DOI:
10.1039/D1RA01648B
(Paper)
RSC Adv., 2021,
11, 12442-12448
Prominent roles of Ni(OH)2 deposited on ZnIn2S4 microspheres in efficient charge separation and photocatalytic H2 evolution†
Received
2nd March 2021
, Accepted 23rd March 2021
First published on 29th March 2021
Abstract
In this work, Ni(OH)2-deposited ZnIn2S4 microspheres (Ni(OH)2/ZnIn2S4) were fabricated using a hydrothermal process, followed by a facile in situ precipitation method. It was demonstrated that the deposition of Ni(OH)2 on ZnIn2S4 effectively promotes the separation of charges photogenerated over ZnIn2S4, and significantly enhances photocatalytic H2 evolution. The optimum rate of the photocatalytic H2 evolution over the 6% Ni(OH)2/ZnIn2S4 composite reaches 4.43 mmol g−1 h−1, which is 21.1 times higher than that of the pure ZnIn2S4. Based on various characterization results and Au photo-deposition on the composite, it was proposed that the capture of the photogenerated holes by the deposited Ni(OH)2 would be responsible for the efficient charge separation, which allows more photogenerated electrons to be left on the ZnIn2S4 for the reduction of H+ to H2 with a higher rate.
1. Introduction
Photocatalytic hydrogen production by water splitting has become one of the sustainable and environmentally friendly strategies for solving the consumption of fossil fuels and the resulting environmental pollution.1–3 For the full utilization of solar energy, a considerable number of narrow band gap semiconductors have been widely investigated as visible light photocatalysts for hydrogen production. Of these semiconductors, CdS, g-C3N4, and ZnIn2S4 have received intensive attention since their band gaps match well with the spectrum of sunlight, and their conduction band edges are more negative than the redox potential of H+/H2.4–6 Unfortunately, the direct application of these semiconductors themselves for photocatalytic H2 production is almost infeasible mainly due to their rapid charge recombination.7–9 For an efficient photocatalytic H2 production reaction, it is indispensable to load a noble metal such as Pt, Pd, Ag, or Au as a cocatalyst on the host semiconductors.10–13 The noble metals as cocatalysts can not only facilitate the separation of electron–hole pairs over host semiconductor effectively, but also function as active sites for H2 production. Nevertheless, the high cost and scarcity of these noble metals seriously hinder their practical application. Therefore, it is desirable to exploit noble metal-free, abundant, and durable cocatalysts for photocatalytic H2 production.
Recently, numerous works reported that the modification of various narrow band gap semiconductors with molybdenum disulfide (MoS2), an earth-abundant compound, significantly enhances photocatalytic H2 production from water splitting and quantum efficiency.14–16 Both experimental and computational results also well revealed the photogenerated charge transfer, capture, and active sites for H2 production in the systems with MoS2 as a cocatalyst.17,18 Afterward, other transition metal (e.g. Ni, Co, Cu, Fe) disulfides and phosphides have been investigated and utilized as cocatalysts for assisting photocatalytic H2 production.19–22 In addition, hydroxide can also act as a cocatalyst for photocatalytic H2 production. In 2011, a typical pioneer work reported that Ni(OH)2-decorated TiO2 could give a H2 evolution rate of 3056 μmol g−1 h−1 and a quantum efficiency of 12.4% under UV irradiation, which is 233 times than that of pure TiO2.23 Following this work, hydroxides such as Ni(OH)2 and Co(OH)2 have been widely applied as cocatalysts for photocatalytic H2 production by coupling with proper narrow band gap semiconductors including CdS, g-C3N4, and ZnIn2S4.24–29 These works have well demonstrated that the hydroxides are capable of being alternatives for noble metal cocatalysts, and deserve more and further attention in photocatalytic H2 production. Especially, the deepening of the knowledge of photogenerated charge transfer, capture, and active sites for H2 production would be helpful for the design and optimization of hydroxide-modified photocatalysts. In the present work, we fabricated the Ni(OH)2-deposited ZnIn2S4 composites by a facile in situ precipitation method. Upon visible light irradiation, the photocatalytic activity of ZnIn2S4 for H2 evolution has been shown to be significantly enhanced after the deposition of Ni(OH)2. Furthermore, the roles of the deposited Ni(OH)2 in the enhanced photocatalytic H2 evolution of the composite were revealed according to various characterization results and photodeposition of Au nanoparticles on the composite. This work, we believe, offers a new insight into the photogenerated charge capture, separation, and active sites for H2 evolution in the composite.
2. Experimental section
2.1. Sample preparation
ZnIn2S4 samples were synthesized by a literature method with a slight modification.30 Briefly, 0.2862 g of ZnCl2 and 1.2484 g of InCl3·4H2O were dissolved in 40 ml of distilled water under vigorous stirring, followed by the addition of polyethylene glycol (PEG-6000, 0.8836 g) and thioacetamide (TAA, 1.2096 g). The obtained mixture, a homogeneous solution, was transferred into a 100 ml Teflon-lined stainless steel autoclave and heated at 160 °C for 16 h in an oven. After cooled down to room temperature naturally, the yellow products ZnIn2S4 were collected by centrifugation and washed with water and ethanol for three times before dried at 60 °C in a vacuum oven for overnight.
The deposition of Ni(OH)2 on the surface of ZnIn2S4 was conducted by a facile in situ precipitation method. In a typical procedure, 0.2 g of the as-prepared ZnIn2S4 was dispersed in 50 ml of NaOH aqueous solution (0.25 M). Under agitation, into the dispersion was the desired amount of Ni(NO3)2·6H2O aqueous solution (0.05 M) added dropwise. The resulting mixture was stirred for 5 h at room temperature. Subsequently, the final product was collected by centrifugation, and thoroughly washed with deionized water, and finally dried in a vacuum oven at 60 °C for 12 h. According to the nominal molar ratios of Ni(NO3)2 to (Ni(NO3)2 + ZnIn2S4) in the above procedure, the obtained samples were denoted as 0%, 2%, 4%, 6%, and 8% Ni(OH)2/ZnIn2S4, respectively.
2.2. Characterization
The polycrystalline X-ray diffraction (XRD) patterns of the prepared samples were collected on an X-ray diffractometer (Shimadzu, XRD-7000) with Cu Kα radiation under 40 kV and 150 mA. The morphological and microstructural features of the samples were captured by using a field-emission scanning microscope (FESEM) (JEOL 6701F) and a high-resolution transmission electron microscope (HRTEM) (JEOL-2010), respectively. X-ray photoelectron spectroscopy (XPS) analysis was performed in a Thermo ESCALAB 250 electron spectrometer with a monochromatic Al Kα source. All the binding energies were referenced to the C 1s peak of the surface adventitious carbon at 284.6 eV. The optical absorption properties of the samples were analyzed with UV-visible diffuse reflectance spectroscopy (Hitachi UH-4150) with BaSO4 as a reflectance standard.
Transient photocurrent response and electrochemical impedance spectroscopy (EIS) measurements were carried out in a three-electrode system on an electrochemical work station (Chenhua, Shanghai). A Pt wire and a Ag/AgCl in a saturated KCl solution were employed as the counter electrode and the reference electrode, respectively. The working electrode was prepared by coating sample slurry onto ITO glass substrate with a fixed area of 1 × 1 cm2 followed by drying with an infrared lamp irradiation. A 1.0 M Na2SO4 aqueous solution was used as the electrolyte for both photocurrent response and electrochemical impedance spectroscopy (EIS) measurements. The visible light irradiation source is a 3 W monochromatic light (λ = 420 nm).
2.3. Photocatalytic experiments
The experiments of the photocatalytic H2 production over these prepared samples irradiated by visible light were carried out in a gas-closed Pyrex flask at ambient temperature. Typically, 20 mg of the prepared sample powder was suspended in 100 ml of 10 vol% lactic acid aqueous solution under magnetic stirring. The suspension was thoroughly degassed with N2 for 30 min before irradiated by a 300 W Xe arc lamp (LabSolar II, Perfect Light Co., Ltd) equipped with a cut-off filter (λ > 420 nm). The amount of hydrogen evolution during the reaction was periodically analyzed by a gas chromatography (GC-2014C, Shimadzu, Japan) with a TCD detector and a molecular sieve 5A column with N2 as the carrier gas.
To study the reduction sites for H2 evolution in the Ni(OH)2/ZnIn2S4 composite, the photocatalytic deposition of Au on 6% Ni(OH)2/ZnIn2S4 was performed at ambient temperature. Briefly, 50 mg of 6% Ni(OH)2/ZnIn2S4 composite was dispersed in 50 ml of 10 vol% benzyl alcohol aqueous solution under stirring, followed by the addition of 52 μl of HAuCl4·4H2O aqueous solution (1 g/50 ml). Prior to the irradiation by a 300 W Xe arc lamp (LabSolar II, Perfect Light Co., Ltd) equipped with a cut-off filter (λ > 420 nm), the dispersion was thoroughly degassed with N2 for 30 min. After the irradiation for 2 h, the composite was collected by centrifugation, washed with ethanol and water several times, and finally dried at 60 °C in a vacuum oven overnight for further TEM observation.
3. Results and discussion
3.1. Phase structure and morphology
The polycrystalline X-ray diffraction patterns of the prepared pure ZnIn2S4 and Ni(OH)2/ZnIn2S4 composites are shown in Fig. 1. All samples exhibit a single characteristic hexagonal phase of ZnIn2S4 (JPCDS no. 65-2023), and no changes in the intensities and widths of the XDR peaks occur with the Ni(OH)2 loading. These indicate that the deposition of Ni(OH)2 on ZnIn2S4 doesn't alter the phase structure and crystallinity of ZnIn2S4 substrate. Notably, the relative intensity of (112) diffraction peak at approximately 47.5 degree for all samples is obviously enhanced as compared to the corresponding one of the reference (JPCDS no. 65-2023). This is perhaps due to the preferential orientation growth of the crystalline plane (112) in our synthesis process. Moreover, no characteristic XRD peaks associated with Ni(OH)2 are observed in all Ni(OH)2/ZnIn2S4 composites, which is probably due to the low content and high dispersion of Ni(OH)2 on ZnIn2S4. The presence of Ni(OH)2 would be confirmed by the HRTEM observation and XPS analysis below.
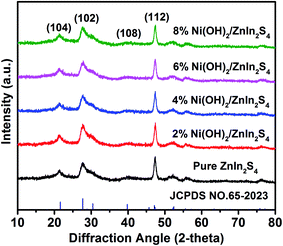 |
| Fig. 1 Polycrystalline X-ray diffraction patterns of pure ZnIn2S4 and Ni(OH)2/ZnIn2S4 composites. | |
Fig. 2a and b display the FESEM images of the pure ZnIn2S4 and 6% Ni(OH)2/ZnIn2S4 composite, respectively. As can be seen in Fig. 2a, the pure ZnIn2S4 shows uniform microspheres with an average diameter of ca. 6–8 μm. These microspheres (Fig. 2c) consist of a great number of curling nanosheets, which could be helpful for the deposition of Ni(OH)2 and photocatalytic H2 evolution by offering a large surface area. A careful observation (Fig. 2b and d) indicates that the introduction of Ni(OH)2 doesn't alert the morphology and size of ZnIn2S4 microsphere, except that the curling nanosheets become a little rough. The HRTEM images (Fig. 2e and f) of 6% Ni(OH)2/ZnIn2S4 composite reveals that the crystalline Ni(OH)2 are well deposited onto the surface of well-crystalline ZnIn2S4 to form heterostructures with the intimate interfaces.7,8 The lattice fringes with the spacing of ca. 0.32 nm and 0.27 nm well correspond to the crystallographic plane (102) of hexagonal ZnIn2S4 and the crystallographic plane (100) of hexagonal Ni(OH)2, respectively.25,31 The high-angle annular dark field-scanning transmission electron microscopy (HAADF-STEM) image (Fig. S1a†) and energy dispersive spectroscopy (EDS) elemental mapping (Fig. S1b–f†) analysis of 6% Ni(OH)2/ZnIn2S4 composite was performed to characterize the elemental constitute and distribution. The elemental mapping confirms the uniform distribution of Zn, In, S, Ni, and O elements over the selected detection area of the composite. The weaker signals of Ni and O are well consistent with the lower molar percentage of Ni(OH)2 in the composite.
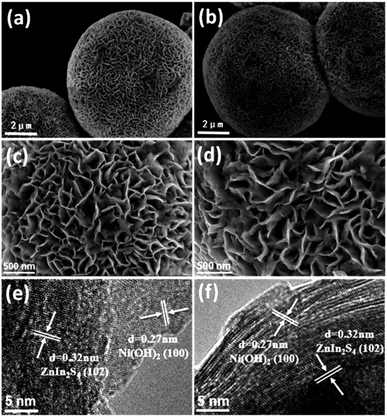 |
| Fig. 2 FESEM images of (a and c) pure ZnIn2S4 and (b and d) 6% Ni(OH)2/ZnIn2S4 with different scales; (e and f) HRTEM images of 6% Ni(OH)2/ZnIn2S4. | |
3.2. XPS analysis and optical property
The compositions and chemical states of the elements in the pure ZnIn2S4 and 6% Ni(OH)2/ZnIn2S4 were further analyzed by XPS. The XPS survey spectra, as displayed in Fig. S2,† clearly indicate the presence of Zn, In, S, O, and C in both samples, and Ni in 6% Ni(OH)2/ZnIn2S4 only. Fig. 3a–c show the high resolution spectra of Zn 2p, In 3d, and S 2p regions of the pure ZnIn2S4 and 6% Ni(OH)2/ZnIn2S4, respectively. In the Zn 2p core-level spectrum of pure ZnIn2S4 (Fig. 3a), two peaks at the binding energies of 1021.9 and 1045.0 eV, with a separation of 23.1 eV, are assigned to Zn 2p3/2 and Zn 2p1/2 of Zn2+, respectively.32 The two symmetric peaks of In 3d located at the binding energies of 444.8 and 452.4 eV in the In 3d core-level spectrum of pure ZnIn2S4 with a peak difference of 7.6 eV (Fig. 3b) are ascribed to In 3d5/2 and In 3d3/2 of In3+, respectively.33,34 The S 2p spectrum of pure ZnIn2S4 shown in Fig. 3c appears to be a broad peak, which can be deconvoluted two peaks with the binding energies of 161.7 and 162.9 eV, corresponding to S 2p3/2 and S 2p1/2, respectively.31,35 Collectively, the binding energies of Zn, In, and S match well the feature of ZnIn2S4. The high resolution XPS spectrum of Ni 2p, as shown in Fig. 3d, clearly demonstrates two main peaks at 855.7 and 873.1 eV corresponding to typical Ni 2p3/2 and Ni 2p1/2, respectively.25 Two shake-up satellite peaks for Ni 2p3/2 and Ni 2p1/2 are observed at 861.5 and 880.8 eV. These binding energies of Ni 2p indicate the characteristic of Ni2+ in Ni(OH)2 based on the literature reports.36 It is worth noting that the deposition of Ni(OH)2 on ZnIn2S4 cause a slight shift in the binding energies of Zn 2p, In 3d, and S 2p peaks. The observed shifts suggest a strong chemical bond between Ni(OH)2 and ZnIn2S4, which would be favorable for the charge transfer between them. The XPS measurements evidence the formation of Ni(OH)2-deposited ZnIn2S4 heterostructures with the well-contacted interfaces, further confirming the above HRTEM observation.
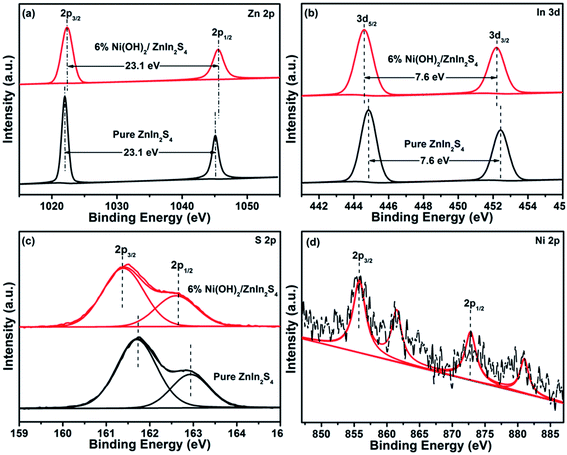 |
| Fig. 3 High resolution XPS spectra of Zn 2p (a), In 3d (b), and S 2p (c) in pure ZnIn2S4 and 6% Ni(OH)2/ZnIn2S4, and Ni 2p (d) in 6% Ni(OH)2/ZnIn2S4. | |
The optical absorption properties of the pure ZnIn2S4 and Ni(OH)2/ZnIn2S4 composites with the different amount of deposited Ni(OH)2 were investigated by UV-visible diffuse reflectance spectroscopy. As displayed in Fig. 4, the pure ZnIn2S4 exhibits an intense absorption with an edge at ca. 525 nm, which can be attributed to the intrinsic band gap absorption of hexagonal ZnIn2S4 (∼2.36 eV). This coincides with the reported value of ZnIn2S4.37 In contrast with the pure ZnIn2S4, the deposition of Ni(OH)2 enhances the light absorption in the visible region. The absorption intensity of the composites in visible region gradually increases with the increase of the molar ratio of Ni(OH)2 in the composites. Furthermore, almost no obvious absorption edge differences among the absorption spectra of the pure ZnIn2S4 and Ni(OH)2/ZnIn2S4 composites suggest the deposition of Ni(OH)2 on the surface of ZnIn2S4.
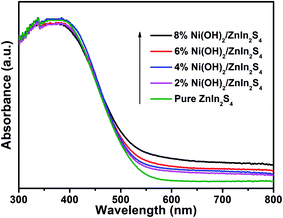 |
| Fig. 4 UV-vis diffuse reflectance spectra of pure ZnIn2S4 and Ni(OH)2/ZnIn2S4 composites. | |
3.3. Photocatalytic H2 evolution
The photocatalytic H2 evolution over the pure ZnIn2S4, Ni(OH)2, and Ni(OH)2/ZnIn2S4 composites was evaluated under visible light (λ > 420 nm) irradiation in the presence of lactic acid as a sacrificial reagent. Control experiments show that no appreciable H2 evolution is detected in the absence of either visible light irradiation or catalyst. As depicted in Fig. 5a, H2 evolution rate over the pure ZnIn2S4 is negligible due to the rapid recombination of electron–hole pairs over the irradiated pure ZnIn2S4, and also no appreciable H2 activity is observed on pure Ni(OH)2. However, a proper combination of both generates excellent photocatalytic H2 evolution. As the deposition of 2% Ni(OH)2 on ZnIn2S4, the rate of H2 evolution is significantly enhanced to 2.20 mmol g−1 h−1. The rate of H2 evolution further increases with the amount of deposited Ni(OH)2, and reaches an optimum of 4.43 mmol g−1 h−1 over 6% Ni(OH)2/ZnIn2S4, which is 21.2 times higher than that of pure ZnIn2S4. After the optimum, the reduction in H2 evolution rate over 8% Ni(OH)2/ZnIn2S4 would be attributed to the shielding effect of excessive Ni(OH)2 which impedes the light absorption of the substrate ZnIn2S4. The above result well demonstrates the deposition of Ni(OH)2 with an appropriate amount is an effective method for boosting the photocatalytic H2 evolution over ZnIn2S4, which is consistent with the related literatures.25
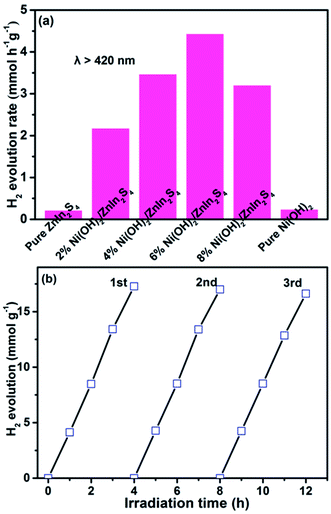 |
| Fig. 5 (a) Photocatalytic H2 evolution rates over pure ZnIn2S4, Ni(OH)2, and Ni(OH)2/ZnIn2S4 composites. (b) Cycling photocatalytic H2 evolution over 6% Ni(OH)2/ZnIn2S4. | |
The stability of photocatalytic H2 evolution over 6% Ni(OH)2/ZnIn2S4 was examined by conducting the photocatalytic recycle experiments. As shown in Fig. 5b, after three recycle (12 h), no apparent decrease in H2 evolution over the composite can be observed, indicating the good stability of the composite for photocatalytic H2 evolution.
3.4. Mechanism of enhanced photocatalytic H2 evolution over Ni(OH)2/ZnIn2S4
To understand the mechanism of the enhanced photocatalytic H2 evolution over Ni(OH)2/ZnIn2S4, the role of the deposited Ni(OH)2 on the surface of ZnIn2S4 in the transfer and separation of charges photogenerated over ZnIn2S4 was investigated by measuring the transient photocurrent response. Fig. 6a shows the transient photocurrent responses of the pure ZnIn2S4 and 6% Ni(OH)2/ZnIn2S4 under visible light irradiation (λ = 420 nm) with 50 s light on/off cycles. It can be clearly observed that 6% Ni(OH)2/ZnIn2S4 exhibits much higher photocurrent density than pure ZnIn2S4. As well known, the photocurrent density is directly proportional to the transfer and separation of photogenerated charge.38,39 The higher photocurrent density over 6% Ni(OH)2/ZnIn2S4 composite than pure Ni(OH)2 reveals that the deposition of Ni(OH)2 on ZnIn2S4 benefits the charge transfer across the intimate interfaces between Ni(OH)2 and ZnIn2S4, and achieves the efficient separation of charges photogenerated over ZnIn2S4. Moreover, the interfacial charge transfer and separation of two samples was also characterized by electrochemical impedance spectroscopy (EIS) measurements, as shown in Fig. 6b. Generally, the small curvature radius of the Nyquist curves represents a low charge transfer resistance, suggesting the efficient separation and transfer of photogenerated charges. The smaller curvature radius of the EIS plot of 6% Ni(OH)2/ZnIn2S4 compared to that of pure ZnIn2S4 further confirms that the efficient separation and transfer of photogenerated charges are realized after the introduction of Ni(OH)2.40 The transient photocurrent response and electrochemical impedance spectroscopy (EIS) results of two samples parallel their performances (Fig. 5a) for photocatalytic H2 evolution.
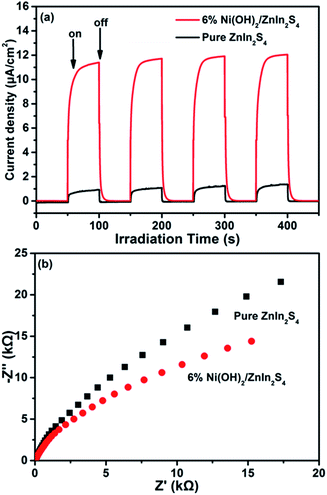 |
| Fig. 6 Transient photocurrent responses (a) and electrochemical impedance spectroscopy Nyquist plots (b) of pure ZnIn2S4 and 6% Ni(OH)2/ZnIn2S4. | |
Based on the above results of the transient photocurrent response and electrochemical impedance spectroscopy measurements, without a doubt, it is the deposition of Ni(OH)2 on the surface of ZnIn2S4 that the efficient separation of electron–hole pairs photogenerated over ZnIn2S4 has been achieved through the charge transfer across the intimate interfaces between Ni(OH)2 and ZnIn2S4. Subsequently, we need to elucidate how the deposited Ni(OH)2 effectively promotes the charge transfer to realize the separation of electron–hole pairs. According to the literature reports, the conduction band (CB) and valence band (VB) edges of ZnIn2S4 are −1.35 V and +1.4 V vs. NHE, respectively,31 while the redox potentials of Ni(OH)2/Ni and Ni(OH)3/Ni(OH)2 are −0.72 V and +0.48 vs. NHE, respectively.41 From the view of thermodynamics, thus, there are two possible paths that the deposited Ni(OH)2 promotes the separation of electron–hole pairs photogenerated over ZnIn2S4 composite. In path I, the photo-induced electrons on CB of ZnIn2S4 transfer to the deposited Ni(OH)2 and reduce partial Ni2+ to form Ni0, where the bound proton H+ accepts the electrons from Ni0 to be reduced to H2. In path II, the photo-induced holes on VB of ZnIn2S4 are scavenged by the deposited Ni(OH)2 to form Ni(OH)3, allowing the electrons left on CB of ZnIn2S4 to reduce H+ to H2. Obviously, the reduction sites of H+ to H2 in two paths are on the deposited Ni(OH)2 and ZnIn2S4, respectively. Either path I or path II is an actual running one. This problem, we believe, can be clarified by the identification of the reduction sites in Ni(OH)2/ZnIn2S4 composite. To this end, the photocatalytic deposition of Au on 6% Ni(OH)2/ZnIn2S4 composite was conducted in the presence of HAuCl4·4H2O as Au precursor. As shown in Fig. 7a, after the photo-deposition of Au on the composite, several dark gray nanoparticles clearly appear on the surface of the crystalline ZnIn2S4 only.7,8 A HRTEM (Fig. 7b) analysis further indicates that the lattice fringe of 0.23 nm in a dark gray nanoparticle matches the crystallographic plane (111) of metallic cubic-phase Au.42 The results well demonstrate that the reduction of AuCl4− to Au0 by the photogenerated electrons in the composite occurs on the surface of ZnIn2S4 rather than Ni(OH)2, suggesting that the photogenerated electrons are left on the ZnIn2S4, not transferred to the Ni(OH)2. In the same way, the sites for the reduction of H+ to H2 in Ni(OH)2/ZnIn2S4 composite should be also on the surface of ZnIn2S4, which excludes the possibility of path I.
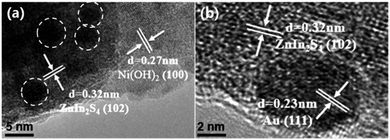 |
| Fig. 7 TEM (a) and HRTEM (b) images of Au-photodeposited 6% Ni(OH)2/ZnIn2S4. | |
According to the above discussion and various experiments, therefore, a understanding of the enhanced photocatalytic H2 evolution over Ni(OH)2/ZnIn2S4 composite can be schematically illustrated in Scheme 1. The H2 evolution rate is very low over the pure ZnIn2S4 due to the rapid recombination of CB electrons and VB holes. After the deposition of Ni(OH)2 on ZnIn2S4, the VB holes can be scavenged by deposited Ni(OH)2 to form Ni(OH)3 across the intimate interfaces between ZnIn2S4 and Ni(OH)2 since the valence band edge of ZnIn2S4 (+1.4 V vs. NHE) is more positive than the redox potential of Ni(OH)3/Ni(OH)2 (+0.48 vs. NHE).41 This leads to an efficient separation of charges photogenerated over ZnIn2S4 and allows more photogenerated electrons left on the surface of ZnIn2S4 to reduce the bound protons H+ to H2, enhancing photocatalytic activity. Meanwhile, the formed Ni(OH)3 can easily become back to Ni(OH)2 through the oxidation of the added lactic acid as a sacrificial reagent, continuously driving the charge separation.
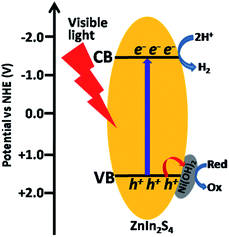 |
| Scheme 1 Schematic illustration of photocatalytic H2 evolution over Ni(OH)2/ZnIn2S4 composite. | |
4. Conclusion
In summary, the crystalline Ni(OH)2 have been successfully deposited on the surface of ZnIn2S4 through a facile in situ precipitation method. The deposition of Ni(OH)2 on ZnIn2S4 was demonstrated to significantly enhance the photocatalytic H2 evolution over ZnIn2S4. Various characterization results indicate that the deposition of Ni(OH)2 on ZnIn2S4 effectively promotes the separation of charges photogenerated over ZnIn2S4. Furthermore, the experiment of the photodeposition of Au nanoparticles reveals that the scavenging of the photogenerated holes by the deposited Ni(OH)2 effectively inhibits the recombination rate of the electron–hole pairs, boosting a high photocatalytic H2 evolution rate over ZnIn2S4.
Author contributions
Zhuang Guo synthesized samples, conducted various characterizations, and drafted the paper. Huixia Hou, Jingyi Zhang and Pinglong Cai performed the electrochemical measurements and participated in the photocatalytic experiments. Jun Lin conceived the idea, revised the paper, and approved the final version.
Conflicts of interest
There are no conflicts to declare.
Acknowledgements
This work was financially supported by the National Natural Science Foundation of China (Grant No. 21673287).
Notes and references
- A. Kudo and Y. Miseki, Chem. Soc. Rev., 2009, 38, 253–278 RSC.
- U. Maitra, U. Gupta, M. De, R. Datta, A. Govindaraj and C. Rao, Angew. Chem., Int. Ed., 2013, 52, 13057–13061 CrossRef CAS PubMed.
- Y. X. Fang, X. C. Li and X. C. Wang, ACS Catal., 2018, 8, 8774–8780 CrossRef CAS.
- X. Yan, Z. L. Jin, Y. P. Zhang, H. Liu and X. L. Ma, Phys. Chem. Chem. Phys., 2019, 21, 4501–4512 RSC.
- H. Wei, W. A. McMaster, J. Z. Y. Tan, L. Cao, D. Chen and R. A. Caruso, J. Phys. Chem. C, 2017, 121, 22114–22122 CrossRef CAS.
- Z. Guan, Z. Xu, Q. Li, P. Wang, G. Li and J. Yang, Appl. Catal., B, 2018, 227, 512–518 CrossRef CAS.
- A. Luna-Flores, M. A. Morales, R. Agustín-Serrano, R. Portillo, J. A. Luna-López, G. F. Pérez-Sánchez, A. D. Hernández-de la Luz and N. Tepale, Catalysts, 2019, 9, 817 CrossRef CAS.
- M. A. Moralesa, I. Fernández-Cervantesb, R. Agustín-Serranoe, S. Ruíz-Salgadoa, M. P. Sampedroa, J. L. Varela-Caselisc, R. Portillod and E. Rubio, Results Phys., 2019, 12, 1344–1356 CrossRef.
- S. Kim, Y. Wang, M. S. Zhu, M. Fujitsuka and T. Majima, Chem.–Eur. J., 2018, 24, 14928–14932 CrossRef CAS PubMed.
- S. Z. Liu, Z. Guo, X. H. Qian, J. Y. Zhang, J. X. Liu and J. Lin, Sustainable Energy Fuels, 2019, 3, 1048–1054 RSC.
- F. N. Sayed, O. D. Jayakumar, R. Sasikala, R. M. Kadam, S. R. Bharadwaj, L. Kienle, U. SchÜrmann, S. Kaps, R. Adelung, J. P. Mittal and A. K. Tyagi, J. Phys. Chem. C, 2012, 116, 12462–12467 CrossRef CAS.
- S. Onsuratoom, T. Puangpetch and S. Chavadej, Chem. Eng. J., 2011, 173, 667–675 CrossRef CAS.
- M. Murdoch, G. I. N. Waterhouse, M. A. Nadeem, J. B. Metson, M. A. Keane, R. F. Howe, J. Llorca and H. Idriss, Nat. Chem., 2011, 3, 489–492 CrossRef CAS PubMed.
- S. Hong, D. P. Kumar, E. H. Kim, H. Park, M. Gopannagari, D. A. Reddy and T. K. Kim, J. Mater. Chem. A, 2017, 5, 20851–20859 RSC.
- S. Zhang, X. Liu, C. Liu, S. Luo, L. Wang, T. Cai, Y. Zeng, J. Yuan, W. Dong, Y. Pei and Y. Liu, ACS Nano, 2018, 12, 751–758 CrossRef CAS PubMed.
- H. Han, K. M. Kim, C. W. Lee, C. S. Lee, R. C. Pawar, J. L. Jones, Y. R. Hong, J. H. Ryu, T. Song, S. H. Kang, H. Choi and S. Mhin, Phys. Chem. Chem. Phys., 2017, 19, 28207–28215 RSC.
- X. H. Qian, J. Y. Zhang, Z. Guo, S. Z. Liu, J. X. Liu and J. Lin, Appl.
Surf. Sci., 2019, 481, 795–801 CrossRef CAS.
- D. A. Reddy, H. Park, S. Hong, D. P. Kumar and T. K. Kim, J. Mater. Chem. A, 2017, 5, 6981–6991 RSC.
- Y. Lu, D. Chu, M. Zhu, Y. Du and P. Yang, Phys. Chem. Chem. Phys., 2015, 17, 17355–17361 RSC.
- J. Y. Zhao, X. M. Yan, N. Zhao, X. Li, B. Lu, X. H. Zhang and H. T. Yu, RSC Adv., 2020, 10, 32652–32661 RSC.
- Z. Sun, Q. Yue, J. Li, J. Xu, H. Zheng and P. Du, J. Mater. Chem. A, 2015, 3, 10243–10247 RSC.
- K. H. Sun, J. Shen, Y. T. Yang, H. Tang and C. S. Lei, Appl. Surf. Sci., 2020, 505, 144042 CrossRef CAS.
- J. G. Yu, Y. Hai and B. Cheng, J. Phys. Chem. C, 2011, 115, 4953–4958 CrossRef CAS.
- J. R. Ran, J. G. Yu and M. Jaroniec, Green Chem., 2011, 13, 2708–2713 RSC.
- S. S. Li, D. S. Dai, L. Ge, Y. Q. Gao, C. C. Han and N. Xiao, Dalton Trans., 2017, 46, 10620–10629 RSC.
- R. Y. Cao, H. C. Yang, S. W. Zhang and X. J. Xu, Appl. Catal., B, 2019, 258, 117997 CrossRef CAS.
- X. A. Zhou, J. Jin, X. J. Zhu, J. Huang, J. G. Yu, W. Y. Wong and W. K. Wong, J. Mater. Chem. A, 2016, 4, 5282–5287 RSC.
- J. G. Yu, S. H. Wang, B. Cheng, Z. Lin and F. Huang, Catal. Sci. Technol., 2013, 3, 1782–1789 RSC.
- L. R. Nagappagari, S. Samanta, N. Sharma, V. R. Battula and K. Kailasam, Sustainable Energy Fuels, 2020, 4, 750–759 RSC.
- X. Gou, F. Cheng, Y. Shi, L. Zhang and P. Shen, J. Am. Chem. Soc., 2006, 128, 7222–7229 CrossRef CAS PubMed.
- Z. Z. Zhang, L. Huang, J. J. Zhang, F. J. Wang, Y. Y. Xie, X. T. Shang, Y. Y. Gu, H. B. Zhao and X. X. Wang, Appl. Catal., B, 2018, 233, 112–119 CrossRef CAS.
- M. H. Xiong, B. Chai, J. T. Yan, G. Z. Fan and G. S. Song, Appl. Surf. Sci., 2020, 514, 145965 CrossRef CAS.
- J. G. Hou, C. Yang, H. J. Cheng, Z. Wang, S. Q. Jiao and H. M. Zhu, Phys. Chem. Chem. Phys., 2013, 15, 15660–15668 RSC.
- C. Liu, B. Chai, C. L. Wang, J. T. Yan and Z. D. Ren, Int. J. Hydrogen Energy, 2018, 43, 6977–6986 CrossRef CAS.
- J. Y. Liu, W. J. Fang, Z. D. Wei and Z. Qin, Catal. Sci. Technol., 2018, 8, 1375–1382 RSC.
- Z. P. Yan, X. X. Yu, A. Han, P. Xu and P. W. Du, J. Phys. Chem. C, 2014, 118, 22896–22903 CrossRef CAS.
- Q. W. Liu, M. D. Wang, Y. S. He, X. X. Wang and W. Y. Su, Nanoscale, 2018, 10, 19100–19106 RSC.
- S. Wang, B. Y. Guan, Y. Lu and X. W. Lou, J. Am. Chem. Soc., 2017, 139, 17305–17308 CrossRef CAS PubMed.
- B. Qiu, Q. Zhu, M. Du, L. Fan, M. Xing and J. Zhang, Angew. Chem., Int. Ed., 2017, 56, 2684–2688 CrossRef CAS PubMed.
- L. L. Bi, D. D. Meng, Q. J. Bu, Y. H. Lin, D. J. Wang and T. F. Xie, Phys. Chem. Chem. Phys., 2016, 18, 31534–31541 RSC.
- A. J. Bard, R. Parsons and J. Jordan, Standard Potentials in Aqueous Solution, Routledge, New York, 1985 Search PubMed.
- M. S. Nasir, G. R. Yang, L. Ayub, S. L. Wang and W. Yan, Appl. Surf. Sci., 2020, 519, 146208 CrossRef CAS.
Footnote |
† Electronic supplementary information (ESI) available. See DOI: 10.1039/d1ra01648b |
|
This journal is © The Royal Society of Chemistry 2021 |