DOI:
10.1039/D1RA01189H
(Paper)
RSC Adv., 2021,
11, 18870-18880
Synthesis, optical and electrochemical properties of 4,4′-bibenzo[c]thiophene derivatives†
Received
13th February 2021
, Accepted 19th May 2021
First published on 25th May 2021
Abstract
We designed and synthesized unsubstituted 4,4′-bibenzo[c]thiophene 4,4′-BBT and its silyl-substituted derivatives 1,1′-Si-4,4′-BBT and 1,1′,3,3′-Si-4,4′-BBT with one or two tert-butyldimethylsilyl groups on each thiophene ring, as new π-building blocks in emitters, photosensitizers and semiconductors for organic optoelectronic devices. The characterization of 4,4′-BBT, 1,1′-Si-4,4′-BBT and 1,1′,3,3′-Si-4,4′-BBT was successfully determined by FTIR, 1H and 13C NMR measurements, high-resolution mass spectrometry (HRMS) analysis, photoabsorption and fluorescence spectroscopy, cyclic voltammetry (CV) and density functional theory (DFT) calculations. Moreover, a single-crystal X-ray structural analysis was successfully made for 1,1′-Si-4,4′-BBT and 1,1′,3,3′-Si-4,4′-BBT. The photoabsorption and fluorescence maxima (λabsmax and λflmax) of the three 4,4′-bibenzo[c]thiophene derivatives in toluene exhibit bathochromic shifts in the order of 4,4′-BBT (359 nm and 410 nm) < 1,1′-Si-4,4′-BBT (366 nm and 420 nm) < 1,1′,3,3′-Si-4,4′-BBT (371 nm and 451 nm). The HOMO and LUMO energy levels rise in the order of 4,4′-BBT (−5.55 eV and −2.39 eV) < 1,1′-Si-4,4′-BBT (−5.45 eV and −2.34 eV) < 1,1′,3,3′-Si-4,4′-BBT (−5.34 eV and −2.30 eV), but the rise of the HOMO energy level is larger than that of the LUMO energy level, resulting in the bathochromic shift of the photoabsorption band from 4,4′-BBT to 1,1′,3,3′-Si-4,4′-BBT. The fluorescence quantum yields (Φfl) of 4,4′-BBT, 1,1′-Si-4,4′-BBT and 1,1′,3,3′-Si-4,4′-BBT in toluene are 0.41, 0.41 and 0.36, respectively. It is worth mentioning that in the solid state 1,1′-Si-4,4′-BBT and 1,1′,3,3′-Si-4,4′-BBT show relatively high Φfl-solid values of 0.22 and 0.25, respectively, whereas 4,4′-BBT exhibits poor solid-state fluorescence properties (Φfl-solid < 0.02). This work provides an efficient synthetic method for the 4,4′-bibenzo[c]thiophene derivatives and their photophysical properties in the solution and solid state, electrochemical properties and X-ray crystal structures.
Introduction
Benzo[b]thiophene as a π-building block has created considerable scientific interest in synthetic organic chemistry, photochemistry, electrochemistry and theoretical chemistry as well as materials chemistry, because it is an especially crucial player for functional materials due to the air-stability and commercial availability (Fig. 1).1 Actually, benzo[b]thiophene derivatives are key constituents of emitters, semiconductors and photosensitizers for organic optoelectronic devices, such as organic light-emitting diodes (OLEDs),2 organic field-effect transistors (OFETs),3 organic photovoltaics (OPVs)4 and dye-sensitized solar cells (DSSCs).5 Furthermore, much effort has been made towards the construction and characterization of fused benzo[b]thiophene systems such as thienoacenes (e.g., [1]benzothieno[3,2-b][1]benzothiophene (BTBT), dinaphtho[2,3-b:2′,3′-f]thieno[3,2-b]thiophene (DNTT) and dianthra[2,3-b:2′,3′-f]thieno[3,2-b]thiophene (DATT))6a and thiophene-fused naphtho[2,3-b:6,7-b]dithiophene diimide (NDTI)6b in the past two decades. Indeed, these benzo[b]thiophene derivatives have been used as organic semiconductors with high carrier mobility and stability under ambient conditions. Similarly, benzo[c]thiophene is also an interesting π-building block (Fig. 1). For example, Wudl and Heeger have prepared poly(1,3-benzo[c]thiophene) (i.e., poly(isothianaphthene) (PITN)) and demonstrated that PITN can form the aromatic and quinoidal states by the bond alternation.7 In the aromatic state, the benzo[c]thiophene unit contains a thiophene ring in the structure. On the other hand, in the quinoidal state, the unit contains the more stable benzene ring in the structure. Thus, PITN has a low band gap (Eg = 1.0–1.2 eV), which is about 1.0 eV lower than that of polythiophene (PT), because the polymer backbone of PITN intrinsically stabilizes its quinoidal state, that is, the contribution of the quinoidal state decreases the Eg. However, unsubstituted benzo[c]thiophene has never been isolated so far due to the instability in air, in contrast to unsubstituted benzo[b]thiophene. Nevertheless, some benzo[c]thiophene derivatives with substituents on the thiophene ring and/or the benzene ring such as 1,3-diarylbenzo[c]thiophenes,8–12 5,6-disubstituted benzo[c]thiophenes13 and 1,3,4,7- or 1,3,5,6-tetrasubstituted benzo[c]thiophenes14–16 have been synthesized and their optical and electrochemical properties were investigated, although much less substituted benzo[c]thiophene derivatives have been reported than substituted benzo[b]thiophene derivatives. In particular, few 3,3′-disubstituted-1,1′-bibenzo[c]thiophenes as the 1,1′-dimer of bibenzo[c]thiophene have been developed by Cava,17 Mohanakrishnan18 and Ono et al.,19 and they revealed their synthetic methods and, optical and electrochemical properties, although there are no reports on the synthesis and physical properties of unsubstituted 1,1′-bibenzo[c]thiophene 1,1′-BBT (Fig. 1). On the other hand, in our previous work,20 we have designed and developed 1,1′-bis(tert-butyldimethylsilyl)-4,4′-bibenzo[c]thiophene (1,1′-Si-4,4′-BBT) as the 4,4′-dimer of bibenzo[c]thiophene and the fused-bibenzo[c]thiophene (PHDT-Si), which is the first report on the synthesis, characterization and optical and electrochemical properties of 4,4′-bibenzo[c]thiophene and fused-bibenzo[c]thiophene derivatives (Fig. 2). PHDT-Si exhibits intense vibronic-structured photoabsorption (λabsmax = 598 nm, molar extinction coefficient (εmax) = 80
900 M−1 cm−1 in toluene) and fluorescence (λflmax = 613 nm, fluorescence quantum yield (Φfl) = 0.74 in toluene) bands in a significantly longer wavelength region and a smaller Stokes shift (409 cm−1), compared to those of 1,1′-Si-4,4′-BBT (λabsmax = 366 nm, λflmax = 420 nm, εmax = 14
400 M−1 cm−1, Φfl = 0.41, Stokes shift = 3513 cm−1 in toluene). It is worth noting here that 1,1′-Si-4,4′-BBT exhibits the λabsmax in a shorter wavelength region by 40 nm in comparison with that of the isomer 3,3′-bis(tert-butyldimethylsilyl)-1,1′-bibenzo[c]thiophene 3,3′-Si-1,1′-BBT (λabsmax = 406 nm, εmax = ca. 11
500 M−1 cm−1 in CH2Cl2) reported by Cava et al.17a However, in order to use extensively and commonly benzo[c]thiophene derivatives as π-building blocks in the emitters, photosensitizers and semiconductors for organic optoelectronic devices, it is necessary to develop efficient and facile synthetic methods for benzo[c]thiophene derivatives.
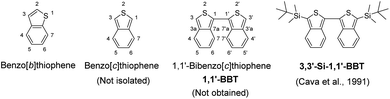 |
| Fig. 1 Chemical structures of benzo[b]thiophene, benzo[c]thiophene, 1,1′-bibenzo[c]thiophene 1,1′-BBT and 3,3′-silyl-disubstituted-1,1′-bibenzo[c]thiophene 3,3′-Si-1,1′-BBT. | |
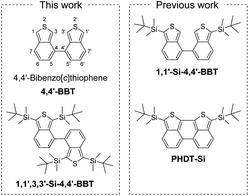 |
| Fig. 2 Chemical structures of 4,4′-bibenzo[c]thiophene 4,4′-BBT (BBT-1), 1,1′-silyl-disubstituted-4,4′-bibenzo[c]thiophene 1,1′-Si-4,4′-BBT (BBT-2), 1,1′,3,3′-silyl-tetrasubstituted-4,4′-bibenzo[c]thiophene 1,1′,3,3′-Si-4,4′-BBT (BBT-3) and fused-bibenzo[c]thiophene PHDT-Si. | |
Therefore, the aim of this work is to provide the synthetic strategy for 4,4′-bibenzo[c]thiophene derivatives and to reveal their optical and electrochemical properties. With this aim, we designed and synthesized unsubstituted 4,4′-bibenzo[c]thiophene (4,4′-BBT: abbr. as BBT-1) and its silyl-substituted derivatives 1,1′,3,3′-Si-4,4′-BBT (abbr. as BBT-3) with two sterically hindered tert-butyldimethylsilyl groups on each thiophene ring as well as 1,1′-Si-4,4′-BBT (abbr. as BBT-2) (Fig. 2). The characterization of BBT-1, BBT-2 and BBT-3 was successfully determined by FTIR, 1H and 13C NMR measurements, high-resolution mass spectrometry (HRMS) analysis, photoabsorption and fluorescence spectroscopy, cyclic voltammetry (CV) and density functional theory (DFT) calculations. This work is the first to achieve the synthesis, photophysical and electrochemical characteristics of unsubstituted 4,4′-bibenzo[c]thiophene BBT-1. Moreover, we achieved the single-crystal X-ray structural analysis of BBT-2 and BBT-3. Herein we report an efficient synthetic method for the 4,4′-bibenzo[c]thiophene derivatives and their photophysical properties in the solution and the solid state, electrochemical properties and X-ray crystal structures.
Results and discussion
Synthesis
4,4′-Bibenzo[c]thiophene (BBT-1) and its silyl-substituted derivatives (BBT-2 and BBT-3) were synthesized according to a stepwise synthetic protocol (Scheme 1). Indeed, unsubstituted 4,4′-bibenzo[c]thiophene (BBT-1) was successfully prepared by treatment of 1,1′,3,3′-tetrahydro-[4,4′-bibenzo[c]thiophene] 2,2′-dioxide 1 (see ref. 20 for the synthesis) with lithium hexamethyldisilazide (LHMDS). In our previous work, we demonstrated that BBT-2 with a tert-butyldimethylsilyl group on each thiophene ring was obtained by the reaction of 1 with tetramethylethylenediamine (TMEDA) and then nBuLi, followed by treatment with tert-butyldimethylsilyl chloride (TBDMSCl).20 In this work, we found that BBT-2 was also prepared by the reaction of BBT-1 with lithium diisopropylamide (LDA), followed by treatment with TBDMSCl. It is worth noting here that the above reactions from 1 or BBT-1 did not yield 3,3′-Si-4,4′-BBT with two tert-butyldimethylsilyl groups at the 3,3′-positions. This result indicates that the lithiation of BBT-1 preferentially occurs at the 1,1′-positions rather than the 3,3′-positions. On the other hand, BBT-3 was not obtained directly from 1 even under the condition using TMEDA, nBuLi (10 eq.) and then TBDMSCl (10 eq.). Finally, we prepared BBT-3 with two tert-butyldimethylsilyl groups on each thiophene ring by the reaction of BBT-1 or BBT-2 with LDA, followed by treatment with TBDMSCl. The characterization of BBT-1, BBT-2 and BBT-3 was successfully determined by FTIR, 1H and 13C NMR measurements and HRMS analysis. Therefore, this result proposes the stepwise synthetic method for the introduction of substituents into the thiophene rings of the 4,4′-bibenzo[c]thiophene skeleton.
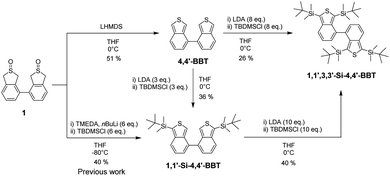 |
| Scheme 1 Synthetic route to 4,4′-bibenzo[c]thiophene derivatives 4,4′-BBT (BBT-1), 1,1′-Si-4,4′-BBT (BBT-2) and 1,1′,3,3′-Si-4,4′-BBT (BBT-3). | |
X-ray crystal structures
A single-crystal X-ray structural analysis was successfully made for BBT-2 and BBT-3 (Fig. 3), while unfortunately, we could not obtain single crystals of BBT-1 with sufficient size to make the X-ray structural analysis possible. The dihedral angles between the two benzo[c]thiophene units in BBT-2 and BBT-3 are 50.7° and 67.7° (Fig. 3a–d), respectively, which show that the two units in BBT-3 twist considerably due to the steric hindrance of the tert-butyldimethylsilyl groups at the 3,3′-positions, compared to those in BBT-2. The crystal structure of BBT-2 is made up of dimer units composed of pairs of molecules (Fig. 3e and g). There are two short interatomic contacts of less than 3.60 Å between a pair of molecules, that is, the interatomic distance between C(3) in a benzo[c]thiophene unit and C(5) in the other unit is ca. 3.58 Å. In the crystal structure of BBT-3, on the other hand, there are no short π–π contacts of less than 3.60 Å between the neighboring molecules (Fig. 3f and h), which indicates the absence of the π–π interactions between the molecules.
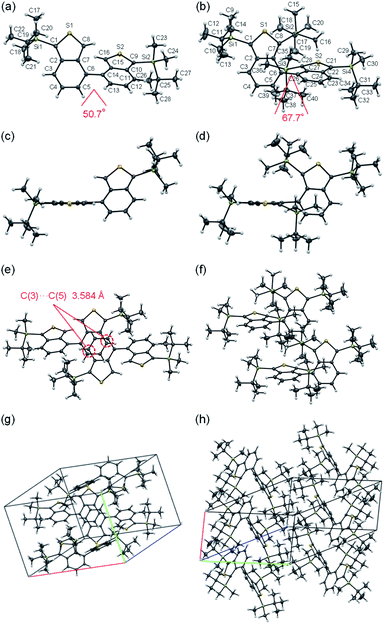 |
| Fig. 3 Crystal structures of 1,1′-Si-4,4′-BBT (BBT-2) and 1,1′,3,3′-Si-4,4′-BBT (BBT-3): (a) top view, (c) side view, (e) a top view of the pair of molecules and (g) molecular packing structure of BBT-2, and (b) top view, (d) side view, (f) a top view of the pair of molecules and (h) molecular packing structure of BBT-3. | |
Photophysical properties in the solution and the solid state
The photoabsorption and fluorescence spectra of BBT-1, BBT-2 and BBT-3 in toluene are shown in Fig. 4a, and their photophysical data are summarized in Table 1. The photoabsorption spectra demonstrated that BBT-2 and BBT-3 exhibit an intense photoabsorption band (λabsmax = 366 nm and 371 nm, respectively) with a relatively high εmax value (14
400 M−1 cm−1 and 21
300 M−1 cm−1, respectively) in a longer wavelength region by 7 nm and 12 nm, respectively, in comparison with that of BBT-1 (λabsmax = 359 nm, εmax = 7500 M−1 cm−1), due to the electron-donating tert-butyldimethylsilyl group. The corresponding fluorescent bands of the three fluorophores appear in longer wavelength regions in the order of BBT-1 (λflmax = 410 nm) < BBT-2 (λflmax = 420 nm) < BBT-3 (λflmax = 451 nm), and thus, the Stokes shift (SS) values increase in the order of BBT-1 (3465 cm−1) ≈ BBT-2 (3513 cm−1) < BBT-3 (4781 cm−1). The Φfl values of BBT-1, BBT-2 and BBT-3 are 0.41, 0.41 and 0.36, respectively, indicating moderate fluorescence properties. The relatively low Φfl and large SS values of BBT-3 indicate significant changes in the molecular and electronic structures between the ground and excited states by the rotation or twisting of the two benzo[c]thiophene units, due to the steric hindrance of the tert-butyldimethylsilyl groups at the 3,3′-positions. The time-resolved fluorescence spectroscopy of the three fluorophores demonstrated that the fluorescence lifetimes (τfl) are 3.46 ns for BBT-1, 3.19 ns for BBT-2 and 3.59 ns for BBT-3, and thus, there are little difference in the τfl values between the three fluorophores. The radiative rate constant (kr = 1.00 × 108 s−1) for BBT-3 is slightly smaller than those (1.18 × 108 s−1 and 1.29 × 108 s−1, respectively) for BBT-1 and BBT-2. In contrast, the nonradiative rate constants (knr = 1.70–1.84 × 108 s−1) of the three fluorophores resemble each other. Consequently, the ratio of nonradiative constant to radiative constant (knr/kr = 1.78) for BBT-3 is larger than those (1.44 and 1.43, respectively) for BBT-1 and BBT-2, suggesting that the lower Φfl value of BBT-3 is mainly due to the smaller kr value compared to those of BBT-1 and BBT-2.
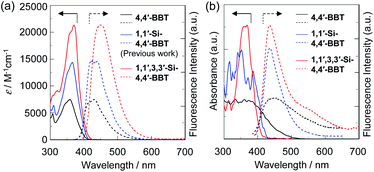 |
| Fig. 4 (a) Photoabsorption (solid line) and fluorescence (dotted line) spectra of 4,4′-BBT (BBT-1), 1,1′-Si-4,4′-BBT (BBT-2) and 1,1′,3,3′-Si-4,4′-BBT (BBT-3) in toluene. (b) Solid-state UV-vis diffuse reflection–absorption (solid line) and fluorescence (dotted line) spectra (λex = 360 nm) of the as-recrystallized BBT-1, BBT-2 and BBT-3. | |
Table 1 Photophysical and electrochemical data and HOMO and LUMO energy levels of 4,4′-bibenzo[c]thiophene derivatives in the solution
Dye |
λabsmaxa/nm (εmax/M−1 cm−1) |
λflmaxb/nm (Φfl) |
τflc/ns |
krd/s−1 |
knre/s−1 |
knr/kr |
Eoxonsetf/V |
Eoptgg/eV |
HOMOh/eV |
LUMOh/eV |
In toluene. In toluene. Fluorescence quantum yields (Φfl) were determined by using a calibrated integrating sphere system (λex = 359 nm, 366 nm and 371 nm for 4,4′-BBT (BBT-1), 1,1′-Si-4,4′-BBT (BBT-2) and 1,1′,3,3′-Si-4,4′-BBT (BBT-3), respectively). Fluorescence lifetime. Radiative rate constant (kr = Φfl/τfl). Nonradiative rate constant (knr = (1 − Φfl)/τfl). Onset (Eoxonset) versus Fc/Fc+ of the oxidation potential. Optical energy gaps (Eoptg) were determined from the intersection (393 nm, 399 nm and 408 nm for BBT-1, BBT-2 and BBT-3, respectively) of photoabsorption and fluorescence spectra in toluene. Versus vacuum level. Previous work (ref. 20). |
4,4′-BBT |
359 (7500) |
410 (0.41) |
3.46 |
1.18 × 108 |
1.70 × 108 |
1.44 |
0.75 |
3.16 |
−5.55 |
−2.39 |
1,1′-Si-4,4′-BBT |
366 (14 400)i |
420 (0.41)i |
3.19i |
1.29 × 108i |
1.84 × 108i |
1.43i |
0.65i |
3.11i |
−5.45i |
−2.34i |
1,1′,3,3′-Si-4,4′-BBT |
371 (21 300) |
451 (0.36) |
3.59 |
1.00 × 108 |
1.78 × 108 |
1.78 |
0.54 |
3.04 |
−5.34 |
−2.30 |
In order to investigate the solid-state photophysical properties of BBT-1, BBT-2 and BBT-3, we have measured the solid-state UV-Vis diffuse reflection–photoabsorption and fluorescence spectra for the solids (Fig. 4b), and their photophysical data are summarized in Table 2. Both BBT-2 and BBT-3 in the solid state show a photoabsorption band at around 360 nm with an onset at 420–425 nm, which is similar to the corresponding photoabsorption band of the two fluorophores in toluene (Fig. 4a). On the other hand, the photoabsorption band of BBT-1 in the solid state is broadened in a longer wavelength region with an onset of ca. 500 nm, in comparison with that in toluene. The corresponding solid-state fluorescence spectra revealed that BBT-1 and BBT-2 show a fluorescence band (λfl-solidmax = 455 nm and 435 nm, respectively) in a longer wavelength region by 45 nm and 15 nm, respectively, compared to those in toluene. It is worth mentioning here that the fluorescence band (λfl-solidmax = 435 nm) of BBT-3 in the solid state appeared in a shorter wavelength region than that (λflmax = 451 nm) in toluene (Fig. 4a). The Φfl-solid values of BBT-1, BBT-2 and BBT-3 in the solid state are <0.02, 0.22 and 0.25, respectively, which are lower than those in toluene (Table 1). In particular, the fluorescence properties of BBT-1 were strongly quenched in the solid state. The bathochromic shifts of λabsmax and λflmax and the lowering of Φfl value by changing from the solution to the solid state are quite common and explained in terms of the formation of intermolecular π–π interactions between the fluorophores in the solid state and consequent delocalization of excitons or excimers.21 Thus, for BBT-1, the bathochromic shift of λflmax and the significant lowering of Φfl value by changing from the solution to the solid state would be attributed to the formation of intermolecular π–π interactions between the fluorophores in the solid state. On the other hand, the relatively high Φfl-solid value of BBT-3 in the solid state is based on the fact that the short π–π contacts of less than 3.60 Å between the neighboring molecules were not observed in the crystal structure of BBT-3 (Fig. 3f), which indicates the absence of the intermolecular π–π interactions between the fluorophores. Indeed, the four tert-butyldimethylsilyl groups at the 1,1′,3,3′-positions can effectively prevent the fluorophores from forming intermolecular π–π interactions in the solid state. In addition, for BBT-3, the hypsochromic shift of λflmax by changing from the solution to the solid state may be attributed to inhibition of structural relaxation in the excited state by restriction of the rotation or twisting of the two benzo[c]thiophenes in the solid state, due to the steric hindrance of the tert-butyldimethylsilyl groups at the 3,3′-positions between the neighboring fluorophores. The time-resolved fluorescence spectroscopy of the three 4,4′-bibenzo[c]thiophene derivatives in the solid state demonstrated that the τfl-solid values are 1.61 ns for BBT-2 and 2.33 ns for BBT-3, which are shorter than those (3.19 ns and 3.59 ns, respectively) in toluene. However, the precise evaluation of the τfl-solid value (<1 ns) of BBT-1 was difficult due to its feeble solid-state fluorescence properties. The kr-solid values for BBT-2 and BBT-3 in the solid state are 1.38 × 108 s−1 and 9.00 × 107 s−1, respectively, which are almost equivalent to those (1.29 × 108 s−1 and 1.00 × 108 s−1, respectively) in toluene. In contrast, the knr-solid values (4.84 × 108 s−1 and 3.38 × 108 s−1, respectively) for BBT-2 and BBT-3 in the solid state are larger than those (1.84 × 108 s−1 and 1.78 × 108 s−1, respectively) in toluene. The knr-solid/kr-solid values for BBT-2 and BBT-3 in the solid state are 3.55 and 3.76, respectively, which are larger than those (1.43 and 1.78, respectively) in toluene, suggesting that the non-radiative decay in the solid state is accelerated. Consequently, the relatively low Φfl-solid values of BBT-2 and BBT-3 in the solid state are mainly due to the larger knr-solid values compared to those in toluene.
Table 2 Photophysical data of 4,4′-bibenzo[c]thiophene derivatives in the solid state
Dye |
λabs-solidmax/nm |
λfl-solidmaxa/nm (Φfl-solid) |
τfl-solidb/ns |
kr-solidc/s−1 |
knr-solidd/s−1 |
knr-solid/kr-solid |
Fluorescence quantum yields (Φfl-solid) were determined by using a calibrated integrating sphere system (λex = 360 nm for 4,4′-BBT (BBT-1), 1,1′-Si-4,4′-BBT (BBT-2) and 1,1′,3,3′-Si-4,4′-BBT (BBT-3)). Fluorescence lifetime. Radiative rate constant (kr-solid = Φfl-solid/τfl-solid). Nonradiative rate constant (knr-solid = (1 − Φfl-solid)/τfl-solid). Due to feeble solid-state fluorescence properties. |
4,4′-BBT (BBT-1) |
360 |
455 (<0.02) |
<1 |
—e |
—e |
—e |
1,1′-Si-4,4′-BBT (BBT-2) |
360 |
435 (0.22) |
1.61 |
1.38 × 108 |
4.84 × 108 |
3.55 |
1,1′,3,3′-Si-4,4′-BBT (BBT-3) |
370 |
435 (0.25) |
2.33 |
9.00 × 107 |
3.38 × 108 |
3.76 |
Electrochemical properties
The electrochemical properties of BBT-1, BBT-2 and BBT-3 were determined using CV in acetonitrile containing 0.1 M tetrabutylammonium perchlorate (Bu4NClO4). The potentials were internally referenced to ferrocene/ferrocenium (Fc/Fc+). The cyclic voltammograms of the three compounds are shown in Fig. 5, and their electrochemical data and the HOMO and LUMO energy levels are summarized in Table 1. For all the three compounds, an irreversible oxidation wave was observed at 0.88 V for BBT-1, 0.78 V for BBT-2 and 0.67 V for BBT-3, while any obvious reduction wave did not appear within the potential window. The oxidation waves for BBT-2 and BBT-3 are cathodically shifted by 0.10 V and 0.21 V, respectively, compared to that for BBT-1, indicating that the introduction of the tert-butyldimethylsilyl group into the benzo[c]thiophene skeleton can lower the oxidation potential. The HOMO energy levels (−[Eoxonset + 4.8] eV) versus vacuum level were estimated from the onset potentials (Eoxonset = 0.75 V for BBT-1, 0.65 V for BBT-2 and 0.54 V for BBT-3) of the oxidation waves, and the LUMO energy levels were estimated from the Eoxonset and intersections (optical energy gap: Eoptg = 3.16 eV for BBT-1, 3.11 eV for BBT-2 and 3.04 eV for BBT-3) of the photoabsorption and fluorescence spectra in toluene. The HOMO energy level rises in the order of BBT-1 (−5.55 eV) < BBT-2 (−5.45 eV) < BBT-3 (−5.34 eV). Similarly, the LUMO energy level rises in the order of BBT-1 (−2.39 eV) < BBT-2 (−2.34 eV) < BBT-3 (−2.30 eV). However, from BBT-1 to BBT-3, the rise of the HOMO energy level is larger than that of the LUMO energy level. Consequently, the fact reveals that the bathochromic shift of the photoabsorption band from BBT-1 to BBT-2 and BBT-3 is mainly attributed to the destabilization of the HOMO energy level through the introduction of the electron-donating tert-butyldimethylsilyl group into the benzo[c]thiophene skeleton, resulting in a decrease in the HOMO–LUMO band gap.
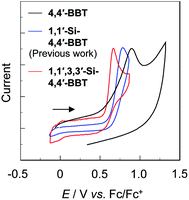 |
| Fig. 5 Cyclic voltammograms of 4,4′-BBT (BBT-1), 1,1′-Si-4,4′-BBT (BBT-2) and 1,1′,3,3′-Si-4,4′-BBT (BBT-3) in acetonitrile containing 0.1 M Bu4NClO4 at a scan rate of 100 mV s−1. The arrow denotes the direction of the potential scan. | |
Theoretical calculations
In order to examine the electronic structures of the 4,4′-bibenzo[c]thiophene derivatives, the molecular structures and molecular orbitals of BBT-1, BBT-2 and BBT-3 and benzo[c]thiophene (BT) as a reference were calculated using DFT at the B3LYP/6-31G(d,p) level22 (Fig. 6). The DFT calculations demonstrate that the calculated dihedral angles between the two benzo[c]thiophene units are 57.8° for BBT-1, 59.2° for BBT-2 and 96.8° for BBT-3, that is, the two units in BBT-3 twist considerably due to the tert-butyldimethylsilyl groups at the 3,3′-positions, compared to those in BBT-1 and BBT-2 (Fig. 6a). Therefore, good correlation was observed between the molecular structures estimated by the DFT calculations and experimentally obtained from the X-ray crystal structure analysis, although for BBT-3, the calculated dihedral angle between the two benzo[c]thiophene units is larger than that determined from the X-ray crystal structure analysis (Fig. 3a and b). As shown in Fig. 6b, for the three 4,4′-bibenzo[c]thiophene derivatives the HOMO are delocalized on each benzo[c]thiophene unit, as with the pattern of BT. However, the LUMO for the three 4,4′-bibenzo[c]thiophene derivatives are delocalized over the whole molecule through the 4,4′-positions. It was found that the HOMO energy levels of the three 4,4′-bibenzo[c]thiophene derivatives are higher than that (−5.38 eV) of BT, but their LUMO energy levels are lower than that (−1.38 eV) of BT. The HOMO and LUMO energy levels rise in the order of BBT-1 (−5.29 eV and −1.55 eV) < BBT-2 (−5.22 eV and −1.53 eV) < BBT-3 (−5.07 eV and −1.52 eV), while from BBT-1 to BBT-3, the rise of the HOMO energy level is larger than that of the LUMO energy level, resulting in a decrease in the HOMO–LUMO band gap. Moreover, the time-dependent density functional theory (TD-DFT) calculations indicate that the calculated λabs-calcdmax of BBT-1, BBT-2 and BBT-3 is 345 nm, 354 nm and 356 nm, respectively, which appears in a significantly longer wavelength region than that (332 nm) of BT (Fig. 7). For BT and the three 4,4′-bibenzo[c]thiophene derivative, the S0 → S1 transitions are mainly attributed to the transitions from the HOMO to the LUMO (70% for BT, 67% for BBT-1, 67% for BBT-2 and 68% for BBT-3). The corresponding oscillator strength (f) value increases in the order of BT (0.07) < BBT-1 (0.11) < BBT-3 (0.14) < BBT-2 (0.16), and indeed, the calculated εcalcd value also increases in the order of BT (3000 M−1 cm−1) < BBT-1 (7800 M−1 cm−1) < BBT-3 (13
200 M−1 cm−1) < BBT-2 (15
600 M−1 cm−1). Thus, the DFT calculations reveal that the bathochromic shift of the photoabsorption band from BBT-1 to BBT-2 and BBT-3 is ascribable to the destabilization of the HOMO energy level through the introduction of the electron-donating tert-butyldimethylsilyl group into the benzo[c]thiophene skeleton. Consequently, the DFT calculations are in good agreement with the experimental results from the CV and the photoabsorption and fluorescence spectral analyses, although the f and εcalcd values of BBT-3 are slightly lower than those of BBT-2, which is opposite in the values to the experimental results.
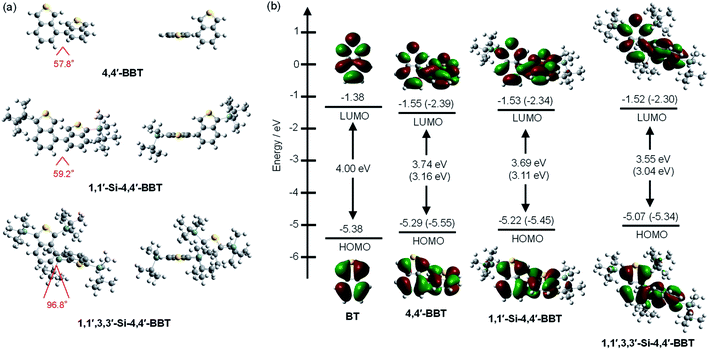 |
| Fig. 6 (a) Optimized geometries (top and side views) of 4,4′-BBT (BBT-1), 1,1′-Si-4,4′-BBT (BBT-2) and 1,1′,3,3′-Si-4,4′-BBT (BBT-3) and (b) energy level diagram, HOMO and LUMO of BT and the three 4,4′-bibenzo[c]thiophene derivatives derived from DFT calculations at the B3LYP/6-31G(d,p) level. Numbers in parentheses are the experimental values. | |
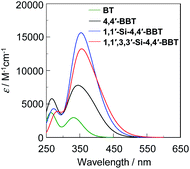 |
| Fig. 7 Photoabsorption spectra of BT, 4,4′-BBT (BBT-1), 1,1′-Si-4,4′-BBT (BBT-2) and 1,1′,3,3′-Si-4,4′-BBT (BBT-3) derived from TD-DFT calculations. | |
Conclusions
We have achieved a facile synthesis of 4,4′-bibenzo[c]thiophene derivatives, unsubstituted 4,4′-bibenzo[c]thiophene 4,4′-BBT and its silyl-substituted derivatives 1,1′-Si-4,4′-BBT and 1,1′,3,3′-Si-4,4′-BBT with one or two tert-butyldimethylsilyl groups on each thiophene ring, and revealed their photophysical properties in the solution and in the solid state and electrochemical properties. It was found that the bathochromic shift of the photoabsorption band from 4,4′-BBT to 1,1′-Si-4,4′-BBT and 1,1′,3,3′-Si-4,4′-BBT is mainly attributed to the destabilization of the HOMO energy level through the introduction of the electron-donating tert-butyldimethylsilyl group into the benzo[c]thiophene skeleton, resulting in a decrease in the HOMO–LUMO band gap. The three 4,4′-bibenzo[c]thiophene derivatives exhibit moderate fluorescence properties in the solution (Φfl = ca. 0.4). Moreover, the silyl-substituted derivatives 1,1′-Si-4,4′-BBT and 1,1′,3,3′-Si-4,4′-BBT show relatively high Φfl-solid value (0.22 and 0.25, respectively) in the solid state, compared to 4,4′-BBT (Φfl-solid < 0.02). The DFT calculations demonstrate that the HOMO energy levels of the 4,4′-bibenzo[c]thiophene derivatives are higher than that of benzo[c]thiophene (BT), while their LUMO energy levels are lower than that of BT. Consequently, this work opened up a new way for not only synthetic method and photophysical and electrochemical properties of 4,4′-bibenzo[c]thiophene derivatives with the different substituents on the thiophene rings, but also development of fused-bibenzo[c]thiophene derivatives as functional dyes.
Experimental
General
Melting points were measured with an AS ONE ATM-02. IR spectra were recorded on a SHIMADZU IRTracer-100 by ATR method. 1H NMR and 13C NMR spectra were recorded on a Varian-500 FT NMR spectrometer and NMR solvent was used as an external standard for calibration. High-resolution mass spectral data by APCI and GC-EI were acquired on a Thermo Fisher Scientific LTQ Orbitrap XL and JEOL JMS-T100 GCV 4G, respectively. Recycling gel permeation chromatography (GPC) were preformed using RI-detector (GL Science RI 704) and pump (GILSON 307 PUMP) with column (Shodex GPC H-2001L). Photoabsorption spectra of solution were observed with a Shimadzu UV-3600 plus spectrophotometer. Photoabsorption spectra of the solid were recorded by a Shimadzu UV-3600 plus spectrophotometer with a calibrated integrating sphere system. Fluorescence spectra of solution and the solid were measured with a HORIBA FluoroMax-4 spectrofluorometer. The fluorescence quantum yields in solution and in the solid state were determined using a HORIBA FluoroMax-4 spectrofluorometer with a calibrated integrating sphere system. Fluorescence decay measurements were performed on a HORIBA DeltaFlex modular fluorescence lifetime system, using a Nano LED pulsed diode excitation source (370 nm). Cyclic voltammetry (CV) curves were recorded in acetonitrile/Bu4NClO4 (0.1 M) solution at a scan rate of 100 mV s−1 with a three-electrode system consisting of Ag/Ag+ as the reference electrode, a Pt plate as the working electrode and a Pt wire as the counter electrode using an Electrochemical Measurement System HZ-7000 (HOKUTO DENKO).
Synthesis
4,4′-Bibenzo[c]thiophene (4,4′-BBT). To a THF solution (20 mL) of 1,1′,3,3′-tetrahydro-[4,4′-bibenzo[c]thiophene] 2,2′-dioxide (1)20 (0.20 g, 0.66 mmol) under a nitrogen atmosphere at 0 °C was added dropwise a 1.3 M THF solution of lithium hexamethyldisilazide (3.1 mL, 4.0 mmol). After stirring for 3 h, the reaction mixture was quenched with water, and then, the solution was extracted with ethyl acetate. The ethyl acetate extract was dried over anhydrous MgSO4, filtrated and concentrated. Recycling GPC (toluene as eluent) was performed to give 4,4′-BBT (0.09 g, yield 51%) as a light-yellow solid; decomposed at around 145 °C; FT-IR (ATR):
= 3103, 1686, 1172, 864 cm−1; 1H NMR (500 MHz, acetone-d6): δ = 7.19–7.24 (m, 4H), 7.59 (dd, J = 1.1 and 3.4 Hz, 2H), 7.75 (dt, 2H), 8.00 (d, J = 3.4 Hz, 2H) ppm; 13C NMR (125 MHz, acetone-d6): δ = 117.96, 118.31, 122.51, 124.29, 124.35, 134.56, 138.45, 139.91 ppm; HRMS (GC-EI): m/z (%): [M − H+] calcd for C16H9S2, 265.01457; found 265.01442.
1,1′-Bis(tert-butyldimethylsilyl)-4,4′-bibenzo[c]thiophene (1,1′-Si-4,4′-BBT). (Method A: previous work)20 To a THF solution (60 mL) of 1 (0.50 g, 1.65 mmol) under an argon atmosphere at −80 °C was added tetramethylethylenediamine (1.48 g, 9.92 mmol). After stirring for 30 min, a 1.6 M hexane solution of nBuLi (6.20 mL, 9.92 mmol) was added dropwise for 30 min, and then, a THF solution (10 mL) of tert-butyldimethylsilyl chloride (0.75 g, 4.96 mmol) was added dropwise for 20 min. After stirring for 12 h at room temperature, the reaction mixture was quenched with water, and then, the solution was extracted with ethyl acetate. The ethyl acetate extract was dried over anhydrous MgSO4, filtrated and concentrated. The residue was chromatographed on silica gel (hexane as eluent), and then, recycling GPC (toluene as eluent) was performed to give 1,1′-Si-4,4′-BBT (0.33 g, yield 40%) as a light-yellow solid. (Method B) To a THF solution (0.8 mL) of 4,4′-BBT (0.14 g, 0.53 mmol) under a nitrogen atmosphere at 0 °C was added dropwise a 1.0 M hexane/THF solution of lithium diisopropylamide (1.6 mL, 1.6 mmol). After stirring for 3 h, a THF solution (0.3 mL) of tert-butyldimethylsilyl chloride (0.24 g, 1.6 mmol) was added dropwise. The reaction mixture was further stirred for 12 h. The reaction mixture was quenched with water, and then, the solution was extracted with ethyl acetate. The ethyl acetate extract was dried over anhydrous MgSO4, filtrated and concentrated. The residue was chromatographed on silica gel (dichloromethane
:
hexane = 1
:
1 as eluent) and then on alumina (dichloromethane
:
hexane = 1
:
3 as eluent) to give 1,1′-Si-4,4′-BBT (0.095 g, yield 36%) as a yellow solid; mp 153–155 °C; FT-IR (ATR):
= 2949 (aliphatic C–H str.), 2926 (aliphatic C–H str.), 2855 (aliphatic C–H str.), 1458 (Si–C(Ar) str.), 1360, 1250 (aliphatic Si–CH3 str.), 804 (aliphatic Si–CH3 str.) cm−1; 1H NMR (500 MHz, CDCl3): δ = 0.53 (s, 12H), 0.99 (s, 18H), 7.18–7.23 (m, 4H), 7.78 (d, J = 8.1 Hz, 2H), 7.81 (s, 2H) ppm; 13C NMR (125 MHz, CDCl3): δ = −3.73, 18.37, 26.92, 123.17, 123.25, 123.50, 124.03, 128.34, 134.88, 140.20, 145.12 ppm; HRMS (APCI): m/z (%): [M + H+] calcd for C28H39S2Si2, 495.20262; found 495.20303.
1,1′,3,3′-Tetrakis(tert-butyldimethylsilyl)-4,4′-bibenzo[c]thiophene (1,1′,3,3′-Si-4,4′-BBT). (Method A) To a THF solution (0.8 mL) of 4,4′-BBT (0.14 g, 0.53 mmol) under a nitrogen atmosphere at 0 °C was added dropwise a 1.0 M hexane/THF solution of lithium diisopropylamide (4.2 mL, 4.2 mmol). After stirring for 3 h, a THF solution (1.0 mL) of tert-butyldimethylsilyl chloride (0.62 g, 4.2 mmol) was added dropwise. The reaction mixture was further stirred for 12 h. The reaction mixture was quenched with water, and then, the solution was extracted with ethyl acetate. The ethyl acetate extract was dried over anhydrous MgSO4, filtrated and concentrated. The residue was chromatographed on silica gel (dichloromethane
:
hexane = 1
:
3 as eluent) to give 1,1′,3,3′-Si-4,4′-BBT (0.1 g, yield 26%) as a white solid. (Method B) To a THF solution (0.3 mL) of 1,1′-Si-4,4′-BBT (0.07 g, 0.14 mmol) under a nitrogen atmosphere at 0 °C was added dropwise a 1.0 M hexane/THF solution of lithium diisopropylamide (1.4 mL, 1.4 mmol). After stirring for 3 h, a THF solution (0.3 mL) of tert-butyldimethylsilyl chloride (0.21 g, 1.4 mmol) was added dropwise. The reaction mixture was further stirred for 14 h. The reaction mixture was quenched with water, and then, the solution was extracted with dichloromethane. The dichloromethane extract was dried over anhydrous MgSO4, filtrated and concentrated. The residue was chromatographed on silica gel (hexane as eluent) to give 1,1′,3,3′-Si-4,4′-BBT (0.04 g, yield 40%) as a white solid; mp 172–173 °C; FT-IR (ATR):
= 2955 (aliphatic C–H str.), 2926 (aliphatic C–H str.), 2855 (aliphatic C–H str.), 1470 (Si–C(Ar) str.), 1362, 1250 (aliphatic Si–CH3 str.), 804 (aliphatic Si–CH3 str.) cm−1; 1H NMR (500 MHz, acetone-d6): δ = −0.87 (s, 3H), −0.04 (s, 3H), 0.57 (s, 3H), 0.62 (s, 3H), 0.77 (s, 9H), 0.99 (s, 9H), 7.02 (dd, J = 1.2 and 6.6 Hz, 2H), 7.19–7.22 (m, 2H), 7.99 (dd, J = 8.8 Hz, 2H) ppm; 13C NMR (125 MHz, acetone-d6): δ = −3.78, −3.61, 18.97, 19.32, 27.15, 28.25, 123.12, 125.27, 128.83, 136.01, 136.12, 139.28, 147.78, 149.76 ppm; HRMS (APCI): m/z (%): [M+•] calcd for C40H66S2Si4, 722.36775; found 722.36843.
X-ray crystallographic analysis
The reflection data were collected at 100 K on a Bruker AXS SMART APEX II ULTRA diffractometer using monochromated Mo-Kα (λ = 0.71073 Å). The structure was solved by the SHELXT 2014/5 method and refined based on full-matrix least squares on F2 using SHELXL-2017/1. The non-hydrogen atoms were refined anisotropically. Hydrogen atoms were fixed geometrically and not refined. Crystallographic data have been deposited in the Cambridge Crystallographic Data Centre (CCDC 2055734† for 1,1′-Si-4,4′-BBT and CCDC 2055735† for 1,1′,3,3′-Si-4,4′-BBT, respectively).
Crystal of 1,1′-Si-4,4′-BBT. A suitable single crystal of 1,1′-Si-4,4′-BBT was grown by slow evaporation of acetone/ethanol solution at room temperature for several days, as colorless block crystal, air stable. Crystallographic data: C28H38S2Si2, M = 494.88, monoclinic, a = 17.6427(9), b = 11.2395(6), c = 14.1794(7) Å, β = 95.121(1)°, V = 2800.5(2) Å3, Dcalcd = 1.174 g cm−3, space group P21/c (no. 14), Z = 4, 17
974 reflections measured, 6739 unique (Rint = 0.027), which were used in all calculations. The final R1(reflections) = 0.0292(5949) [I > 2σ(I)], wR2(reflections) = 0.0743(6739). GOF = 0.955 (Table S1†).
Crystal of 1,1′,3,3′-Si-4,4′-BBT. A suitable single crystal of 1,1′,3,3′-Si-4,4′-BBT was grown by slow evaporation of acetone/ethanol solution at room temperature for several days, as colorless plate crystal, air stable. Crystallographic data: C40H66S2Si4, M = 723.40, triclinic, a = 7.5572(9), b = 13.6372(16), c = 22.295(3) Å, α = 72.5230(10)°, β = 89.9300(10)°, γ = 81.0900(10)°, V = 2162.7(4) Å3, Dcalcd = 1.111 g cm−3, space group P
(no. 2), Z = 2, 10
428 reflections measured, 10
234 unique (Rint = 0.041), which were used in all calculations. The final R1(reflections) = 0.0449(7519) [I > 2σ(I)], wR2(reflections) = 0.1092(10
234). GOF = 1.011 (Table S1†).
Conflicts of interest
There are no conflicts to declare.
Acknowledgements
This work was supported by Grants-in-Aid for Scientific Research (B) from the Japan Society for the Promotion of Science (JSPS) KAKENHI Grant Number 19H02754.
Notes and references
- M. E. Cinar and T. Ozturk, Thienothiophenes, Dithienothiophenes, and Thienoacenes: Syntheses, Oligomers, Polymers, and Properties, Chem. Rev., 2015, 115, 3036–3140 CrossRef CAS PubMed
. - J. Y. Kim, D. Yokoyama and C. Adachi, Horizontal Orientation of Disk-like Hole Transport Molecules and Their Application for Organic Light-Emitting Diodes Requiring a Lower Driving Voltage, J. Phys. Chem. C, 2012, 116, 8699–8706 CrossRef CAS
. -
(a) H. Usta, D. Kim, R. Ozdemir, Y. Zorlu, S. Kim, M. C. R. Delgado, A. Harbuzaru, S. Kim, G. Demirel, J. Hong, Y.-G. Ha, K. Cho, A. Facchetti and M.-G. Kim, High Electron Mobility in [1]Benzothieno[3,2-b][1]benzothiophene-Based Field-Effect Transistors: Toward n-Type BTBTs, Chem. Mater., 2019, 31, 5254–5263 CrossRef CAS
;
(b) J. Li, L. Zheng, L. Sun, C. Li, X. Zhang, S. Cheng and W. Hu, New anthracene derivatives integrating high mobility and strong emission, J. Mater. Chem. C, 2018, 6, 13257–13260 RSC
. -
(a) Y.-J. Cheng, S.-H. Yang and C.-S. Hsu, Synthesis of Conjugated Polymers for Organic Solar Cell Applications, Chem. Rev., 2009, 109, 5868–5923 CrossRef CAS PubMed
;
(b) H. Tan, H. Tan, X. Zheng, J. Yang, J. Yu and W. Zhu, Significant influence of the benzothiophene ring substitution position on the photovoltaic performance of benzodithiophene-based donor polymers, J. Mater. Chem. C, 2020, 8, 3183–3191 RSC
;
(c) J. Kim, H. Min Ko, N. Cho, S. Paek, J. K. Lee and J. Ko, Efficient small molecule organic semiconductor containing bis-dimethylfluorenyl amino benzo[b]thiophene for high open circuit voltage in high efficiency solution processed organic solar cell, RSC Adv., 2012, 2, 2692–2695 RSC
. -
(a) M. Liang and J. Chen, Arylamine organic dyes for dye-sensitized solar cells, Chem. Soc. Rev., 2013, 42, 3453–3488 RSC
;
(b) H. Choi, J. K. Lee, K. Song, S. O. Kang and J. Ko, Novel organic dyes containing bis-dimethylfluorenyl amino benzo[b]thiophene for highly efficient dye-sensitized solar cell, Tetrahedron, 2007, 63, 3115–3121 CrossRef CAS
. -
(a) K. Takimiya, I. Osaka, T. Mori and M. Nakano, Organic Semiconductors Based on [1]Benzothieno[3,2-b][1]benzothiophene Substructure, Acc. Chem. Res., 2014, 47, 1493–1502 CrossRef CAS PubMed
;
(b) M. Nakano, I. Osaka, D. Hashizume and K. Takimiya, α-Modified Naphthodithiophene Diimides-Molecular Design Strategy for Air-Stable n-Channel Organic Semiconductors, Chem. Mater., 2015, 27, 6418–6425 CrossRef CAS
. -
(a) F. Wudl, M. Kobayashi and A. J. Heeger, Poly(isothianaphthene), J. Org. Chem., 1984, 49, 3382–3384 CrossRef CAS
;
(b) K. Kobayashi, N. Colaneri, M. Boysel, F. Wudl and A. J. Heeger, The electronic and electrochemical properties of poly(isothianaphthene), J. Chem. Phys., 1985, 82, 5717–5723 CrossRef
;
(c) R. Lazzaroni, J. Riga, J. Verbist, J. L. Brédas and F. Wudl, J. Chem. Phys., 1988, 88, 4257–4262 CrossRef CAS
;
(d) H. Meng and F. Wudl, A Robust Low Band Gap Processable n-Type Conducting Polymer Based on Poly(isothianaphthene), Macromolecules, 2001, 34, 1810–1816 CrossRef CAS
. -
(a) S. T. Meek, E. E. Nesterov and T. M. Swager, Near-Infrared Fluorophores Containing Benzo[c]heterocycle Subunits, Org. Lett., 2008, 10, 2991–2993 CrossRef CAS PubMed
;
(b) A. K. Mohanakrishnan and P. Amaladass, Synthesis of 1,3-diaryl benzo[c]thiophenes, Tetrahedron Lett., 2005, 46, 4225–4229 CrossRef CAS
;
(c) K. Kawabata and H. Goto, Synthesis and optical properties of 1,1-binaphthyl–thiophene alternating
copolymers with main chain chirality, J. Mater. Chem., 2012, 22, 23514–23524 RSC
;
(d) J. A. Clement, P. Gunasekaran and A. K. Mohanakrishnan, Synthesis and characterization of benzo[c]thiophene analogs incorporating benzo[b]thiophene/1-hexylindole/benzo[b]furan, Tetrahedron, 2009, 65, 4113–4123 CrossRef
;
(e) S. Ogawa, H. Muraoka, K. Kikuta, F. Saito and R. Sato, Design of reversible multi-electron redox systems using benzochalcogenophenes containing aryl and/or ferrocenyl fragments, J. Organomet. Chem., 2007, 692, 60–69 CrossRef CAS
. -
(a) G. G. Rajeshwaran, M. Nandakumar, R. Sureshbabu and A. K Mohanakrishnan, Lewis Acid-Mediated Michaelis–Arbuzov Reaction at Room Temperature: A Facile Preparation of Arylmethyl/Heteroarylmethyl Phosphonates, Org. Lett., 2011, 13, 1270–1273 CrossRef CAS PubMed
;
(b) Y. Qin, J. Y. Kim, C. D. Frisbie and M. A. Hillmyer, Distannylated Isothianaphthene: A Versatile Building Block for Low Bandgap Conjugated Polymers, Macromolecules, 2008, 41, 5563–5570 CrossRef CAS
;
(c) R. A. Kumar, M. R. Pattanayak, E. Yen-Pon, J. Eliyan, K. Porte, S. Bernard, M. Riomet, P. Thuéry, D. Audisio and F. Taran, Strain-Promoted 1,3-Dithiolium-4-olates–Alkyne Cycloaddition, Angew. Chem., Int. Ed., 2019, 58, 14544–14548 CrossRef CAS PubMed
;
(d) M. R. Raj and S. Anandan, Donor conjugated polymers-based on alkyl chain substituted oligobenzo[c]thiophene derivatives with well-balanced energy levels for bulk heterojunction solar cells, RSC Adv., 2013, 3, 14595–14608 RSC
;
(e) T. J. L. Silva, P. J. Mendes, M. H. Garcia, M. P. Robalo, J. P. P. Ramalho, A. J. P. Carvalho, M. Büchert, C. Wittenburg and J. Heck, Benzo[c]thiophene Chromophores Linked to Cationic Fe and Ru Derivatives for NLO Materials: Synthesis Characterization and Quadratic Hyperpolarizabilities, Eur. J. Inorg. Chem., 2013, 3506–3517 CrossRef CAS
. -
(a) N. S. Kumar and A. K. Mohanakrishnan, Synthesis and characterization of fluorene tethered benzo[c]thiophene/benzo[c]selenophene analogs, Tetrahedron, 2010, 66, 5660–5670 CrossRef CAS
;
(b) R. Sivasakthikumaran, J. A. Clement, M. Nandakumar and A. K. Mohanakrishnan, Synthesis and Characterization of Thienothiophene Conjugated Benzo[c]Thiophene Analogs, J. Heterocycl. Chem., 2016, 53, 1461–1468 CrossRef CAS
;
(c) R. D. A. Hudson, I. Asselberghs, K. Clays, L. P. Cuffe, J. F. Gallagher, A. R. Manning, A. Persoons and K. Wostyn, The linear and nonlinear optical properties of organometallic chromophores derived from ferrocene, [Fe2(η5-C5H5)2(CO)2(μ-CO)(μ-C–CH3)]+[BF4]− and terthienyl spacers. Crystal structure of 2-[(E)-2-ferrocenylethenyl]-5-(2-thienyl)thiophene, J. Organomet. Chem., 2001, 637–639, 435–444 CrossRef CAS
;
(d) W. Debrouwer, R. A. J. Seigneur, T. S. A. Heugebaert and C. V. Stevens, Gold superacid-catalyzed preparation of benzo[c]thiophenes, Chem. Commun., 2015, 51, 729–732 RSC
;
(e) R. Sato, E. Takeda, S. Nakajo, T. Kimura, S. Ogawa and Y. Kawai, Oxidation of 1,4-dihydro-1,4-diphenyl-2,3-benzodithiin, Heteroat. Chem., 2001, 12, 209–216 CrossRef CAS
;
(f) R. Kiebooms, I. Hoogmartens, P. Adriaensens, D. Vanderzande and J. Gelan, Low-Band-Gap Conjugated Polymers. Improved Model Compounds for the Structural Analysis of Poly(isothianaphthene), Macromolecules, 1995, 28, 4961–4969 CrossRef CAS
. -
(a) U. Mitschke and P. Bäuerle, Synthesis, characterization, and electrogenerated chemiluminescence of phenyl-substituted, phenyl-annulated, and spirofluorenyl-bridged oligothiophenes, J. Chem. Soc., Perkin Trans. 1, 2001, 740–753 RSC
;
(b) R. Kisselev and M. Thelakkat, Synthesis and Characterization of Poly(triarylamine)s Containing Isothianaphthene Moieties, Macromolecules, 2004, 37, 8951–8958 CrossRef CAS
;
(c) T. V. Hughes and M. P. Cava, Electrophilic Cyanations Using 1-Cyanobenzotriazole:
sp2 and sp Carbanions, J. Org. Chem., 1999, 64, 313–315 CrossRef CAS PubMed
;
(d) V. Dhayalan, J. A. Clement, R. Jagan and A. K. Mohanakrishnan, A Versatile Synthesis of Annulated Carbazole Analogs Involving a DominoReaction of Bromomethylindoles with Arenes/Heteroarenes, Eur. J. Org. Chem., 2009, 531–546 CrossRef CAS
;
(e) K. Willinger, K. Fischer, R. Kisselev and M. Thelakkat, Synthesis, spectral, electrochemical and photovoltaic properties of novel heteroleptic polypyridyl ruthenium(II) donor-antenna dyes, J. Mater. Chem., 2009, 19, 5364–5376 RSC
;
(f) M. Hori, T. Kataoka, H. Shimizu, J. Hongo and M. Kido, Reactions of 1H-2-benzothiopyran 2-oxides with active methylene compounds: a novel ring contraction of 1-aryl derivatives to benzo[c]thiophenes. X-Ray molecular structure of 1-(2,2-diacetylvinyl)-3-phenylbenzo[c]thiophene, J. Chem. Soc., Perkin Trans. 1, 1989, 1611–1618 RSC
. -
(a) M. Usui, H. Fukumoto and T. Yamamoto, Synthesis of New Thiperkinophene-Based π-Conjugated Organic Compounds and Polymer, Bull. Chem. Soc. Jpn., 2010, 83, 1397–1399 CrossRef CAS
;
(b) Q. Liu, Q.-Y. Feng, H. Yamada, Z.-S. Wang, N. Ono, X.-Z. You and Z. Shen, Tuning the Spectroscopic, Electrochemical, and Photovoltaic Properties of Triaryl Amine Based Sensitizers through Ring-Fused Thiophene Bridges, Chem.–Asian J., 2012, 7, 1312–1319 CrossRef CAS PubMed
;
(c) A. K. Mohanakrishnan, J. A. Clement, P. Amaladass and V. S. Thirunavukkarasu, Synthesis of 1,3-disubstituted benzo[c]thiophene analogs containing benzo[b]thiophene/benzo[b]pyrrole, Tetrahedron Lett., 2007, 48, 8715–8720 CrossRef CAS
;
(d) A. K. Mohanakrishnan, N. S. Kumar and P. Amaladass, Synthesis and characterization of 9,9-dialkylfluorene capped benzo[c]thiophene/benzo[c]selenophene analogs as potential OLEDs, Tetrahedron Lett., 2008, 49, 4792–4795 CrossRef CAS
;
(e) N. S. Kumar, J. A. Clement and A. K. Mohanakrishnan, Synthesis and characterization of benzo[c]thiophene analogs tethered with dibenzo-heterocycles as potential OLEDs, Tetrahedron, 2009, 65, 822–830 CrossRef CAS
;
(f) R. H. L. Kiebooms, P. J. A. Adriaensens, D. J. M. Vanderzande and J. M. J. V. Gelan, Grignard Reactions on Ortho Dicarboxylic Arene Derivatives. Synthesis of 1,3-Dithienylisothianaphthene Compounds, J. Org. Chem., 1997, 62, 1473–1480 CrossRef CAS
. -
(a) J. D. Douglas, G. Griffini, T. W. Holcombe, E. P. Young, O. P. Lee, M. S. Chen and J. M. J. Fréchet, Functionalized Isothianaphthene Monomers That Promote Quinoidal Character in Donor–Acceptor Copolymers for Organic Photovoltaics, Macromolecules, 2012, 45, 4069–4074 CrossRef CAS
;
(b) J. W. Terpstra and A. M. van Leusen, A new synthesis of benzo[b]thiophenes and benzo[c]thiophenes by annulation of disubstituted thiophenes, J. Org. Chem., 1986, 51, 230–238 CrossRef CAS
;
(c) R. M. El-Shishtawy, K. Fukunishi and S. Miki, Synthesis and photoreaction of 4,5,7-tri-t-butyl benzo[c]thiophene: A novel benzo[c]thiophene derivative and its Dewar isomer, Tetrahedron Lett., 1995, 36, 3177–3180 CrossRef CAS
;
(d) J. C. Throgmorton, S. M. Chintala and R. D. McCulla, Synthesis of Unsymmetric Monosubstituted and Disubstituted Dinaphthothiophenes, J. Heterocycl. Chem., 2017, 54, 3682–3688 CrossRef CAS
. -
(a) K. Yamamoto, Y. Ie, M. Nitani, N. Tohnai, F. Kakiuchi, K. Zhang, W. Pisula, K. Asadi, P. W. M. Blom and Y. Aso, Oligothiophene quinoids containing a benzo[c]thiophene unit for the stabilization of the quinoidal electronic structure, J. Mater. Chem. C, 2018, 6, 7493–7500 RSC
;
(b) K. Fukuzumi, Y. Unoh, Y. Nishii, T. Satoh, K. Hirano and M. Miura, Synthesis of Benzo[c]thiophenes by Rhodium(III)-Catalyzed Dehydrogenative Annulation, J. Org. Chem., 2016, 81, 2474–2481 CrossRef CAS PubMed
;
(c) Y.-C. Hu, Z.-L. Lin, T.-C. Huang, J.-W. Lee, W.-C. Wei, T.-Y. Ko, C.-Y. Lo, D.-G. Chen, P.-T. Chou, W.-Y. Hung and K.-T. Wong, New exciplex systems composed of triazatruxene donors and N-heteroarene-cored acceptors, Mater. Chem. Front., 2020, 4, 2029–2039 RSC
;
(d) K. Kobayashi, Y. Honda and Y. Shigemura, An Efficient Synthesis of 1-Arylbenzo[c]thiophenes via the Reaction of 2-(Chloromethyl)phenyllithiums with Aromatic Aldehydes, Heterocycles, 2017, 94, 1707–1717 CrossRef CAS
;
(e) C. P. Yu, R. Kimura, T. Kurosawa, E. Fukuzaki, T. Watanabe, H. Ishii, S. Kumagai, M. Yano, J. Takeya and T. Okamoto, Air-Stable Benzo[c]thiophene Diimide n-Type π-Electron Core, Org. Lett., 2019, 21, 4448–4453 CrossRef CAS PubMed
. -
(a) D.-T. Hsu and C.-H. Lin, Synthesis of Benzo[c] and Naphtho[c]heterocycle Diesters and Dinitriles via Homoelongation, J. Org. Chem., 2009, 74, 9180–9187 CrossRef CAS PubMed
;
(b) W. A. Braunecker, S. D. Oosterhout, Z. R. Owczarczyk, R. E. Larsen, B. W. Larson, D. S. Ginley, O. V. Boltalina, S. H. Strauss, N. Kopidakis and D. C. Olson, Ethynylene-Linked Donor–Acceptor Alternating Copolymers, Macromolecules, 2013, 46, 3367–3375 CrossRef CAS
;
(c) A. Ishii, J. Nakayama, J. Kazami, Y. Ida, T. Nakamura and M. Hoshino, Synthesis and reactions of 1,3,4,6-tetra-2-thienylthieno[3,4-c]thiophene, J. Org. Chem., 1991, 56, 78–82 CrossRef CAS
;
(d) G. M. Brooke and S. D. Mawson, Partially fluorinated heterocyclic compounds. Part 27. The synthesis of 4,5,6,7-tetrafluorobenzo[c]thiophene and 4,5,6,7,8,9-hexafluoronaphtho-[1,2-c]thiophene; some chemistry and electrochemistry, J. Chem. Soc., Perkin Trans. 1, 1990, 1919–1923 RSC
. -
(a) T. Saito, H. Ayukawa, N. Sumizawa, T. Shizuta, S. Motoki and K. Kobayashi, Reaction of aryl biphenyl-2-yl thioketones with ditosyldiazomethane; internal cyclisation of the thioketone S-methylides to afford benzo[c]thiophene derivatives. Structure determination of the products by Raney nickel degradation and X-ray crystallographic analysis, J. Chem. Soc., Perkin Trans. 1, 1991, 1405–1410 RSC
;
(b) R. R. Amaresh, M. V. Lakshmikantham, J. W. Baldwin, M. P. Cava, R. M. Metzger and R. D. Rogers, Condensed Thiophenes and Selenophenes:
Thionyl Chloride and Selenium Oxychloride as Sulfur and Selenium Transfer Reagents, J. Org. Chem., 2002, 67, 2453–2458 CrossRef CAS PubMed
;
(c) D. Rajagopal, M. V. Lakshmikantham, E. H. Mørkved and M. P. Cava, Generation of the First Tellurium-Containing Diheteropentalene, Org. Lett., 2002, 4, 1193–1195 CrossRef CAS PubMed
;
(d) N. Yasmin, M. Ghosh and J. K. Ray, P4S10 and Na2S-mediated novel annulation routes to c-fused thiophenes, RSC Adv., 2014, 4, 19932–19938 RSC
. -
(a) Y. Okuda, M. V. Lakshmikantham and M. P. Cava, A new route to 1,3-disubstituted benzo[c]thiophenes, J. Org. Chem., 1991, 56, 6024–6026 CrossRef CAS
;
(b) E. Aqad, M. V. Lakshmikantham and M. P. Cava, N,N-Dimethylformamide-Mediated Sodium Reduction of trans-3,3′-Benzo[c]thienylidene-1,1′-dithione and trans-3,3′-Benzo[c]selenonylidene-1,1′-dithione, Org. Lett., 2004, 6, 3039–3041 CrossRef CAS PubMed
. -
(a) A. K. Mohanakrishnan, P. Amaladass and J. A. Clement, Synthesis of end-blocked thienyl oligomers incorporating benzo[c]thiophene, Tetrahedron Lett., 2007, 48, 779–784 CrossRef CAS
;
(b) P. Amaladass, J. A. Clement and A. K. Mohanakrishnan, Synthesis and Characterization of Benzannelated Thienyl Oligomers, Eur. J. Org. Chem., 2008, 3798–3810 CrossRef CAS
. -
(a) Y. Shimizu, Z. Shen, S. Ito, Hi. Uno, J. Daub and N. Ono, A convenient synthesis of isothianaphthene oligomers and theirelectrochemical studies, Tetrahedron Lett., 2002, 43, 8485–8488 CrossRef CAS
;
(b) Q. Liu, F.-T. Kong, T. Okujima, H. Yamada, S.-Y. Dai, H. Uno, N. Ono, X.-Z. You and Z. Shen, Synthesis and spectroscopic properties of ring-fused thiophene bridged push–pull dyes and their application in dye-sensitized solar cells, Tetrahedron Lett., 2012, 53, 3264–3267 CrossRef CAS
. - Y. Ooyama, T. Enoki, S. Aoyama and J. Ohshita, Synthesis and optical and electrochemical properties of a phenanthrodithiophene (fused-bibenzo[c]thiophene) derivative, Org. Biomol. Chem., 2017, 15, 7302–7307 RSC
. -
(a) H. Langhals, T. Potrawa, H. Nöth and G. Linti, The Influence of Packing Effects on the Solid-State Fluorescence of Diketopyrrolopyrroles, Angew. Chem., Int. Ed., 1989, 28, 478–480 CrossRef
;
(b) H.-C. Yeh, W.-C. Wu, Y.-S. Wen, D.-C. Dai, J.-K. Wang and C.-T. Chen, Derivative of α,β-Dicyanostilbene:
Convenient Precursor for the Synthesis of Diphenylmaleimide Compounds, E–Z Isomerization, Crystal Structure, and Solid-State Fluorescence, J. Org. Chem., 2004, 69, 6455–6462 CrossRef CAS PubMed
;
(c) Y. Ooyama, T. Okamoto, T. Yamaguchi, T. Suzuki, A. Hayashi and K. Yoshida, Heterocyclic Quinol-Type Fluorophores: Synthesis, X-ray Crystal Structures, and Solid-State Photophysical Properties of Novel 5-Hydroxy-5-substituent-benzo[b]naphtho[1,2-d]furan-6-one and 3-Hydroxy-3-substituent-benzo[kl]xanthen-2-one Derivatives, Chem.–Eur. J., 2006, 12, 7827–7838 CrossRef CAS PubMed
;
(d) Y. Ooyama, Y. Hagiwara, Y. Oda, H. Fukuoka and J. Ohshita, BODIPY dye possessing solid-state red fluorescence and green metallic luster properties in both crystalline and amorphous states, RSC Adv., 2014, 4, 1163–1167 RSC
. - Both the geometry optimization and energy calculation were performed by employing the density functional theory (DFT), at the level of B3LYP/6-31G(d,p) on the Gaussian 16 program package: M. J. Frisch, G. W. Trucks, H. B. Schlegel, G. E. Scuseria, M. A. Robb, J. R. Cheeseman, G. Scalmani, V. Barone, G. A. Petersson, H. Nakatsuji, X. Li, M. Caricato, A. V. Marenich, J. Bloino, B. G. Janesko, R. Gomperts, B. Mennucci, H. P. Hratchian, J. V. Ortiz, A. F. Izmaylov, J. L. Sonnenberg, D. Williams-Young, F. Ding, F. Lipparini, F. Egidi, J. Goings, B. Peng, A. Petrone, T. Henderson, D. Ranasinghe, V. G. Zakrzewski, J. Gao, N. Rega, G. Zheng, W. Liang, M. Hada, M. Ehara, K. Toyota, R. Fukuda, J. Hasegawa, M. Ishida, T. Nakajima, Y. Honda, O. Kitao, H. Nakai, T. Vreven, K. Throssell, J. A. Montgomery, Jr., J. E. Peralta, F. Ogliaro, M. J. Bearpark, J. J. Heyd, E. N. Brothers, K. N. Kudin, V. N. Staroverov, T. A. Keith, R. Kobayashi, J. Normand, K. Raghavachari, A. P. Rendell, J. C. Burant, S. S. Iyengar, J. Tomasi, M. Cossi, J. M. Millam, M. Klene, C. Adamo, R. Cammi, J. W. Ochterski, R. L. Martin, K. Morokuma, O. Farkas, J. B. Foresman, and D. J. Fox, Gaussian 16, Revision B.01, Gaussian, Inc., Wallingford CT, 2016 Search PubMed
.
Footnote |
† Electronic supplementary information (ESI) available. CCDC [2055734, 2055735]. For ESI and crystallographic data in CIF or other electronic format see DOI: 10.1039/d1ra01189h |
|
This journal is © The Royal Society of Chemistry 2021 |