DOI:
10.1039/D1RA01360B
(Paper)
RSC Adv., 2021,
11, 14269-14275
Is natural fraxin an overlooked radical scavenger?†
Received
19th February 2021
, Accepted 2nd April 2021
First published on 15th April 2021
Abstract
Fraxin (FX) (7-hydroxy-6-methoxycoumarin 8-glucoside) is a typical natural product of the coumarin family. This compound was shown to protect endothelial cells from oxidative stress; however, the nature of its antioxidant properties is still ambiguous. In this study, we report on a systematic evaluation of the radical scavenging activity of FX using a two-tier protocol based on thermodynamic and kinetic calculations. The results show that FX has moderate activity in the aqueous physiological environment against a range of radicals including HO˙, CCl3O˙, CCl3OO˙, NO2,
,
and HOO˙. The latter was examined in detail due to the prevalence of HOO˙ as a source of oxidative stress in biological systems. HOO˙ scavenging activity was promising in the gas phase but low in physiological environments with koverall = 1.57 × 106, 3.13 × 102 and 2.68 × 103 M−1 s−1 in the gas phase, pentyl ethanoate and water solvents, respectively. The formal hydrogen transfer mechanism at the O7–H bond dominates the hydroperoxyl radical scavenging of FX in the nonpolar media, whereas, in the polar environment, the activity is exerted by the single electron transfer mechanism of the anion state. This activity falls behind typical antioxidants such as Trolox, ascorbic acid, and trans-resveratrol under the studied conditions. Thus FX may have multiple health benefits, but it is not an outstanding natural antioxidant.
1. Introduction
Fraxin (FX) (7-hydroxy-6-methoxycoumarin 8-glucoside) is a typical compound of the coumarin family.1–3 This compound was found in the ash (Fraxinus) bark, along with esculin in the bark of the horse-chestnut,4 Ulmus macrocarpa,5 and Stewartia koreana plants.6 FX exhibits antioxidant activity through inhibition of cyclo AMP phosphodiesterase enzyme7 and analgesic effects like nonsteroidal anti-inflammatory drugs.8 Fraxin also shows free radical scavenging activity at high concentrations (0.5 mM). It was shown that FX could protect against H2O2-induced cytotoxicity in human umbilical vein endothelial cells.4 However, FX exhibited low inhibition in the 1,1-diphenyl-2-picrylhydrazyl (DPPH) assay and low superoxide quenching activity compared to vitamin C or ascorbic acid.5,6 Thus, the details of antioxidant activity of FX need further investigation.
Recent studies showed that radical reactions could be modeled with high accuracy using quantum chemical methods and a combination of thermodynamic and kinetic modeling.9–13 This approach provides an efficient and practical protocol to screen the antioxidant activity of natural products in silico with high accuracy; this is particularly useful for evaluating the activity in physiological environments.10,14 The computational method was successfully applied to investigate the antioxidant activity of numerous organic compounds.9,11,14–16 Continuing our program of evaluating the antioxidant activity of natural products,11,17,18 in this study, we investigated the radical scavenging activity of FX against HOO˙, a typical radical that has widely used as a model to evaluated the antioxidant activity of organic compounds,9,10,14 using density functional theory (DFT) calculations. Using the overall radical scavenging characteristics hence established, the activity of FX against a broad range of oxidizing radicals and radical ions is also modeled in the aqueous environment (Fig. 1).
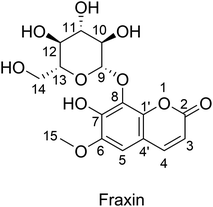 |
| Fig. 1 Molecular structure and the atomic numbering of fraxin (FX). | |
2. Computational details
All calculations were carried out with Gaussian 16 suite of programs19 using M06-2X functional.20 This functional was demonstrated to be highly accurate for both thermodynamic and kinetic calculations when modelling radical reactions.9,21 To save computing time, the M06-2X/6-311++G(d,p)//M06-2X/6-31+G(d) method9,20 was used to calculate the thermodynamic parameters, whereas the kinetic study was performed by the M06-2X/6-311++G(d,p) method.9,22,23 Solvent effects of water and pentyl ethanoate were modelled by solvation model density (SMD) method.10,24–29 The bond dissociation enthalpy (BDE), proton affinity (PA) and ionization energy (IE) were calculated as follows.17,30
BDE = H(FX˙) + H(H˙) − H(FX–H) |
PA = H(FX−) + H(H+) − H(FX–H) |
IE = H(FX–H+˙) + H(e−) − H(FX–H) |
Where H(FX–H), H(FX˙), H(FX−), H(FX–H+˙), H(H˙), and H(H+) are enthalpies of neutral molecule, radical, anion, cation-radical, hydrogen atom, and proton, respectively.
The kinetic calculations were performed following the quantum mechanics-based test for the overall free radical scavenging activity (QM-ORSA) protocol.10 All of the species (molecules, anions, radicals, reactants, pre-complexes, post-complexes, TSs and products) were optimized directly in the specific environments, i.e. in gas phase, pentyl ethanoate or water. The rate constant (k) was calculated using the conventional transition state theory (TST) and 1 M standard state at 298.15 K.28,29,31–36
|
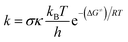 | (1) |
where
σ is the reaction symmetry number,
37,38 κ contains the tunneling corrections calculated using the Eckart barrier,
39 kB is the Boltzmann constant,
h is the Planck constant, Δ
G≠ is the Gibbs free energy of activation.
The Marcus Theory was used to estimate the reaction barriers of single electron transfer (SET) reactions.40–42 The free energy of reaction ΔG≠ for the SET pathway was computed following the eqn (2) and (3).
|
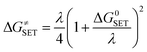 | (2) |
where Δ
GSET is the Gibbs energy of reaction, Δ
ESET is the non-adiabatic energy difference between reactants and vertical products for SET.
43,44
For rate constants that were close to the diffusion limit a correction was applied to yield realistic results.10 The apparent rate constants (kapp) were calculated following the Collins–Kimball theory in the solvents at 298.15 K;45 the steady-state Smoluchowski rate constant (kD) for an irreversible bimolecular diffusion-controlled reaction was calculated following the literature as corroding to eqn (4) and (5).10,46
|
 | (4) |
where
RAB is the reaction distance,
NA is the Avogadro constant, and
DAB =
DA +
DB (
DAB is the mutual diffusion coefficient of the reactants A and B),
45,47 where
DA or
DB is estimated using the Stokes–Einstein formulation (6).
48,49 |
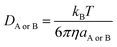 | (6) |
η is the viscosity of the solvents (
i.e. η(H
2O) = 8.91 × 10
−4 Pa s,
η(pentyl ethanoate) = 8.62 × 10
−4 Pa s) and
a is the radius of the solute.
The kinetic study requires different considerations. Water (dielectric constants, ε = 78.35) and pentyl ethanoate (ε = 4.73) are the de facto standard solvents in the literature to mimic the polar and nonpolar environments in the human body.10,14,50,51 Thus, these solvents were used to model the physiological environments. The solvent cage effects were included following the corrections proposed by Okuno,52 adjusted with the free volume theory according to the Benson correction10,53–55 to reduce over-penalizing entropy losses in solution. For the species that have multiple conformers, all of these were screened56 and the conformer with the lowest electronic energy was included in the analysis.50,51 The hindered internal rotation treatment was also applied to the single bonds to ensure that the obtained conformer has the lowest electronic energy.51,57 All transition states were characterized by the existence of only one single imaginary frequency. Intrinsic coordinate calculations (IRCs) were performed to ensure that each transition state is connected correctly with the pre-complex and post-complex.
3. Results and discussion
3.1. Free radical scavenging activity of FX in the gas phase
3.1.1. Thermodynamic evaluation. In the first step, the ability of FX (FX–H) to react (R˙) with free radicals by either of the three typical mechanisms:- Single electron transfer proton transfer (SETPT)58,59
|
FX–H˙+ + R− → FX˙ + RH
| (8) |
- Sequential proton loss electron transfer (SPLET)60–63
- Formal hydrogen transfer (FHT)9
|
FX–H + R˙ → FX˙ + RH
| (11) |
Thermochemical parameters (IE, PA and BDE) corresponding to three pathways were evaluated. Thus, the BDE, PA and IE values in the gas phase of all possible X–H (X = C, O) bonds were calculated and the results are shown in Table S2, ESI; † the lowest values are presented in the Table 1.
Table 1 The lowest values of the thermochemical parameters (BDE, PA and IE) of FX (in kcal mol−1) and the Gibbs free energy of the reaction with HOO˙ radical via the main mechanisms in the gas phase
FHT |
SPLET |
SETPT |
BDE (O7–H) |
ΔG° |
PA (O7–H) |
ΔG°a |
IE |
ΔG°a |
For the first step reaction of the mechanisms. |
89.4 |
2.6 |
327.0 |
176.1 |
175.4 |
152.5 |
The BDE values of the C–H bonds are in the range of 93.9 to 97.3 kcal mol−1, whereas those for the O–H bonds are around 89.4–109.6 kcal mol−1 (Table S2†). The lowest BDE is observed at the O7–H bond with BDE = 89.4 kcal mol−1. This value is much lower than the BDEs of any other moieties and thus clearly identifies the site of antiradical activity of FX according to the FHT mechanism.29 Therefore the kinetic study for the antiradical activity of FX following the mechanism in the gas phase and lipid should be performed for this bond.
Previous studies suggested that in aromatic antioxidants, the phenolic O–H bond is the most likely deprotonation site in the gas phase.17,18,29,64 Therefore the PA values were computed for the O7–H bond only, yielding PA = 327.0 kcal mol−1. The computed IE value is 175.4 kcal mol−1. Comparing the data reveals that PA and IE values are about 3.7 and 1.9 times higher than the lowest BDE value (BDE(O7–H) = 89.4 kcal mol−1), thus the antioxidant activity of FX in the gas phase is dominated by the FHT pathway.
The calculated Gibbs free energies (ΔG°) of the reactions of FX with HOO˙ radical via FHT, proton loss (the first step of the SPLET mechanism) and single electron transfer (the first step of the SETPT) (Table 1) also showed that the SPLET and SETPT mechanisms are not thermodynamically favorable in the gas phase (ΔG° = 176.1 and 152.5 kcal mol−1 for the sequential proton and the single electron transfer, respectively). The Gibbs free energy of the reaction of FX with HOO˙ radical via the FHT mechanism is the lowest at O7–H with ΔG° = 2.6 kcal mol−1. Knowing that FX does have reported antioxidant activity and that the thermodynamic descriptors calculated herein are reduced by the dielectric constant of the environment, there is a high likelihood that the reactions with small positive ΔG° (i.e. O7–H) do contribute to the radical scavenging activity of FX in media. Modelling the entire reaction as in the kinetics calculations is more accurate than the thermodynamic modeling shown here, and therefore there is a possibility that the reaction might proceed in spite of the small positive ΔG°. Thus, the FHT mechanism at the O7–H bond will be included in further investigations.
3.1.2. Kinetic study. As concluded in the previous section, the HOO˙ antiradical activity of FX in the gas phase may be possible via the FHT mechanism at the O7–H bond. Thus, in this section, the kinetics of the FX + HOO˙ reaction was evaluated for this bond by following the (QM-ORSA) protocol and the M06-2X/6-311++G(d,p) method.9,10,22 The results are presented in Fig. 2 and Table 2.
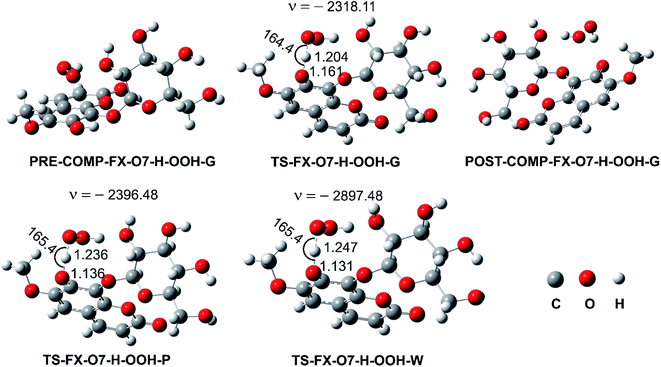 |
| Fig. 2 Optimized geometries of the pre-complex (PRE-COMP), TSs, post-complex (POST-COMP) between the FX–O7–H + HOO˙ reaction following the FHT mechanism (G: gas phase, W: water, P: pentyl ethanoate). | |
Table 2 Calculated ΔH (kcal mol−1), ΔG≠ (kcal mol−1), tunneling corrections (κ), and kEck (M−1 s−1) for the FX + HOO˙ reaction in the gas phase
Reactions |
ΔH |
ΔG≠ |
κ |
kEck |
FX–O7–H + HOO˙ |
0.7 |
12.2 |
213.6 |
1.57 × 106 |
It is found that the H− abstraction at the O7–H bond against HOO˙ radical in the gas phase occurs with ΔG≠ = 12.2 kcal mol−1 and rate constant kEck = 1.57 × 106 M−1 s−1. This value is slightly lowest than that of the reference antioxidant Trolox (kEck = 1.87 × 107 M−1 s−1).17
3.2. Free radical scavenging activity of FX in physiological environments
3.2.1. Acid-base equilibrium. As FX is a phenolic compound, the deprotonation of the OH moieties must be considered in the evaluation of the antioxidant activity in aqueous solution.9,22 The initial calculation of PA values showed that the site most likely to dissociate is the O7–H bond. Thus, this bond was used to calculate the pKa of FX. The pKa was computed following the literature,22,64 the molar fractions (% A− and % HA) were computed following eqn (12) and the results are shown in Fig. 3. |
 | (12) |
where [H+] = 3.98 × 10−8 M (at water pH = 7.4) and Ka = 10−pKa
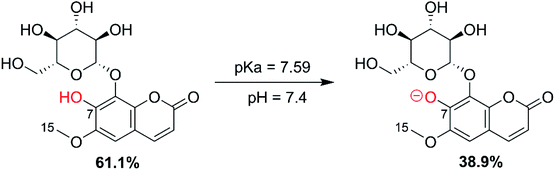 |
| Fig. 3 The acid dissociation equilibrium of FX at pH = 7.4. | |
The calculated pKa value was 7.59; thus, in water at pH = 7.4, FX exists in neutral (HA, 61.1%) and anion (A−, 38.9%) states. In the lipid medium (i.e. pentyl ethanoate solvent), FX exists in a neutral state (HA). Therefore, these states were used in the following kinetic study in physiological environments.
3.2.2. Kinetic study in physiological environments. Based on the kinetic study results in the gas phase, the HOO˙ antiradical activity in lipid media was modeled by the FHT mechanism at the O7–H bond. As it was shown in previous studies,9,17,18 the SPLET pathway is frequently the dominant process in aqueous environment, because the first step, proton dissociation, takes place spontaneously; the second step is essentially the transfer of an electron between the radical and the anion and hence this activity of the anionic species in water is often called single electron transfer (SET) mechanism. Therefore, in the aqueous environment, the SET mechanism was also investigated for the states (HA and A−) of FX.The overall rate constants (koverall) were computed following the (QM-ORSA) protocol with the M06-2X/6-311++G(d,p) method,10,27 (Table 3) according to eqn (13) and (14).
Table 3 Gibbs free energies of activation (ΔG≠, kcal mol−1), tunneling corrections (κ), rate constants (kapp, kf, koverall, M−1 s−1), and branching ratios (Γ, %) at 298.15 K for the FX + HOO˙ reaction in water and pentyl ethanoate solvents at the M06-2X/6-311++G(d,p) method
Mechanism |
Pentyl ethanoate |
Water |
ΔG≠ |
κ |
kapp |
States |
ΔG≠ |
κ |
kapp |
f |
kfb |
Γ |
The nuclear reorganization energy (λ, kcal mol−1). kf = f.kapp, f = % A−(HA)/100; Γ = kf × 100/koverall. |
SET |
|
|
|
HA |
35.4 |
21.5a |
8.60 × 10−14 |
0.611 |
5.25 × 10−14 |
0.0 |
|
|
|
A− |
12.2 |
16.1a |
6.70 × 103 |
0.389 |
2.61 × 103 |
97.3 |
FHT |
17.4 |
286.7 |
3.13 × 102 |
HA |
18.1 |
347.4 |
1.20 × 102 |
0.611 |
7.33 × 101 |
2.7 |
koverall |
|
|
3.13 × 102 |
|
|
|
|
|
2.68 × 103 |
|
In lipid medium:
|
koverall = kapp(FHT(O7–H)-neutral)
| (13) |
In the aqueous solution:
|
koverall = kf(SET-HA) + kf(SET-A−) + kf(FHT-neutral)
| (14) |
As per Table 3, the HOO˙ radical scavenging activity of FX in the lipid medium is moderate with the overall rate constant koverall = 3.13 × 102 M−1 s−1. Thus, as suggested by the gas phase results, FX is not as good HOO˙ radical scavenger as Trolox (koverall = 1.00 × 105 M−1 s−1)17 in lipid medium. At the same time, FX exhibits moderate HOO˙ antiradical activity in the aqueous solution with koverall = 2.68 × 103 M−1 s−1. The donated electron of anion A− plays a dominant role (∼97.3%) in the HOO˙ antiradical activity of FX. The rate constant for the H-abstraction of O7–H bond against HOO˙ radical is kf = 7.33 × 101 M−1 s−1; however, this reaction plays only a minor role (∼2.7%) in the HOO˙ antiradical activity of FX. The HOO˙ radical scavenging activity of FX in the physiological environments is lower than that of the reference antioxidants Trolox (k = 1.00 × 105 and 1.30 × 105 M−1 s−1),17 ascorbic acid (k = 5.71 × 103 and 1.00 × 108 M−1 s−1),10 and trans-resveratrol (k = 1.31 × 104 and 5.62 × 107 M−1 s−1, in pentyl ethanoate and water, respectively).65 Previous studies established that primary antioxidants are inefficient if the rate constant for scavenging the reference alkylperoxy radical is less than 1.18 × 103 M−1 s−1.10,66 Based on that, FX cannot be considered an efficient radical scavenger in the physiological environments.
3.2.3. The radical scavenging activity of FX against ordinary free radicals in aqueous solution. While the activity against HOO˙ is used as a benchmark, variations do exist in the radical scavenging reactions against other species. Therefore the radical scavenging activity of FX was modeled against typical free radicals HO˙, CH3O˙, HOO˙, CH3OO˙, CCl3OO˙, NO, NO2 (these oxides of nitrogen are free radicals but that is normally not indicated in the formula),
,
, and
; the interaction of the anion state of FX (A−) and these radicals was also investigated following the primary mechanism in the aqueous phase (the SET mechanism) at pH = 7.4 and the results are shown in Table 4.
Table 4 Calculated ΔG≠, λ, (kcal mol−1), the nuclear reorganization energy (λ, kcal mol−1), the diffusion-limited rate constant (kD), kapp and kf (M−1 s−1) of the reaction between A− and selected radicals following the SET mechanism in aqueous solution at pH 7.4
Radical |
ΔG≠ |
λ |
kD |
kapp |
kfa |
kf = f.kapp; f(A−) = 0.389. |
HO˙ |
2.1 |
4.2 |
8.70 × 109 |
8.30 × 109 |
3.23 × 109 |
CH3O˙ |
6.7 |
5.3 |
8.20 × 109 |
7.20 × 107 |
2.80 × 107 |
CCl3O˙ |
0.3 |
51.9 |
7.70 × 109 |
7.60 × 109 |
2.96 × 109 |
HOO˙ |
12.2 |
16.1 |
8.00 × 109 |
6.70 × 103 |
2.61 × 103 |
CH3OO˙ |
13.9 |
15.5 |
8.00 × 109 |
4.20 × 102 |
1.63 × 102 |
CCl3OO˙ |
1.0 |
17.6 |
7.70 × 109 |
7.60 × 109 |
2.96 × 109 |
NO |
111.7 |
15.1 |
8.40 × 109 |
8.10 × 10−70 |
3.15 × 10−70 |
NO2 |
3.4 |
28.5 |
8.20 × 109 |
5.90 × 109 |
2.30 × 109 |
 |
64.9 |
17.9 |
8.30 × 109 |
1.60 × 10−35 |
6.22 × 10−36 |
 |
2.4 |
18.4 |
7.90 × 109 |
7.30 × 109 |
2.84 × 109 |
 |
1.6 |
3.2 |
8.10 × 109 |
7.90 × 109 |
3.07 × 109 |
As shown in Table 4, the calculations predict that FX should have good activity against HO˙, CCl3O˙, CCl3OO˙, NO2,
and
radicals with the kf in the range of 2.30 × 109–3.23 × 109 M−1 s−1 (∼kD), whereas NO and
radicals could not be eliminated under the studied conditions. The good HO˙ radical scavenging activity of FX in the aqueous solution may explain the experimental observations regarding the antioxidant activity of FX in human umbilical vein endothelial cells, where it was protective against H2O2-mediated oxidative stress.4
4. Conclusion
The antioxidant activity of fraxin was investigated using thermodynamic and kinetic calculations. The results showed that FX had average HOO˙ scavenging activity in the studied environments with koverall = 1.57 × 106, 3.13 × 102 and 2.68 × 103 M−1 s−1 in the gas phase, pentyl ethanoate and water solvents, respectively. The FHT mechanism via the O7–H bond dominates the hydroperoxyl radical scavenging of FX in the lipid media; however, in the aqueous solution, the activity is defined by the single electron transfer mechanism of the anion state. It was found that FX exhibited good activity against HO˙, CCl3O˙, CCl3OO˙, NO2,
and
radicals with the kf in the range of 2.30 × 109–3.23 × 109 M−1 s−1. FX is thus a weak antioxidant and cannot compete with the reference compounds Trolox, ascorbic acid, or trans-resveratrol.
Conflicts of interest
There are no conflicts to declare.
Acknowledgements
The research is funded by Vietnam National Foundation for Science and Technology Development (NAFOSTED) under grant number 104.06-2020.17 (P. C. N).
References
- B. Y. Chang, Y. S. Jung, C. S. Yoon, J. S. Oh, J. H. Hong, Y. C. Kim and S. Y. Kim, Molecules, 2017, 22 Search PubMed.
- A. M. Hirsch, A. Longeon and M. Guyot, Biochem. Syst. Ecol., 2002, 30, 55–60 CrossRef CAS.
- G. Stanic, B. Jurisic and D. Brkic, Croat. Chem. Acta, 1999, 72, 827–834 CAS.
- W. K. Whang, H. S. Park, I. Ham, M. Oh, H. Namkoong, H. K. Kim, D. W. Hwang, S. Y. Hur, T. E. Kim, Y. G. Park, J. R. Kim and J. W. Kim, Exp. Mol. Med., 2005, 37, 436–446 CrossRef CAS PubMed.
- J.-H. Kwon, S.-B. Kim, K.-H. Park and M.-W. Lee, Arch. Pharmacal Res., 2011, 34, 1459 CrossRef CAS PubMed.
- J. H. Y. Sa, Im Lee and D. K. Kim, Biomol. Ther., 2010, 18, 191–196 CrossRef.
- H. Schempp, D. Weiser and E. F. Elstner, Arzneimittelforschung, 2000, 50, 362–372 CAS.
- C. Klein-Galczinsky, Wien. Med. Wochenschr., 1999, 149, 248 CAS.
- A. Galano and J. R. Alvarez-Idaboy, J. Comput. Chem., 2014, 35, 2019–2026 CrossRef CAS PubMed.
- A. Galano and J. R. Alvarez-Idaboy, J. Comput. Chem., 2013, 34, 2430–2445 CrossRef CAS PubMed.
- H. Boulebd, A. Mechler, N. T. Hoa and Q. V. Vo, New J. Chem., 2020, 44, 9863–9869 RSC.
- A. Schumann-Gillett and M. L. O'Mara, Biochim. Biophys. Acta, 2019, 1861, 210–219 CrossRef CAS PubMed.
- M. Thomas, T. Anglim Lagones, M. Judd, M. Morshedi, M. L. O'Mara and N. G. White, Chem.–Asian J., 2017, 12, 1587–1597 CrossRef CAS PubMed.
- A. Galano and J. Raúl Alvarez-Idaboy, Int. J. Quantum Chem., 2019, 119, e25665 CrossRef.
- M. Cordova-Gomez, A. Galano and J. R. Alvarez-Idaboy, RSC Adv., 2013, 3, 20209–20218 RSC.
- M. Carreon-Gonzalez, A. Vivier-Bunge and J. R. Alvarez-Idaboy, J. Comput. Chem., 2019, 15, 2103–2110 CrossRef PubMed.
- Q. V. Vo, N. M. Thong, T. Le Huyen, P. C. Nam, N. M. Tam, N. T. Hoa and A. Mechler, RSC Adv., 2020, 10, 20089–20097 RSC.
- Q. V. Vo, P. C. Nam, M. Van Bay, N. M. Thong and A. Mechler, RSC Adv., 2019, 9, 42020–42028 RSC.
- M. J. Frisch, G. W. Trucks, H. B. Schlegel, G. E. Scuseria, M. A. Robb, J. R. Cheeseman, G. Scalmani, V. Barone, B. Mennucci, G. A. Petersson, H. Nakatsuji, M. Caricato, X. Li, H. P. Hratchian, A. F. Izmaylov, G. Z. J. Bloino, J. L. Sonnenberg, M. Hada, M. Ehara, K. Toyota, R. Fukuda, J. Hasegawa, M. Ishida, T. Nakajima, Y. Honda, O. Kitao, H. Nakai, T. Vreven, J. A. Montgomery Jr, J. E. Peralta, F. Ogliaro, M. Bearpark, J. J. Heyd, E. Brothers, K. N. Kudin, V. N. Staroverov, T. Keith, R. Kobayashi, J. Normand, K. Raghavachari, A. Rendell, J. C. Burant, S. S. Iyengar, J. Tomasi, M. Cossi, N. Rega, J. M. Millam, M. Klene, J. E. Knox, J. B. Cross, V. Bakken, C. Adamo, J. Jaramillo, R. Gomperts, R. E. Stratmann, O. Yazyev, A. J. Austin, R. Cammi, C. Pomelli, J. W. Ochterski, R. L. Martin, K. Morokuma, V. G. Zakrzewski, G. A. Voth, P. Salvador, J. J. Dannenberg, S. Dapprich, A. D. Daniels, O. Farkas, J. B. Foresman, J. V. Ortiz, J. Cioslowski and D. J. Fox, Gaussian 16, Revision A.02, Gaussian Inc., Wallingford CT Search PubMed.
- Y. Zhao and D. G. Truhlar, J. Phys. Chem. A, 2008, 112, 1095–1099 CrossRef CAS PubMed.
- Y. Zhao and D. G. Truhlar, Theor. Chem. Acc., 2008, 120, 215–241 Search PubMed.
- Q. V. Vo, N. T. Hoa, P. C. Nam, D. T. Quang and A. Mechler, ACS Omega, 2020, 5, 24106–24110 Search PubMed.
- H. Boulebd, J. Biomol. Struct. Dyn., 2020, 1–10 Search PubMed.
- A. V. Marenich, C. J. Cramer and D. G. Truhlar, J. Phys. Chem. B, 2009, 113, 6378–6396 CrossRef CAS PubMed.
- C. P. Kelly, C. J. Cramer and D. G. Truhlar, J. Chem. Theory Comput., 2005, 1, 1133–1152 CrossRef CAS PubMed.
- J. R. l. Alvarez-Idaboy and A. Galano, J. Phys. Chem. B, 2012, 116, 9316–9325 CrossRef CAS PubMed.
- M. E. Alberto, N. Russo, A. Grand and A. Galano, Phys. Chem. Chem. Phys., 2013, 15, 4642–4650 RSC.
- E. Dzib, J. L. Cabellos, F. Ortíz-Chi, S. Pan, A. Galano and G. Merino, Int. J. Quantum Chem., 2019, 119, e25686 CrossRef.
- Q. V. Vo, M. V. Bay, P. C. Nam, D. T. Quang, M. Flavel, N. T. Hoa and A. Mechler, J. Org. Chem., 2020, 85, 15514–15520 CrossRef CAS PubMed.
- Q. V. Vo and A. Mechler, J. Chem. Inf. Model., 2019, 60, 316–321 CrossRef PubMed.
- M. G. Evans and M. Polanyi, Trans. Faraday Soc., 1935, 31, 875–894 RSC.
- H. Eyring, J. Chem. Phys., 1935, 3, 107–115 CrossRef CAS.
- D. G. Truhlar, W. L. Hase and J. T. Hynes, J. Phys. Chem., 1983, 87, 2664–2682 CrossRef CAS.
- T. Furuncuoglu, I. Ugur, I. Degirmenci and V. Aviyente, Macromolecules, 2010, 43, 1823–1835 CrossRef CAS.
- E. Vélez, J. Quijano, R. Notario, E. Pabón, J. Murillo, J. Leal, E. Zapata and G. Alarcón, J. Phys. Org. Chem., 2009, 22, 971–977 CrossRef.
- E. Dzib, J. L. Cabellos, F. Ortiz-Chi, S. Pan, A. Galano and G. Merino, Eyringpy 1.0.2, 2018, Cinvestav, Mérida, Yucatán Search PubMed.
- E. Pollak and P. Pechukas, J. Am. Chem. Soc., 1978, 100, 2984–2991 CrossRef CAS.
- A. Fernández-Ramos, B. A. Ellingson, R. Meana-Pañeda, J. M. Marques and D. G. Truhlar, Theor. Chem. Acc., 2007, 118, 813–826 Search PubMed.
- C. Eckart, Phys. Rev., 1930, 35, 1303 CrossRef CAS.
- R. A. Marcus, Annu. Rev. Phys. Chem., 1964, 15, 155–196 CrossRef CAS.
- R. A. Marcus, Rev. Mod. Phys., 1993, 65, 599 CrossRef CAS.
- Y. Lu, A. Wang, P. Shi, H. Zhang and Z. Li, PLoS One, 2015, 10, e0133259 CrossRef PubMed.
- S. F. Nelsen, S. C. Blackstock and Y. Kim, J. Am. Chem. Soc., 1987, 109, 677–682 CrossRef CAS.
- S. F. Nelsen, M. N. Weaver, Y. Luo, J. R. Pladziewicz, L. K. Ausman, T. L. Jentzsch and J. J. O'Konek, J. Phys. Chem. A, 2006, 110, 11665–11676 CrossRef CAS PubMed.
- F. C. Collins and G. E. Kimball, J. Colloid Sci., 1949, 4, 425–437 CrossRef CAS.
- M. Von Smoluchowski, Z. Physiol. Chem., 1917, 92, 129–168 CAS.
- D. G. Truhlar, J. Chem. Educ., 1985, 62, 104 CrossRef CAS.
- A. Einstein, Ann. Phys., 1905, 17, 549–560 CrossRef CAS.
- G. G. Stokes, Mathematical and Physical Papers, University Press, Cambridge, 1905 Search PubMed.
- Q. V. Vo, T. V. Gon, M. V. Bay and A. Mechler, J. Phys. Chem. B, 2019, 123, 10672–10679 CrossRef CAS PubMed.
- H. Boulebd, I. Amine Khodja, M. V. Bay, N. T. Hoa, A. Mechler and Q. V. Vo, J. Phys. Chem. B, 2020, 124, 4123–4131 CrossRef CAS PubMed.
- Y. Okuno, Chem.–Eur. J., 1997, 3, 212–218 CrossRef CAS PubMed.
- S. Benson, The foundations of chemical kinetics, Malabar, Florida, 1982 Search PubMed.
- C. Iuga, J. R. Alvarez-Idaboy and A. Vivier-Bunge, J. Phys. Chem. B, 2011, 115, 12234–12246 CrossRef CAS PubMed.
- J. R. Alvarez-Idaboy, L. Reyes and N. Mora-Diez, Org. Biomol. Chem., 2007, 5, 3682–3689 RSC.
- W. Hehre, J. Yu, P. Klunzinger and L. Lou, Spartan Software, Wavefunction, Inc., Irvine, 2000.
- T. H. Le, T. T. Tran and L. K. Huynh, Chemom. Intell. Lab. Syst., 2018, 172, 10–16 CrossRef CAS.
- J. S. Wright, E. R. Johnson and G. A. DiLabio, J. Am. Chem. Soc., 2001, 123, 1173–1183 CrossRef CAS PubMed.
- K. U. Ingold and D. A. Pratt, Chem. Rev., 2014, 114, 9022–9046 CrossRef CAS PubMed.
- B. N. Ames, M. K. Shigenaga and T. M. Hagen, Proc. Natl. Acad. Sci. U. S. A., 1993, 90, 7915–7922 CrossRef CAS PubMed.
- G. Wang, Y. Xue, L. An, Y. Zheng, Y. Dou, L. Zhang and Y. Liu, Food Chem., 2015, 171, 89–97 CrossRef CAS PubMed.
- L. Estévez, N. Otero and R. A. Mosquera, J. Phys. Chem. B, 2010, 114, 9706–9712 CrossRef PubMed.
- Y.-Z. Zheng, G. Deng, R. Guo, Z.-M. Fu and D.-F. Chen, Phytochemistry, 2019, 166, 112075 CrossRef CAS PubMed.
- A. Galano, A. Pérez-González, R. Castañeda-Arriaga, L. Muñoz-Rugeles, G. Mendoza-Sarmiento, A. Romero-Silva, A. Ibarra-Escutia, A. M. Rebollar-Zepeda, J. R. León-Carmona, M. A. Hernández-Olivares and J. R. Alvarez-Idaboy, J. Chem. Inf. Model., 2016, 56, 1714–1724 CrossRef CAS PubMed.
- C. Iuga, J. R. l. Alvarez-Idaboy and N. Russo, J. Org. Chem., 2012, 77, 3868–3877 CrossRef CAS PubMed.
- S. Itagaki, T. Kurokawa, C. Nakata, Y. Saito, S. Oikawa, M. Kobayashi, T. Hirano and K. Iseki, Food Chem., 2009, 114, 466–471 CrossRef CAS.
Footnote |
† Electronic supplementary information (ESI) available. See DOI: 10.1039/d1ra01360b |
|
This journal is © The Royal Society of Chemistry 2021 |