia, Z. Merati and V. Izadkhah, Appl. Organomet. Chem., 2018, 32, e4127 CrossRef;
(b) A. Fatehi, R. Ghorbani-Vaghei, S. Alavinia and J. Mahmoodi, ChemistrySelect, 2020, 5, 944 CrossRef CAS;
(c) R. Ghorbani-Vaghei, S. Alavinia and N. Sarmast, Appl. Organomet. Chem., 2018, 32, e4038 Search PubMed.
- W. Stöberand and A. Fink, J. Colloid Interface Sci., 1968, 26, 62–69 CrossRef.
- S. Khan, A. Ghatak and S. Bhar, Tetrahedron Lett., 2015, 56, 2480–2487 CrossRef CAS.
- A. G. Ibrahim, A. Z. Sayed, H. A. El-Wahab and M. M. Sayah, Am. J. Polym. Sci. Technol., 2019, 5, 55–62 CrossRef.
- J. Lin, H. Huang, M. Wang and J. Deng, Polym. Chem., 2016, 7, 1675–1681 RSC.
- M. R. Ahghari, V. Soltaninejad and A. Maleki, Sci. Rep., 2020, 10, 12627 CrossRef CAS PubMed.
- T. A. Saleh, A. M. Elsharif, S. Asiri, A. R. Mohammed and H. Dafalla, Environ. Nanotechnol. Monit. Manag., 2020, 14, 100302 Search PubMed.
- A. Ahmed, F. Mohamed, A. M. Elzanaty and O. F. Abdel-Gawad, Int. J. Biol. Macromol., 2021, 167, 766–776 CrossRef CAS PubMed.
- L. Jia, D. Wang, L. Liu, S. Zhang and T. Xu, Des. Monomers Polym., 2014, 17, 425–429 CrossRef CAS.
- Z. Bahrami, A. Akbari and B. Eftekhari-Sis, Int. J. Biol. Macromol., 2019, 129, 187–197 CrossRef CAS PubMed.
- S. W. Sing and W. Kenneth, Adsorpt. Sci. Technol., 2004, 22, 773–782 CrossRef.
- C. Dobrotă, C. C. Paraschivescu, I. Dumitru, M. Matache, I. Baciu and L. L. Ruţă, Tetrahedron Lett., 2009, 50, 1886–1888 CrossRef.
Footnote |
† Electronic supplementary information (ESI) available: Detailed experimental procedure and FT-IR and MS analysis of all compounds. See DOI: 10.1039/d1ra04022g |
|
This journal is © The Royal Society of Chemistry 2021 |
Click here to see how this site uses Cookies. View our privacy policy here.