DOI:
10.1039/D1RA03815J
(Paper)
RSC Adv., 2021,
11, 24536-24542
Hydrothermal green synthesis of MoS2 nanosheets for pollution abatement and antifungal applications†
Received
16th May 2021
, Accepted 8th July 2021
First published on 13th July 2021
Abstract
In this study, we report a green synthesis of MoS2 nanosheets (NSs) using a facile hydrothermal technique in the presence of L-cysteine. L-Cysteine can serve as a greener source of sulfur as well as a capping agent to help the growth of MoS2 nanosheets. The prepared materials were characterized by X-ray powder diffraction (XRD), scanning electron microscopy (SEM) with energy dispersive spectroscopy (EDS), electron transmission microscopy (TEM), X-ray photoelectron microscopy (XPS), and Brunauer, Emmett, and Teller (BET) analysis. The results showed that MoS2 NSs are of high crystallinity with a lattice spacing of 0.61 nm. The optical bandgap of MoS2 NSs nanosheets prepared using L-cysteine as a source of sulfur was found to be 1.79 eV. The photocatalytic degradation of MoS2 NSs towards methylene orange (MO) and rhodamine blue (RB) dyes under sunlight was found to be promising for practical applications. The fast kinetics of degradation of MO and RhB was observed over a wide range of pH range. Moreover, MoS2 NSs showed excellent antifungal activities against Trichophyton mentagrophytes and Penicillium chrysogenum fungus.
1. Introduction
During the last decades, the rapid increase in industrialization and human population has brought a multitude of contaminants to the aquatic environment. Synthetic organic dyes from dyeing industries make a substantial contribution.1 Due to this millions of people have been dying every year because of water pollution.2 Therefore, it is a prime concern to reduce or remove pollutants from their point source before releasing them into the environment. Adsorption, Fenton-oxidation, flocculation, and photocatalysis techniques have been widely used to abate toxic effluents.3–5 Photocatalytic degradation due to their efficiency can degrade pollutants without leaving unwanted residues.6 TiO2 and ZnO in this regard play a significant role in the harvesting of light for the photocatalytic degradation of organic compounds.7 However, inefficient utilization of light in the visible region and high electron–hole recombination rate prohibit its practical applications. Searching or modification of photocatalysts that working efficiently in the visible region is the prime concern in this topic.8 Composite with different sensitizers, doping of different elements and morphology optimization are common strategies for the modification of photocatalysts.9 More recently, the combination of photocatalyst with persistent luminescent materials as inner secondary light sources is introduced to enhance the photocatalytic efficiency of semiconductors.10–12
Two-dimensional (2D) functional nanostructured materials, such as graphene, hexagonal boron nitride (hBN), graphitic carbon nitride (g-C3N4) and metal dichalcogenides (MX2), owing to their inherent physicochemical properties, including high specific surface-to-volume ratio, anisotropy, chemical inertness, better charge carrier separation, the rich option of host–guest species, and excellent tribological efficiency, have gotten a lot of attention for photocatalytic applications.13,14 Moreover, they are widely used in a variety of fields, such as lubricants, energy storage, electrocatalysis, magnetoresistance, organic catalysis, sensing, and field transit.14,15
Recently, MX2-based transition metal chalcogenides (TMDs) where M (= Mo, W, etc.) is a transition metal of group VI and X (= S, Se, etc.) is a chalcogen that has been received a lot of attention owing to unique electrical, optical and mechanical properties. Among 2D nanomaterials, MoS2 due to intriguing physicochemical properties shows an application in antibacterial,16,17 biomedical,18,19 transistors,20 photodetectors,21 and, solar cells.22 Bulk MoS2 is an indirect bandgap semiconductor. However, as the number of layers is reduced to a few, bilayers, and even monolayers, the bandgap becomes direct.23 Such direct bandgap semiconductor materials are suitable for harvesting light. Hence, the synthesis of MoS2 with a few layered structures is a prime concern for nanosheets for photocatalytic applications such as dye degradation and solar energy conversion.24,25
MoS2 NSs with enhanced properties have been synthesised by various techniques, including chemical vapour deposition (CVD),26 thermal reductions,27 hydrothermal,28 laser ablation,29 Liquid Phase Exfoliation (LPE),30,31 and sol–gel methods.32,33 Despite the quality of MoS2 NSs synthesized by the aforementioned techniques, but they require highly sophisticated equipment and commonly used environmental malignant reagents. Most of the synthesis methods were used organic solvents, and surfactants during the synthesis of MoS2 NSs, which introduce toxicity to the environment and human health. Therefore, searching for kind reagents for the preparation of MoS2 NSs should require considerable attention. Biomolecule-assisted synthetic pathways have been a promising technique in recent years because they are greener and having suitable chemistry for the preparation of nanomaterials.
Green synthesised nanostructured materials play a significant role in practical applications, including medicinal and environmental. Biological ways of synthesizing nanoparticles using microorganisms, enzymes, fungi, and plants or plant extracts are eco-friendly green synthesis alternatives to chemical and physical methods.18,19,34–36 In this regard, amino acids due to interesting aqueous chemistry are widely applicable as a reducing agent, capping agent and a source of dopant atoms during the synthesis of nanomaterials.37 Among these, L-cysteine is a sulphur-containing derivative resulting from the oxidation of the side chains of cysteine amino acid thiol. It functions as an antioxidant, shielding tissues from radiation and toxins and thereby slowing the ageing process. L-Cysteine also reported as a sulphur source for the preparation of MoS2. For example, 1D CNTs–MoS2 hybrid materials synthesised by hydrothermal method using L-cysteine as sulphur soured for anodized materials in lithium-ion batteries.38 Similarly, Veeramalai et al.39 reported MoS2 layered structure by the reduction of MoO3 using L-cysteine as a sulphur source. The synthesised MoS2 showed high field performance applications. Despite the potential of L-cysteine as a source of sulphur and capping agent to protect an irreversible agglomeration, there is no comprehensive report on the facile hydrothermal synthesis and photocatalytic investigation towards dye degradation of MoS2 NSs.
Hence, we aimed to synthesis MoS2 NSs using a facile hydrothermal method. (NH4)6Mo7O24·4H2O salt and L-cysteine are the only precursors for the preparation of MoS2 NSs. The electronic, crystal phase formation, composition and surface morphology of the synthesised MoS2 nanosheet powder was investigated by UV-Vis, UV-DRS, XRD, FT-IR, XPS, TEM/SAED, and FE-SEM/EDX. The Brunauer–Emmett–Teller (BET) method used to determine the surface area analysis. The photocatalytic activities MoS2 NSs was investigated by the degradation of the two stable organic dyes, such as rhodamine B (RhB) and methylene orange. These two dyes commonly found in industrial wastewater and are extremely toxic to human health due to their solubility, carcinogenicity, and teratogenicity. Moreover, the antifungal activity of MoS2 was studied against Penicillium chrysogenum and Trichophyton mentagrophytes fungus.
2. Experimental
2.1. Materials
All of the chemicals used in this analysis were analytical grade levels from Sigma-Aldrich, and Himedia. All chemicals were directly used without further purification. Specifically, ammonium molybdate tetrahydrate [(NH4)6Mo7O24·4H2O], and L-cysteine (C3H7NO2S) were obtained from Himedia, India. Methylene orange (MO), and rhodamine blue (RhB) were supplied from Sigma-Aldrich, India. The ultrapure water (Milli-Q) was used as a solvent throughout the whole experiment.
2.2. Nanosheet MoS2 synthesis
MoS2 NSs were synthesized by a facile and green hydrothermal synthesis approach using L-cysteine as a sulphur source. Particularly, 2.0 g (NH4)6Mo7O24·4H2O and 4.0 g L-cysteine were mixed in 50 mL deionized water. The solution was then sonicated for 30 minutes to create a clear solution. In a muffle furnace, the solution was poured into a 100 mL stainless steel autoclave and heated at 200 °C for 12 hours (Fig. 1). The autoclave was cooled to room temperature after the reaction had completed. Next, the black precipitate was centrifuged and thoroughly washed with water to remove unreacted residue and dried in the oven at 70 °C for 18 hours. The black powder, the as-synthesised MoS2 nanosheet, was kept for further use (ESI, Fig. 1†).
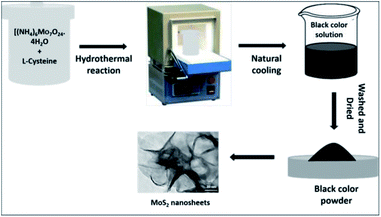 |
| Fig. 1 The schematic illustration for the hydrothermal synthesis of MoS2 NSs. | |
2.3. Characterizations
PANalytical Xpert Pro diffractometer with, Cu K1 = 0.154056 nm as a radiation source was used to record the X-ray diffraction (XRD) of MoS2 NSs. Transmission electron microscopy (TEM) images were recorded from FEI TECNAI G2 S-Twin, equipped with a 200 kV field emission gun (FEG). The field emission scanning electron microscopy (FE-SEM) images were obtained from JEOL, JSM-7600F SEM at an accelerated voltage of 0.1 to 30 kV equipped with X-ray dispersive spectroscopy (EDX). The chemical composition of the MoS2 NSs was detected by X-ray photoelectron spectroscopy (XPS, ESCALAB 250Xi). The Brunauer–Emmett–Teller (BET) (BELSORB-28SA/18SA/18PLUS) analyser studied the surface area and pore size from the N2 adsorption data.40,41
2.4. RhB and MO photocatalytic degradation
The photocatalytic activity of the MoS2 NSs towards the degradation of MO and RhB was investigated under natural sunlight irradiation. The adsorption–desorption equilibrium of dye was carried in the dark for 30 min before illumination. Specifically, 30 mg of MoS2 NSs was taken for 50 mL of 20 ppm dye solution. The sample was in dark to attain the adsorption–desorption and illuminate at midday (11 am to 3 pm) sunlight. At different time intervals, 3 mL of the solution took from the reaction vessel and centrifuged at 5000 rpm for 5 min to separate the catalyst. The residual concentration of MO and RhB in the solution was determined using a UV-visible spectrophotometer at λmax = 500 nm and 553 nm, respectively. The quantitative explanation of adsorption in the dark and degradation under sunlight was calculated by eqn (1) and (2) respectively. |
Degradation (%) = (C0 − Ct) × 100/C0 =
| (2) |
where q (mg g−1) the amount of MO adsorbed on the surface of MoS2 nanosheet; V (L) is the volume of the aqueous solution, m (g) is the mass of MoS2 nanosheet, C0 (mg L−1), the initial concentration of MO/RhB dye; Ct (mg L−1) the concentration of MO/RhB dye after adsorption/degradation. Furthermore, the antifungal activities of MoS2 NSs using Agar-Well diffusion method was further investigated against the T. mentagrophytes and P. chrysogenum fungus. The detailed protocol presents in ESI 1.†
3. Results and discussion
3.1. Characterizations of MoS2 nanosheets
The UV-Vis absorption spectrum of MoS2 NS was recorded to investigate the optical property. As shown in Fig. 2, the absorption edge of MoS2 NS extends to the visible region. Moreover, the optical bandgap energy of MoS2 NS was calculated from the UV-DRS spectrum (Fig. 3). The intrinsic direct bandgap of MoS2 NSs was found to 1.79 eV. This implies that the activation [formation of electron (e−) and holes (h+)] of MoS2 NSs is possible under the irradiation of the visible light region. Similar bandgap energy reported elsewhere.34
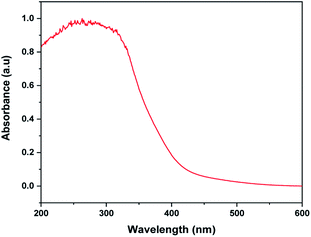 |
| Fig. 2 The UV-Vis absorption spectrum of MoS2 NSs. | |
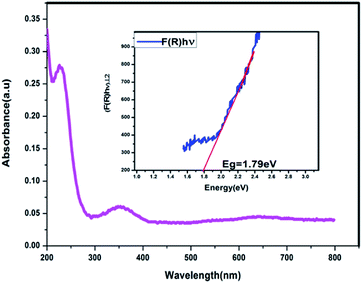 |
| Fig. 3 The UV-DRS absorption spectrum of MoS2 NSs. | |
Fig. 4 shows the XRD pattern for MoS2 NSs. The diffractogram confirms the formation of hexagonal MoS2 NSs from Mo2+ salt and L-cysteine as a sulfur source. The characteristic diffraction peak observed at 13.6°, 31.97°, 32.39°, 36.53°, 40.26°, 42.39°, and 48.94° which are indexed to (002), (100), (101), (102), (103), (106), and (105) planes of MoS2, respectively (JCPDS#: 00-037-1492).42 The broadened of the (002) peak and diffraction at a low angle (2θ: 13.6°) than bulk MoS2 (14.37°) shows the formation of few or single layers of MoS2 like graphene.43
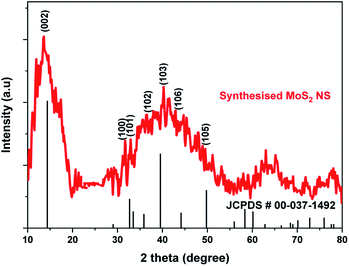 |
| Fig. 4 The XRD graph of MoS2 NSs. | |
The FTIR spectrum of the MoS2 NSs was recorded to investigate the composition of samples based on the vibrational properties (Fig. 5). The spectrum of MoS2 NSs shows the broad bands between 3748 and 3400 cm−1 attribute to the characteristics of O–H stretching of the intermolecular and intramolecular hydrogen bonds.44,45 The characteristic vibrational bands at 3300–2800 cm−1 and 1488 cm−1 can be assigned to the stretching of the C–H alkyl stretching band in L-cysteine.46 Thus, the FTIR results further affirmed the presence of residual amino acid on the surface of MoS2. The medium absorption bands observed at 756 cm−1, 1091 cm−1, and 1400 cm−1 are due to MoS2.47,48 Moreover, the band between 540 cm−1 and 400 cm−1 credit to the S–S bond vibrations.47
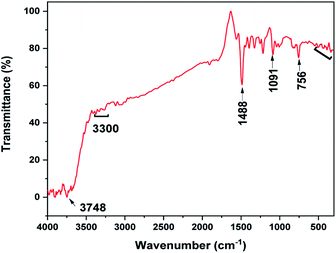 |
| Fig. 5 The FT-IR spectrum of MoS2 NSs. | |
The composition and chemical state of MoS2 NSs was studied by X-ray photoelectron spectroscopy (XPS). Fig. 6a presents the survey scan XPS spectrum of MoS2 NS. The spectrum depicts the presence of peaks due to C1s and O1s at 285.49 eV and 533.07 eV respectively. These are due to the residual L-cysteine capped onto the MoS2 surface. The high-resolution peaks of Mo, S, and O are shown in Fig. 6b–d. The peak binding energies of Mo and S are agreed well with the theoretical binding energies of the corresponding orbital electrons of Mo and S elements. Further, both the Mo 3d5/2 (227.48 eV) and the Mo 3d3/2 (230.63 eV) features presented in Fig. 6b are deconvoluted with only one function, indicating the presence of only one molybdenum chemical species at the surface.49,50 Moreover, the binding energy shows a shift as compared to the elemental Mo peaks affirms the formation of Mo4+ chemical state.39,51 Likewise, a similar analysis was done on sulfur, obtained characteristic peaks at the binding energy of 161.58 eV and 162.78 eV due to the S 2p3/2, and assigned to S 2p1/2, respectively.52,53
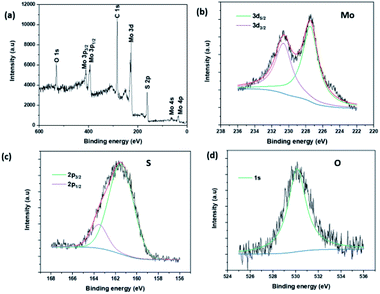 |
| Fig. 6 (a) The survey XPS spectrum of MoS2 NSs, (b–d) the high-resolution XPS spectra of Mo, S and O, respectively. | |
SEM and TEM microscopic techniques were used to investigate the surface morphology of MoS2 NSs. Fig. 7a–c shows the SEM image of the synthesized MoS2 NSs. The image shows an aggregated and rough surface morphology of MoS2 NSs. Interesting sheet-like morphology observed under TEM analysis (Fig. 8). As it is shown in Fig. 8a and b, a few layers and aggregated MoS2 were synthesized via a facile hydrothermal method. The selected area electron diffraction (SAED) pattern (Fig. 8c) of the MoS2 sheet shows the smoothness of the concentric circle, which indicates the poor crystallinity of the sheet. Moreover, the (002), (100), and (001) planes keep stable, indicating the good stability of MoS2 along this plane.
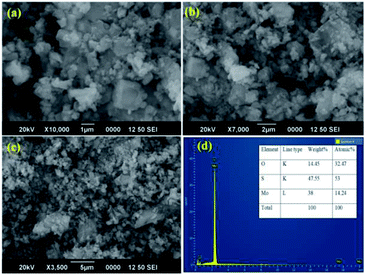 |
| Fig. 7 (a–c) The representative SEM images NSs at different magnifications, (d) EDS spectrum of MoS2. | |
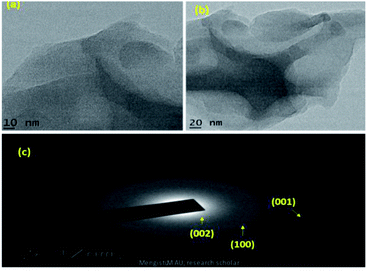 |
| Fig. 8 (a and b) The representative TEM images NSs at different magnifications, (c) SAED pattern of MoS2. | |
3.2. RhB and MO dye degradation via photocatalysis
The pH of the solution an important factor that affects the surface charge of the photocatalyst and the charge of the organic dye. As a result, the electrostatic interaction between the dye molecule and catalyst surface is highly affected by the pH of the solution. Methyl orange (MO) and rhodamine B (RhB) dyes were selected as representative anionic and cationic dyes respectively, for the photocatalytic investigation of MoS2 NSs. The acidic (pH = 4), neutral (pH = 7) and basic (pH = 9) medium were optimized to study the pH effect. Fig. 9 shows the effect of pH on the photocatalytic degradation of MO. As can be seen in Fig. 9, MoS2 NSs (30 mg) show a strong potential to degrade MO (20 mg L−1) at a given pH of the solution. Still, degradation of MO shows fast kinetics and almost complete degradations at pH = 4 due to the protonated surface at low pH enhance the electrostatic interaction with the anionic dye. Alkaline pH (pH = 9) also suitable to get a complete degradation of MO slightly at a longer time (Fig. 9c) than acidic. The increase in hydroxyl ions concentration in the solution facilitated the formation of further hydroxyl radical; this could be plausible for an enhanced reaction rate of degradation under alkaline conditions. The neutral pH (pH = 7) shows a relatively poor rate of degradation and efficiency (72%) (Fig. 9b). Hence, the acid or alkaline medium is suggested to get a better photocatalytic degradation efficiency of MO.
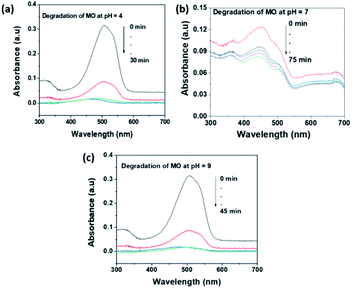 |
| Fig. 9 The effect of pH of solution for the degradation MO at (a) pH = 4, (b) pH = 7 and (c) pH = 9. | |
A similar investigation on the degradation of RhB performed at various pH of the solution. The potential of MoS2 NSs also observes for the degradation of a stable RhB dye. As shown in Fig. 10, complete degradation of 50 mL RhB (20 mg L−1) using 80 mg MoS2 NSs at acid and neutral solutions. Similar justification on the effect of pH for the degradation of RhB is applied to as MO. RhB was successfully removed with a high rate of degradation was observed at neutral pH (Fig. 10b). Concomitantly, the absorption maxima of RhB at λmax = 553 nm showed a shift to a lower wavelength region during the degradation process, which attributes to the formation of intermediate products in the meanwhile.
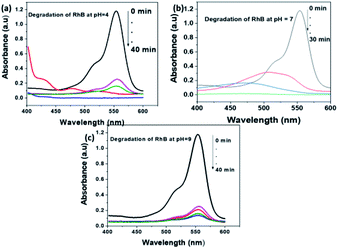 |
| Fig. 10 The effect of pH of solution for the degradation RhB at (a) pH = 4, (b) pH = 7 and (c) pH = 9. | |
The photocatalytic activity of MoS2 NSs was further investigated at various doses of the catalyst. At a fixed amount of dye concentration (50 mL, 20 mg L−1), at neutral pH and room temperature. Fig. 11 shows the effect catalyst dose for the removal of MO at the various dose of MoS2 (10 mg, 20 mg, and 30 mg). Results showed that fast catalytic activity of MoS2 NSs observed as a dosage increases from 20 mg to 30 mg. However, yet there is no significant difference between 20 mg and 30 mg dosages, and therefore 20 mg of could be considered as optimum for 50 mL MO (20 mg L−1). The same analogy was observed for RhB degradation. As shown in Fig. 12, the degradation of RhB (50 mL, 20 mg L−1) was investigated at various doses of MoS2 NSs (40 mg, 60 mg, and 80 mg). The degradation efficiency increases with an increasing dose of MoS2 NSs. The maximum value was obtained at 80 mg MoS2 NSs dose, considered as optimum.
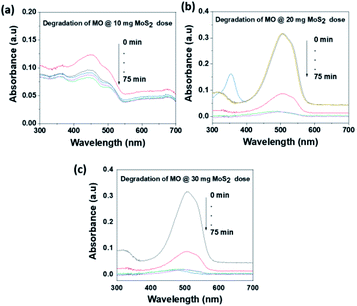 |
| Fig. 11 The effect of MoS2 NSs dose for the degradation MO. | |
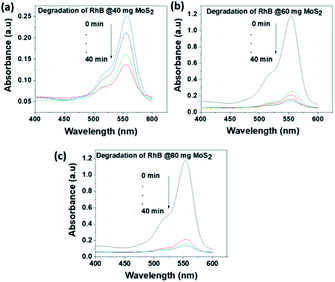 |
| Fig. 12 The effect of MoS2 NSs dose for the degradation RhB. | |
3.3. Photocatalytic mechanism
Fig. 13 shows the photocatalytic degradation mechanism of organic dye using catalysts. Firstly, the dye adsorbed on the surface of the MoS2 NSs in the dark because of the interaction between the shade and MoS2 NSs. Owing to the low bandgap of the MoS2 nanosheets, electrons and holes are generated. Electrons in the conduction band react with the available oxygen to form superoxide radicals (O2˙). Similarly, holes in the valence band react with water to form hydroxyl (OH˙) radicals. The formation of the OH˙ and O2˙ radicals has appeared. As a result, the generated OH radicals degrade the adsorbed dyes efficiently at room temperature by simple magnetic stirring. The production of radicals results in the mineralization of dyes into CO2, H2O, and other gaseous products.
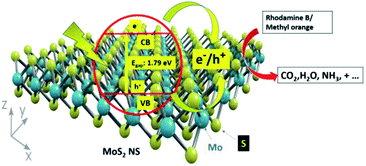 |
| Fig. 13 The plausible degradation mechanism of organic dye. | |
3.4. Anti-fungal activity of MoS2 nanosheets
The antimicrobial activity of MoS2 NSs against standard strains of Trichophyton mentagrophytes and Penicillium chrysogenum presented in Fig. 14. The higher inhibition zone is observed in Penicillium chrysogenum with higher concentrations of the synthesized material, i.e., 5 μg mL−1 of MoS2 nanosheet solutions. Mainly due to the increase in concentrations of MoS2 nanosheets, the diameter of the inhibition zone increased, i.e. 10 mm at 1 μg mL−1, 14 mm at 3 μg mL−1 and 16 mm at 5 μg mL−1. At higher concentrations, the diameter of the inhibition zone was more than the same concentrations of the standard drug inhibition (fluconazole (5 μg mL−1)). This result shows that a newly prepared nanosheet shows promising and requires further study for antifungal-related applications.
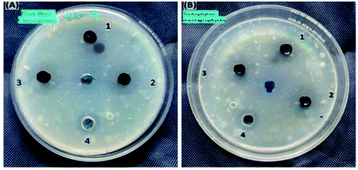 |
| Fig. 14 The antifungal activities of synthesized MoS2 nanosheet against (A) Penicillium chrysogenum and (B) Trichophyton mentagrophytes. | |
4. Conclusions
In conclusion, MoS2 NSs are synthesised successfully via a simple and green hydrothermal method using ammonium molybdenum hydrate and L-cysteine. In this process, to help the growth of MoS2 NSs, L-cysteine can be used as a sulfur source and a capping agent. The synthesized MoS2 NSS exhibited excellent photocatalytic degradation efficiency towards MO and RhB dyes under sunlight irradiation. It was achieved >99% degradation efficiency relatively fast rate reaction. The narrow bandgap (1.79 eV), and large surface area (6.46 m2 g−1) of the MoS2 NSs are responsible for the degradation of the dyes under sunlight. MoS2 NSs shows the toxic effect against fungi growth.
Conflicts of interest
There are no conflicts to declare.
Acknowledgements
The authors acknowledge Prof. K. Basavaiah for helpful discussions and giving guides. In addition, my friend Dr Neway Belachew contributes his role to accomplish this work. The authors also acknowledge the Ethiopian Government for financial assistance.
References
- R. Kant, Nat. Sci., 2012, 4, 22–26 CAS.
- A. S. M. Raja, A. Arputharaj, S. Saxena and P. G. Patil, Water in Textiles and Fashion, 2019, pp. 155–173 Search PubMed.
- N. Belachew, D. Rama Devi and K. Basavaiah, J. Mol. Liq., 2016, 224, 713–720 CrossRef CAS.
- V. Katheresan, J. Kansedo and S. Y. Lau, J. Environ. Chem. Eng., 2018, 6, 4676–4697 CrossRef CAS.
- N. Belachew, M. H. Kahsay, A. Tadesse and K. Basavaiah, J. Environ. Chem. Eng., 2020, 8, 104106 CrossRef CAS.
- D. Chen, Y. Cheng, N. Zhou, P. Chen, Y. Wang, K. Li, S. Huo, P. Cheng, P. Peng, R. Zhang, L. Wang, H. Liu, Y. Liu and R. Ruan, J. Cleaner Prod., 2020, 268, 121725 CrossRef CAS.
- M. H. Kahsay, A. Tadesse, D. RamaDevi, N. Belachew and K. Basavaiah, RSC Adv., 2019, 9, 36967–36981 RSC.
- F. Fresno, R. Portela, S. Suárez and J. M. Coronado, J. Mater. Chem. A, 2014, 2, 2863–2884 RSC.
- A. B. Djurišić, Y. H. Leung and A. M. C. Ng, Mater. Horiz., 2014, 1, 400 RSC.
- F. Kang, G. Sun, P. Boutinaud, H. Wu, F.-X. Ma, J. Lu, J. Gan, H. Bian, F. Gao and S. Xiao, Chem. Eng. J., 2021, 403, 126099 CrossRef CAS.
- A. Wang, F. Kang, Z. Wang, Q. Shao, Z. Li, G. Zhu, J. Lu and Y. Y. Li, Adv. Sustainable Syst., 2019, 3, 1800132 CrossRef.
- A. Wang, Y.-L. Hou, F. Kang, F. Lyu, Y. Xiong, W.-C. Chen, C.-S. Lee, Z. Xu, A. L. Rogach, J. Lu and Y. Y. Li, J. Mater. Chem. C, 2019, 7, 2207–2211 RSC.
- Z. Yu, L. Tetard, L. Zhai and J. Thomas, Energy Environ. Sci., 2015, 8, 702–730 RSC.
- B. Luo, G. Liu and L. Wang, Nanoscale, 2016, 8, 6904–6920 RSC.
- R. Hu, G. Liao, Z. Huang, H. Qiao, H. Liu, Y. Shu, B. Wang and X. Qi, J. Hazard. Mater., 2021, 405, 124179 CrossRef CAS.
- J. Kaur, M. Singh, C. Dell'Aversana, R. Benedetti, P. Giardina, M. Rossi, M. Valadan, A. Vergara, A. Cutarelli, A. M. I. Montone, L. Altucci, F. Corrado, A. Nebbioso and C. Altucci, Sci. Rep., 2018, 8, 16386 CrossRef.
- M. Singh, C. Zannella, V. Folliero, R. Di Girolamo, F. Bajardi, A. Chianese, L. Altucci, A. Damasco, M. R. Del Sorbo, C. Imperatore, M. Rossi, M. Valadan, M. Varra, A. Vergara, G. Franci, M. Galdiero and C. Altucci, Front. Bioeng. Biotechnol., 2020, 8, 1056 Search PubMed.
- J. Kaur, A. M. Gravagnuolo, P. Maddalena, C. Altucci, P. Giardina and F. Gesuele, RSC Adv., 2017, 7, 22400–22408 RSC.
- J. Kaur, A. Vergara, M. Rossi, A. M. Gravagnuolo, M. Valadan, F. Corrado, M. Conte, F. Gesuele, P. Giardina and C. Altucci, RSC Adv., 2017, 7, 50166–50175 RSC.
- B. Radisavljevic, A. Radenovic, J. Brivio, V. Giacometti and A. Kis, Nat. Nanotechnol., 2011, 6, 147–150 CrossRef CAS.
- X. Li and H. Zhu, J. Materiomics, 2015, 1, 33–44 CrossRef.
- S. Wi, H. Kim, M. Chen, H. Nam, L. J. Guo, E. Meyhofer and X. Liang, ACS Nano, 2014, 8, 5270–5281 CrossRef CAS.
- K. F. Mak, C. Lee, J. Hone, J. Shan and T. F. Heinz, Phys. Rev. Lett., 2010, 105, 136805 CrossRef PubMed.
- M. H. Mokari-Manshadi, M. Mahani, Z. Hassani, D. Afzali and E. Esmaeilzadeh, J. Nanosci. Nanotechnol., 2017, 17, 8864–8868 CrossRef CAS.
- M. Sabarinathan, S. Harish, J. Archana, M. Navaneethan, H. Ikeda and Y. Hayakawa, RSC Adv., 2017, 7, 24754–24763 RSC.
- Y.-H. Lee, X.-Q. Zhang, W. Zhang, M.-T. Chang, C.-T. Lin, K.-D. Chang, Y.-C. Yu, J. T.-W. Wang, C.-S. Chang, L.-J. Li and T.-W. Lin, Adv. Mater., 2012, 24, 2320–2325 CrossRef CAS.
- K. Sheybani and S. Javadpour, Int. J. Refract. Met. Hard Mater., 2020, 92, 105277 CrossRef CAS.
- N. Chaudhary, M. Khanuja, Abid and S. S. Islam, Sens. Actuators, A, 2018, 277, 190–198 CrossRef CAS.
- T. Oztas, H. S. Sen, E. Durgun and B. Ortaç, J. Phys. Chem. C, 2014, 118, 30120–30126 CrossRef CAS.
- E. Varrla, C. Backes, K. R. Paton, A. Harvey, Z. Gholamvand, J. McCauley and J. N. Coleman, Chem. Mater., 2015, 27, 1129–1139 CrossRef CAS.
- A. M. Gravagnuolo, E. Morales-Narváez, S. Longobardi, E. T. da Silva, P. Giardina and A. Merkoçi, Adv. Funct. Mater., 2015, 25, 2771–2779 CrossRef CAS.
- I. Uzcanga, I. Bezverkhyy, P. Afanasiev, C. Scott and M. Vrinat, Chem. Mater., 2005, 17, 3575–3577 CrossRef CAS.
- M. Rakibuddin, M. A. Shinde and H. Kim, Electrochim. Acta, 2020, 349, 136403 CrossRef CAS.
- H. K. Sadhanala, S. Senapati, K. V. Harika, K. K. Nanda and A. Gedanken, New J. Chem., 2018, 42, 14318–14324 RSC.
- P. Singh, Y.-J. Kim, D. Zhang and D.-C. Yang, Trends Biotechnol., 2016, 34, 588–599 CrossRef CAS.
- Y. Guo and J. Li, Mater. Sci. Eng. C, 2020, 109, 110511 CrossRef CAS.
- N. Belachew, D. S. Meshesha and K. Basavaiah, RSC Adv., 2019, 9, 39264–39271 RSC.
- S.-K. Park, S.-H. Yu, S. Woo, B. Quan, D.-C. Lee, M. K. Kim, Y.-E. Sung and Y. Piao, Dalton Trans., 2013, 42, 2399–2405 RSC.
- C. P. Veeramalai, F. Li, Y. Liu, Z. Xu, T. Guo and T. W. Kim, Appl. Surf. Sci., 2016, 389, 1017–1022 CrossRef CAS.
- J. Georgin, G. L. Dotto, M. A. Mazutti and E. L. Foletto, J. Environ. Chem. Eng., 2016, 4, 266–275 CrossRef CAS.
- P. Sinha, A. Datar, C. Jeong, X. Deng, Y. G. Chung and L.-C. Lin, J. Phys. Chem. C, 2019, 123, 20195–20209 CrossRef CAS.
- H. F. McMurdie, M. C. Morris, E. H. Evans, B. Paretzkin, W. Wong-NG and C. R. Hubbard, Powder Diffr., 1986, 1, 40–43 CrossRef CAS.
- T. S. Sahu and S. Mitra, Sci. Rep., 2015, 5, 12571 CrossRef CAS.
- N. Belachew and H. Hinsene, Appl. Water Sci., 2020, 10, 38 CrossRef CAS.
- G. A. M. Ali, M. R. Thalji, W. C. Soh, H. Algarni and K. F. Chong, J. Solid State Electrochem., 2020, 24, 25–34 CrossRef CAS.
- G. Qingquan, M. Xinfu, X. Yu, T. Wei and Z. Hui, Colloids Surf., A, 2017, 530, 33–37 CrossRef.
- K. C. Lalithambika, K. Shanmugapriya and S. Sriram, Appl. Phys. A: Solids Surf., 2019, 125, 817 CrossRef CAS.
- W. Feng, L. Chen, M. Qin, X. Zhou, Q. Zhang, Y. Miao, K. Qiu, Y. Zhang and C. He, Sci. Rep., 2015, 5, 17422 CrossRef CAS.
- J. Kibsgaard, Z. Chen, B. N. Reinecke and T. F. Jaramillo, Nat. Mater., 2012, 11, 963–969 CrossRef CAS PubMed.
- S. Cui, Z. Wen, X. Huang, J. Chang and J. Chen, Small, 2015, 11, 2305–2313 CrossRef CAS.
- C. Zhang, Z. Wang, S. Bhoyate, T. Morey, B. Neria, V. Vasiraju, G. Gupta, S. Palchoudhury, P. Kahol, S. Mishra, F. Perez and R. Gupta, C, 2017, 3, 33 Search PubMed.
- L. Ge, C. Han, X. Xiao and L. Guo, Int. J. Hydrogen Energy, 2013, 38, 6960–6969 CrossRef CAS.
- Y. Huang, Y. Chen, C. Hu, B. Zhang, T. Shen, X. Chen and M. Q. Zhang, J. Mater. Chem., 2012, 22, 10999 RSC.
Footnote |
† Electronic supplementary information (ESI) available: Details of antifungal procedure, BET plot and photographic images of MoS2 powder. See DOI: 10.1039/d1ra03815j |
|
This journal is © The Royal Society of Chemistry 2021 |