DOI:
10.1039/D1RA00729G
(Paper)
RSC Adv., 2021,
11, 9788-9796
One-step hydrothermal synthesis of a ternary heterojunction g-C3N4/Bi2S3/In2S3 photocatalyst and its enhanced photocatalytic performance†
Received
27th January 2021
, Accepted 2nd March 2021
First published on 5th March 2021
Abstract
In recent years, photoelectrocatalysis has been one of the hotspots of research. Graphite-like carbon nitride (g-C3N4) is one of the few non-metal semiconductors known and has good potential in the field of photocatalysis due to its simple preparation method and visible light effects. In this study, a method for compounding two semiconductor materials, In2S3 and Bi2S3, on the surface of g-C3N4 via a one-step hydrothermal method is reported, and it was found that this resulting material showed remarkable properties. The advantages of this method are as follows: (1) the formation of a heterojunction, which accelerates the separation efficiency of photogenerated carriers; (2) a large number of holes and defects on the surface of g-C3N4 are conducive to the nucleation, crystallisation and growth of In2S3 and Bi2S3. Compared with its counterpart catalysts, the CN/In2S3/Bi2S3 composite catalyst has significantly improved performance. Due to its high degree of crystallinity, the adsorption capacity of the catalyst itself is also significantly improved. In addition, the stability of the composite material maintains 90.9% after four cycles of use, and the structure is not damaged. In summary, CN/Bi2S3/In2S3 composite materials are believed to have broad application potential in the treatment of dye wastewater.
1 Introduction
With the development of science and technology, the application of photoelectrocatalytic technology1–5 is of great benefit to the water pollution caused by the of the global industrialization process.6–9 As a result, different countries and regions worldwide have made great efforts to protect the environment on which people depend.10–12 Fortunately, at the same time, there have been continuous developments in science and technology toward alleviating pollution in water. Common methods that have been developed for dealing with organic pollution in water can be divided into three categories: physical, chemical and biological methods. Adsorption is the main physical method used,13 which mostly involves the use of activated carbon and diatomaceous earth as adsorbents. Other adsorbents have been also reported recently for wastewater treatment, e.g., sepiolite mineral nanofibers.14 However, the costs associated with this method are high and complete adsorption of the pollutants from water cannot be achieved. Oxidation reactions are the main chemical methods used in the remediation of water pollutants.15,16 Therefore, it is urgent to develop an environmentally friendly method to solve the current water pollution issues. As photocatalytic technology has the advantages of being green, highly efficient and causing no secondary pollution, it is one of the most promising methods for degrading pollutants in water that could be applied as a future strategy.17–19
At present, the reports on photocatalysts in the literature are mainly based on semiconductor materials, including titanium dioxide (TiO2),20 molybdenum sulfide (MoS2),21 Bi2S3,22 zinc oxide (ZnO),23 graphitic carbon nitride (g-C3N4).24 The unique forbidden band structures of semiconductors lead to the generation of electrons and holes (e− and h+) under the excitation of light of a certain wavelength. These e− and h+ then react with water molecules and oxygen in water to form strong oxidising substances, which oxidise and decompose the pollutants present in water into environmentally friendly molecules. Among these semiconductors, in 2009 g-C3N4 was first used by Wang et al. in the photocatalytic production of hydrogen. Due to the excellent photoelectric properties of g-C3N4, it has attracted the attention of many researchers in the field of photocatalysis.19,24 In addition to photocatalytic application,25 graphitic carbon nitride based materials are also promising for biomedical applications.26 However, some studies have shown that the rapid recombination of e− and h+ severely limits the photocatalytic performance of g-C3N4.27 To solve and overcome these difficulties, many researchers have made tremendous efforts,28,29 such as using different strategies to improve the catalytic performance of g-C3N4. For example, a higher number of active sites have been introduced in g-C3N4 by increasing its specific surface area,30 however, the coverage of dyes and other small molecules on the catalyst surface and in the pores makes the catalyst less stable. The latest research shows that a strategy of constructing heterojunction binary and multi-component catalysts not only accelerates the separation of e− and h+ but also expands the range of light response of these materials has attracted great attention.31–33 It has been reported in the literature that the construction of heterostructured catalysts leads to systems that have greatly improved photocatalytic performance.34–37 C. Auttaphon et al.38 synthesised a Z-scheme Bi2S3/ZnIn2S4 heterostructure for methylene blue degradation, which showed higher photocatalytic activity than the uncombined catalysts alone. Geioushy et al. used a one-pot method to prepare BiPO4/Bi2S3 for the reduction of harmful Cr(VI) driven by visible light.39 ABi2S3/Bi2W2O9 composite has also previously been prepared that shows 80% degradation of phenol after 120 min of irradiation, a performance that is substantially higher than those of pure Bi2S3 and Bi2W2O9 under visible-light illumination.40 An et al. synthesised a new Z-scheme In2S3/graphene heterojunction with a core–shell structure, which showed a methyl orange degradation rate of almost five times higher than that of pure In2S3 (ref. 41) and reduced Cr(VI) under visible light. For degradation of rhodamine B (RhB), these photocatalysts have been reported recently.42,43 In summary, from the perspective of development, photocatalytic technology has great application prospects in the degradation of pollution and environmental protection.44,45
In this study, to verify the heterostructure photocatalyst theory, the ternary heterostructured photocatalyst g-C3N4/Bi2S3/In2S3 was prepared via a one-step hydrothermal method. The modification of microscale porous spherical Bi2S3 and dendritic In2S3 on the surface of porous g-C3N4 with a large specific surface area significantly improves its optical, physical and photoelectric conversion properties. Compared with the performances of the g-C3N4, Bi2S3 and In2S3 catalysts alone, and Bi2S3/In2S3 binary heterojunction catalysts, the obtained g-C3N4/Bi2S3/In2S3 ternary heterostructured photocatalyst shows significantly improved degradation of rhodamine B (RhB). In addition, by carrying out powder X-ray diffraction (XRD), photoluminescence (PL), X-ray photoelectron spectroscopy (XPS) and other characterisation methods, the composition of the catalyst in terms of its elemental content, its chemical valence state, morphology, and charge carrier transfer behaviour were carefully analysed, and the possible catalytic mechanism of the catalyst was explored and is discussed in detail. This work may provide a feasible practical example of a way of improving the photocatalytic performance of g-C3N4 through its combination with Bi2S3 and In2S3 in a heterogeneous composition as a reference for subsequent research.
2 Experimental
2.1 Materials
Thiourea, absolute ethanol, RhB, urea, bismuth nitrate pentahydrate, indium nitrate, and other chemicals used in the experiments were of analytical purity (99%) and used without further purification. Deionised water was used throughout all of the experimental work in this study. All medicines come from Titan.
2.2 Sample preparation
2.2.1 Preparation of the g-C3N4 photocatalyst. An appropriate amount of urea was added to a crucible and closed the lid, and heated in a muffle furnace at a heating rate of 2.5 °C min−1 to 550 °C, and held at this temperature for 4 h to obtain a yellow solid of g-C3N4, which was ground into a powder for subsequent use.
2.2.2 Pretreatment scheme of g-C3N4. An appropriate amount of g-C3N4 powder was added to a 50 mL beaker, into which 20 mL of DMF (N,N-dimethylformamide) was poured and the reaction mixture was stirred at room temperature for 12 h to prepare a solution referred to as solution A.
2.2.3 Preparation of g-C3N4/Bi2S3/In2S3. 70 mL of HON3 solution with a concentration of 1 mol L−1 was added to a 100 mL beaker and stirred for ten min before adding 0.1 mmol of Bi (NO3) 5H2O, and stirring the resulting reaction mixture for 30 min. Then, to this mixture 0.1 mmol of In (NO3) xH2O was added and the mixture was stirred for 30 min. After this time 0.2 g of cetrimonium bromide (CTAB) was added and the reaction mixture was ultrasonicated for 30 min, to produce a solution referred to as solution B. The above solution A was slowly added dropwise to solution B to mix them together uniformly, then 0.6 g of thiourea was added and the reaction mixture was stirred for 30 min, before being poured into a 100 mL polytetrafluoroethylene liner and heated to 140 °C under hydrothermal reaction for 12 h. The resulting liquid was washed three times with ethanol and deionised water, and then dried in an oven overnight at 60 °C. g-C3N4/Bi2S3/In2S3 catalysts with different mass fractions of g-C3N4 were prepared, and the catalysts g-C3N4 and Bi2S3/In2S3 were prepared for comparison.
2.3 Characterisation of the photocatalysts
XRD patterns were recorded using a Bruker D8 Advance powder X-ray diffractometer equipped with a Cu-Kα radiation source. A Nicolet iS10 Fourier-transform infrared (FTIR) spectrometer was employed to record FTIR spectra of the samples in the wavenumber range of 500–4000 cm−1. XPS measurements of the prepared samples were recorded using a PHI 5000 Versa Probe spectrometer, in which the binding energy positions were calibrated against the C–C bond peak that has a binding energy of 284.8 eV. The morphology characteristics of the samples were investigated using transmission electron microscopy (TEM, Tecnai G F20, Hitachi, HT7700) and scanning electron microscopy (SEM, Hitachi S-4800). UV-visible diffuse reflection spectroscopy (DRS) measurements were carried out using a UV-visible spectrophotometer (Shimadzu UV-3600) across a wavelength range of 200–800 nm, employing BaSO4 as a benchmark. Fluorescence emission and time-resolved fluorescence spectra were measured using a fluorescence spectrometer (Hitachi F-7000) over a wavelength range of 340–800 nm at an excitation wavelength of 320 nm, employing a 300 W xenon lamp (CEL-HXF300) as a light source.
2.4 Photocatalytic activity
To determine the photocatalytic activity of the synthesised photocatalyst, the catalytic degradation of RhB was evaluated under simulated sunlight using a 500 W xenon lamp as a light source in a photoreactor (CEL-HXF300, Beijing, China). In a typical experiment, before being exposed to light the reactants and catalyst were placed in a quartz tube and magnetically stirred for 30 min in the dark to achieve adsorption–desorption equilibrium. At a given time interval after beginning the visible light irradiation, samples were taken from the reaction suspension (each with a volume of 5 mL), centrifuged at 8000 rpm for 10 minutes, and then the supernatant was removed. Then, the concentration of RhB in the sample solutions was analysed using a (UV-31/32/3300) UV-visible spectrometer (MAPADA) at its maximum absorption wavelength. To ensure the repeatability of the results, each run was repeated to obtain an average for each set of conditions. A blank test was also carried out by irradiating the reactant solution in the absence of the catalyst to evaluate its photo-induced self-sensitised photodegradation.
where η is the photocatalytic efficiency; C0 is the concentration of the reactant before illumination and Ct is the concentration of the reactant after illumination for t hour.
3 Results and discussion
3.1 Morphology and structure
3.1.1 XRD analysis. As shown in Fig. 1, XRD was conducted to identify the crystallographic and phase structures of Bi2S3, CN, In2S3, Bi2S3/In2S3 and CN-xBi2S3/In2S3. The XRD pattern of pure CN shows two distinct diffraction peaks at 2θ = 12.43° and 27.54°, which can be indexed to the (002) and (100) diffraction planes, respectively, of g-C3N4 (JCPDS (87-1526)), attributed to its typical stacked aromatic rings and interlayer π–π stacking.46 Fig. 1 shows distinct diffraction peaks at 2θ = 11.14°, 15.80°, 15.90°, 17.58°, 22.39°, 23.72°, 24.92°, 25.20°, 28.60°, 31.65°, 31.79°, 32.93°, corresponding to the (110), (020), (200), (120), (220), (101), (130), (310), (211), (040), (221), (301) diffraction planes respectively, of Bi2S3 (JCPDS No. 17-0320).22 The profile of In2S3 exhibits the characteristic diffraction peaks of β-In2S3 (JPCDS No. 65-0459).47 However, the XRD pattern of In2S3/Bi2S3 only shows the diffraction peaks of Bi2S3, showing that it contains little In2S3 content. In addition, one new weak characteristic peaks at 27.54° is present in the patterns of the CN/Bi2S3/In2S3 composites, which can be assigned to the (0 0 2) peak of g-C3N4. With an increase in the amount of g-C3N4 present in the catalyst samples, the diffraction peak of Bi2S3/In2S3 at 2θ = 24.92° went from being of high intensity to low intensity, reflecting the influence that it has on the phase crystallinity of Bi2S3/In2S3.
 |
| Fig. 1 The typical XRD patterns of CN, Bi2S3/In2S3 and CN/Bi2S3/In2S3. | |
3.1.2 SEM/TEM analysis. In Fig. 2a the layered porous structure of g-C3N4 can be seen in CN-xBi2S3/In2S3, which is beneficial for the adsorption of dyes by the catalyst. Fig. 2b shows an image of the structure of In2S3, which is made up of a large number of hexagons with side lengths ranging from 0.3–1 μm. Fig. 2c shows an image of Bi2S3, which has a rod-shaped structure, where the rods have diameters in the range of 50–100 nm and a length in the range of 0.5–2.0 μm in size.
 |
| Fig. 2 SEM images of (a) g-C3N4; (b)In2S3; (c) Bi2S3; (d) Bi2S3/In2S3; (e) 0.05CN/Bi2S3/In2S3; TEM images of (f) g-C3N4; (g) Bi2S3/In2S3 and HRTEM images of (h) 0.05CN/Bi2S3/In2S3. | |
Compared with pure In2S3 and Bi2S3, the morphology of the composite of both materials in Fig. 2d shows a spherical structure of In2S3 surrounded by rod-shaped Bi2S3. This structure is more conducive to the transfer of photogenerated e− and h+ than the single catalysts alone. Fig. 2e shows an SEM image of 0.05CN/Bi2S3/In2S3, which shows the combination of flower-like In2S3 and a spherical Bi2S3 structure. Porous layered g-C3N4 has a large specific surface area and pores, so it provides more defects with which to facilitate the crystallisation, nucleation and growth of In2S3 and Bi2S3. In addition, the structures of In2S3 and Bi2S3 are not damaged. To analyse its structure in more depth, TEM and high-resolution TEM (HRTEM) were used to characterise 0.05CN/Bi2S3/In2S3. Fig. 2f shows a TEM image of g-C3N4, from which it can be seen that the layered g-C3N4 of the film structure is consistent with the results presented in the literature. Fig. 2g shows a TEM image of Bi2S3/In2S3, which is consistent with the image shown in Fig. 2d. Fig. 2h shows a HRTEM image of 0.05CN, in which it can be clearly seen that there are two lattice fringes with different widths of 0.352 and 0.278 nm, corresponding to the (1 3 0) crystal plane of Bi2S3 and the (4 0 0) crystal plane of In2S3. This result is consistent with the PXRD results.
3.1.3 FTIR analysis. To further demonstrate the surface properties and functional groups present in the samples, FTIR spectra of In2S3, Bi2S3, g-C3N4 and CN-xBi2S3/In2S3 were recorded and the results are presented in Fig. 3. In the FTIR spectrum of CN-xBi2S3/In2S3 there is no obvious peak that is characteristic of Bi2S3.48 The peaks at 1327, 1417, 1559, and 1631 cm−1 in the same spectrum can be assigned as the characteristic absorption bands of g-C3N4, attributed to the vibration of the C6N7 skeleton and the typical stretching mode of the CN heterocycles.49
 |
| Fig. 3 The FTIR spectra of CN, Bi2S3/In2S3 and CN-xBi2S3/In2S3. | |
The peak at around 809 cm−1 can be assigned to the breathing mode of the triazine units. In addition, the peaks at 772 and 1196 cm−1 are typical of In2S3 and the characteristic peak of Bi2S3/In2S3 is roughly consistent with In2S3.50,51 The characteristic absorption peak of g-C3N4/In2S3/Bi2S3 is consistent with that of g-C3N4, while the peak related to Bi2S3/In2S3 gradually decreases in intensity, which may be a result of the g-C3N4 peak being too intense.
3.1.4 XPS analysis. To study the surface chemical states and chemical compositions of the CN/Bi2S3/In2S3 heterojunction samples, Fig. 4 shows XPS measurements of the samples, in which it can be seen that the survey spectrum of CN–In2S3/Bi2S3 exhibits the typical C 1s, S 2p, S 2s, N 1s, In 3d, and Bi 4f peaks expected for CN–Bi2S3/In2S3, Bi2S3/In2S3 and CN. The survey spectrum indicates that C and N exist on the surface of g C3N4, and Bi, In, and S on the surface of In2S3/Bi2S3. Besides this, as shown in Fig. 4a, Bi, In and S were found to be present on the surface of the g-C3N4 nanosheets, since the peaks at around 443.61, 157.43, and 225.01 eV can be assigned to the In 3d, Bi 4f and S 2s energy levels, respectively. The high-resolution C 1s, N 1s, In 3d, Bi 4f and S 2s XPS spectra of 0.05CN/Bi2S3/In2S3 are presented in Fig. 4b–f. Moreover, the binding energy peak of the N–C
N groups in the 0.05CN–Bi2S3/In2S3 heterostructure shifts lowers by 0.02 eV to 288.14 eV, which indicates that there are weak interactions between the Bi2S3/In2S3 and g-C3N4 nanosheets as a result of surface modification effects.52 The N 1s Gaussian curve in Fig. 4b can be deconvolved into three peaks that have binding energies of 398.47, 400.20, and 401.90 eV, corresponding to sp2-hybridised nitrogen (C–N
C), the N–(C)3 groups of the skeleton, and the surface uncondensed bridging N atoms with C–N–H functional groups attached.53,54 Compared to g-C3N4, the peak positions in the N 1s spectrum of 0.05CN/Bi2S3/In2S3 are shifted to higher binding energies. In the In 3d spectrum (Fig. 4d), the In 3d3/2 and In 3d5/2 peaks of 0.05CN/Bi2S3/In2S3 are located at 452.23 and 444.58 eV, which indicates a slight shift to higher binding energies compared to pristine In2S3 (451.39 and 443.87 eV). As shown in Fig. 4e, the Bi 4f XPS spectrum of Bi2S3 displays clearly two peaks (162.73 and 157.43 eV), which can be attributed to the 4f5/2 and 4f7/2 of Bi3+. Moreover, compared to Bi2S3, the peak positions (163.50 and 158.18 eV) of Bi 4f in 0.05CN/Bi2S3/In2S3 are shifted to higher binding energies. Since the peak of Bi 4f covers the characteristic peak of S 2p, S 2s is added to illustrate the existence of S2−. As shown in Fig. 4f, the S 2s XPS spectrum of Bi2S3/In2S3 shows three peaks at 221.0, 224.0, and 224.9 eV. Compared with Bi2S3/In2S3, 0.05CN/Bi2S3/In2S3 shows peaks that are slightly shifted to higher binding energies (222.6, 225.01, and 227.17 eV). These results suggest the successful heterogeneous growth of In2S3 and Bi2S3 on the g-C3N4 nanosheets.
 |
| Fig. 4 XPS spectra of the CN, In2S3/Bi2S3, and 0.05CN/In2S3/Bi2S3; (a) survey, (b) C 1s, (c) N 1s, (d) In 3d, (e) Bi 4f, and (f) S 2s spectra. | |
3.1.5 UV-vis analysis. To investigate the modification effect that the g-C3N4 nanosheets have on the Bi2S3/In2S3 heterostructure, the optical absorption properties of the as-prepared samples were analysed using UV-vis spectroscopy, the results of which are shown in Fig. 5a. The g-C3N4 shows good light absorption at a wavelength of less than 450 nm. The light absorption of In2S3 and Bi2S3 seem much higher than that of g-C3N4, at a wavelength of 1000 nm. Compared with Bi2S3/In2S3, the absorption edge of the g-C3N4/Bi2S3/In2S3 heterostructure exhibits a distinct red shift, which may originate from the modification effect of Bi2S3/In2S3 on the surface of the g-C3N4 nanosheets. This signifies that a strong interaction is formed between g-C3N4 and Bi2S3/In2S3, which is beneficial to improving the separation efficiency of charge carriers and the stability of the heterostructure. In addition, the band gap energies (Eg) of the samples were calculated using the Tauc equation:
where α, hν, A and Eg are the absorption coefficient, photoenergy, proportionality constant and band gap, respectively. As shown in Fig. 5b, the band gaps of g-C3N4, In2S3 and Bi2S3 are 2.84, 2.2, and 1.30 eV, respectively. In addition, the band gap of Bi2S3/In2S3 is estimated to be 1.26 eV, and the band gaps of 0.01CN/Bi2S3/In2S3 and 0.05CN/Bi2S3/In2S3 are 1.20 and 1.18 eV, respectively. Compared with Bi2S3/In2S3, the band gap of 0.05CN/Bi2S3/In2S3 is narrower, which indicates that the heterostructure of 0.05CN/Bi2S3/In2S3 is more easily excited by visible light and is beneficial for improving photocatalytic performance due to the modification of large holes and defects on the surface of the g-C3N4 nanosheets.
 |
| Fig. 5 (a) UV-vis DRS of g-C3N4, In2S3, Bi2S3, Bi2S3/In2S3, 0.01CN/Bi2S3/In2S3, and 0.05CN/Bi2S3/In2S3 and (b) plot of (αhv)n/2 versus hv. | |
3.1.6 PL spectra and photocurrent analysis. To further understand the reasons for the improvement in the photocatalytic activity of the composite photocatalyst, the PL spectra were measured to investigate the separation efficiency of photo-induced charge carriers.55 The PL spectra of g-C3N4, Bi2S3/In2S3 and 0.05 g-C3N4/Bi2S3/In2S3 are shown in Fig. 6a. The PL emission stems from the radiative recombination between the excited e− and h+ and the weaker the PL peak intensity is, the slower the recombination rate of the excited e− and h+. Comparing the PL emission spectra of the three materials, the 0.05 g-C3N4/Bi2S3/In2S3 heterostructure shows low fluorescence intensity relative to that shown by g-C3N4 and Bi2S3/In2S3. These results indicate that the efficient interfacial charge transfer between Bi2S3/In2S3 and g-C3N4 hinders the recombination of e− and h+ pairs. In addition, Fig. 6b is the photocurrent curve of the catalysts. We can clearly see that the photocurrent value of the composite material is the highest, and the conclusion corresponds to Fig. 6a.
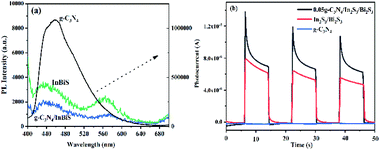 |
| Fig. 6 PL spectra (a) and photocurrent (b) of the g-C3N4, Bi2S3/In2S3 and 0.05 g-C3N4/Bi2S3/In2S3 composites. | |
3.2 Photocatalytic activity
Fig. 7a shows the degradation RhB by the different catalysts. It can be clearly seen that the 0.05 g-C3N4 composite catalyst shows the best catalytic activity, which almost completely degrades the dye after 45 min of exposure to light. Fig. 7b shows the first-order kinetic fitting curve of the degradation, and the results obtained are consistent with the results shown in the degradation curve. Fig. 7c shows a histogram of the catalyst fitting coefficient. The fitting coefficient of the 0.05 g-C3N4/Bi2S3/In2S3 catalyst is 0.02826, which is significantly higher than the values for g-C3N4, In2S3 and Bi2S3. Compared with recently published articles, this study has advantages in degradation efficiency.56–58
 |
| Fig. 7 (a) Influence that the illumination time has on the photocatalytic degradation of the catalysts, (b) the pseudo-first-order dynamics, and (c) the apparent rate constant of the degradation reaction. | |
3.3 Research on the mechanism of photocatalysis
Fig. 8a–c show the valence band spectra of g-C3N4, In2S3 and Bi2S3 respectively (1.95, 0.32, and −0.35 eV). From the results obtained and shown in Fig. 5b, the valence (VB) and conduction bands (CB), respectively, of g-C3N4 (1.95 and −0.89 eV), In2S3 (0.32 and −1.88 eV), and Bi2S3 (−0.35 and −1.65 eV) can be calculated, which facilitate the subsequent analysis of the mechanism of the photocatalyst degradation. Fig. 8e shows the particle capture experiment performed by the catalyst, using isopropanol (IPA), 1,4-benzoquinone (BQ), and Na2C2O4 as the reactive species scavengers for ˙OH, ˙O2−, and h+, respectively.59 It can be seen that BQ, IPA and EDTA-2NA all have different degrees of influence on the catalytic performance of the catalyst. Among them, IPA has the greatest degree of influence, with a degradation rate of 28.3%, whereas EDTA-2Na has the lowest degree of influence and a degradation rate of 65.3%. This shows that photogenerated e− have a greater impact on the ternary heterostructured nanocomposite g-C3N4/In2S3/Bi2S3 than h+. Fig. 8e shows the possible reaction pathway of the photogenerated carriers of the ternary heterostructured nanocomposite g-C3N4/In2S3/Bi2S3 catalyst. When the catalyst is excited by light, e− transition from the VB to the CB. Due to the difference in electronegativity, the excited e− will be transferred from the CBs of In2S3 and Bi2S3 to the CB of g-C3N4. At the same time, h+ will be transferred from the VB of g-C3N4 to the VBs of In2S3 and Bi2S3, and can also oxidise pollutants. The transfer speed of h+ and e− is improved, and the recombination of photogenerated carriers is suppressed, which improves the photocatalytic performance of the material. It is speculated that the photocatalysis may proceed in the following way: |
CN/Bi2S3/In2S3 + hν → e− + h+
| (1) |
|
H2O2+ ˙O2− → ˙OH + OH− + O2
| (7) |
 |
| Fig. 8 (a)–(c) show the valence band diagrams of g-C3N4, In2S3 and Bi2S3; (d) the free radical trapping experiments for the degradation of RhB over the 0.05CN/Bi2S3/In2S3 composite. (e) The proposed photocatalytic mechanism for the degradation of RhB on the surface of the 0.05CN/Bi2S3/In2S3 composite. | |
Of these, (2), (4), and (7) are thought to be the main photocatalytic reactions that occur.
3.4 Stability testing
Fig. 9a shows the stability test results of the 0.05 g-C3N4/Bi2S3/In2S3 catalyst. After repeating the degradation test four times, the degradation performance of the catalyst still reaches 90.9. Therefore, it can be seen that the heterostructure of the composite catalyst not only improves its activity, but also its stability. To further assess whether the structure of the catalyst is damaged after use, PXRD measurements were carried out on the catalyst before and after its use, and the results are shown in Fig. 9b. It can be seen that there is basically no change in the PXRD peak patterns of the catalyst before and after use. Therefore, it can therefore be concluded that the catalyst structure is not destroyed after use.
 |
| Fig. 9 (a) Cycling runs of 0.05CN/Bi2S3/In2S3 in the degradation of RhB under visible light. (b) PXRD pattern of 0.05CN/Bi2S3/In2S3 before and after carrying out the photocatalytic experiments. | |
4 Conclusions
In this paper, a one-step hydrothermal method was used to synthesise a 3D dendritic and porous spherical ternary heterostructure nanocomposite g-C3N4/In2S3/Bi2S3. The g-C3N4/In2S3/Bi2S3 heterostructure catalyst shows significantly improved degradation of RhB compared with the components from which it is made. The reason for the improved photocatalytic performance can be attributed to the increase in the specific surface area of the catalyst, meaning that it has more active sites; the formation of 3D dendritic and porous spherical materials enhances the adsorption performance of the catalyst and the composition of the heterostructure catalyst accelerates the separation of photogenerated carriers, thereby promoting the production of O2−, OH, and h+. The research herein describes the design of a new type of ternary heterostructure catalyst. The disadvantage here is that the decomposition process of the degradants was not able to be analysed in detail, which remains a subject for future work.
Conflicts of interest
The authors declare that they have no competing interests.
Acknowledgements
We are grateful for grants from Natural Science Foundation of China (No. 21766029).
Notes and references
- Z. Wenwu, X. Beibei, L. Zhiping, W. Zongpeng, H. Liangai, S. Shijie, Z. Qinghua and G. Lin, RhSe2: A Superior 3D Electrocatalyst with Multiple Active Facets for Hydrogen Evolution Reaction in Both Acid and Alkaline Solutions, Adv. Mater., 2021, 2007894 Search PubMed.
- Z. Wang, Z. Lin, J. Deng, S. Shen and L. Gu, Elevating the d and Center of Six ordinated Octahedrons in Co9S8 through Fe ncorporated Topochemical Deintercalation, Adv. Energy Mater., 2020, 11, 2003023 CrossRef.
- C. Yang, Z. Y. Zhao, H. T. Wei, X. Y. Deng and Q. J. Liu, DFT calculations for single-atom confinement effects of noble metals on monolayer g-C3N4 for photocatalytic applications, RSC Adv., 2021, 11, 4276–4285 RSC.
- X. Li, H. Jiang, C. Ma, Z. Zhu and X. Li, Local surface plasma resonance effect enhanced Z-scheme ZnO/Au/g-C3N4 film photocatalyst for reduction of CO2 to CO, Appl. Catal., B, 2021, 283, 119638 CrossRef CAS.
- M. Cao, K. Wang, I. Tudela and X. Fan, Improve photocatalytic performance of g-C3N4 through balancing the interstitial and substitutional chlorine doping, Appl. Surf. Sci., 2021, 536, 147784 CrossRef CAS.
- L. Lyu, X. Wang, X. Sui, Z. Liu, Y. Yuan and C. Lin, Heavy Metal Pollution and Ecological Risk Assessment of Cultivated Land Soil in the Farming Areas of Coastal China: A Case Study of Donghai County, Jiangsu Province, Agric. Biotechnol., 2018, 7, 125–129 Search PubMed.
- M. Iqbal, J. H. Syed, K. Breivik, M. J. I. Chaudhry, J. Li, G. Zhang and R. N. Malik, E-Waste Driven Pollution in Pakistan: The First Evidence of Environmental and Human Exposure to Flame Retardants (FRs) in Karachi City, Environ. Sci. Technol., 2017, 51, 13895–13905 CrossRef CAS PubMed.
- G. Imen, Z. Ines, B. Catia, C. Angelo and G. Ahmed, Seasonal occurrence, source evaluation and ecological risk assessment of polycyclic aromatic hydrocarbons in industrial and agricultural effluents discharged in Wadi El Bey (Tunisia), Environ. Geochem. Health, 2018, 40, 1609–1627 CrossRef PubMed.
- S. Varol and K. İlknur, Effect on human health of the arsenic pollution and hydrogeochemistry of the Yazr Lake wetland (Burdur/Turkey), Environ. Sci. Pollut. Res., 2018, 25, 16217–16235 CrossRef CAS PubMed.
- H. Tan, J. Luo, G. I. Orderud, Y. Zheng, J. J. Pan and E. Studies, The Pollution Caused by Protection: The Unintended Consequences of the Local Governance of the Urban Drinking Water Source Protection in Tianjin, China, Chinese Journal of Urban and Environmental Studies, 2015, 3, 1–20 CrossRef.
- M. E. Kahn, P. Li and D. Zhao, Water Pollution Progress at Borders: The Role of Changes in China's Political Promotion Incentives, American Economic Journal, 2015, 7, 223–242 Search PubMed.
- F. Wu, C. Feng, R. Zhang, Y. Li and D. Du, Derivation of water quality criteria for representative water-body pollutants in China, Sci. China: Earth Sci., 2012, 55, 900–906 CrossRef CAS.
- W. Tan, Y. Wang, S. Sun and F. Wu, Zeolite for removal of organic micro-pollutants in water, Miner. Process., 2011, 2, 73–76 Search PubMed.
- W. Fei, H. Ming, L. Jinsheng, G. Peizhang, Z. Maomao, F. Baizeng, Z. Hui and S. Zengyao, A facile fabrication of sepiolite mineral nanofibers with excellent adsorption performance for Cd2+ ions, RSC Adv., 2019, 9, 40184–40189 RSC.
- M. Hassan, H. Olvera-Vargas, X. Zhu, B. Zhang and Y. He, Microbial electro-fenton: an emerging and energy-efficient platform for environmental remediation, J. Power Sources, 2019, 424, 220–244 CrossRef CAS.
- L. Guangfu, L. Chunxue, L. Xinzheng and F. Baizeng, Emerging polymeric carbon nitride Z-scheme systems for photocatalysis, Cell Rep. Phys. Sci., 2021, 2, 100355 CrossRef.
- J. Chaoran, L. Ki Yip, M. A. P. Christopher, K. B. Mustafa, L. Chi Ching, R. Qiushi, J. A. M. Savio, F. L. Adam and T. Junwang, Size-controlled TiO2 nanoparticles on porous hosts for enhanced photocatalytic hydrogen production, Appl. Catal., A, 2015, 521, 133–139 Search PubMed.
- A. Ning, M. Yuwei, L. Juming, M. Huiyan, Y. Jucai and Z. Qiancheng, Enhanced visible-light photocatalytic oxidation capability of carbon-doped TiO2 via coupling with fly ash, Chin. J. Catal., 2018, 39, 1890–1900 CrossRef.
- T. Zhenwei, Y. Dong, X. Tianxiong, T. Yao and J. Zhongyi, Biomimetic fabrication of g-C3N4/TiO2 nanosheets with enhanced photocatalytic activity toward organic pollutant degradation, Chem. Eng. J., 2015, 260, 117–125 CrossRef.
- W. Xin, X. Yuren, Z. Benqing, Z. Youming, W. Jiatao, H. Rui, L. Liwei, S. Jun and Q. Junle, Enhanced photocatalytic performance of Ag/TiO2 nanohybrid sensitized by black phosphorus nanosheets in visible and near-infrared light, J. Colloid Interface Sci., 2019, 534, 1–11 CrossRef PubMed.
- Z. Fenfen, R. Yuefei, W. Junmin, H. Zhiwen, P. Zhiqin and W. Bing, MoS2 quantum dots@TiO2 nanotube composites with enhanced photoexcited charge separation and high-efficiency visible-light driven photocatalysis, Nanotechnology, 2018, 29, 105403 CrossRef PubMed.
- G. Ruonan, Z. Guixian, G. Yingjie, L. Bo, G. Jianfeng and C. Xiuwen, Synthesis of 3D Bi2S3/TiO2 NTAs photocatalytic system and its high visible light driven photocatalytic performance for organic compound degradation, Sep. Purif. Technol., 2019, 226, 31–39 CrossRef.
- T. Pham Van, P. Tran Thi, T. Vu Thi, N. Sang Xuan and K. Tran Ngoc, In situ hydrothermal fabrication and photocatalytic behavior of ZnO/reduced graphene oxide nanocomposites with varying graphene oxide concentrations, Mater. Sci. Semicond. Process., 2020, 115, 105–114 Search PubMed.
- S. Zhichao, Y. Zhiquan, L. Yingya, S. Chuan, Z. Mingshan and W. Anjie, Construction of 2D/2D BiVO4/g-C3N4 nanosheet heterostructures with improved photocatalytic activity, J. Colloid Interface Sci., 2019, 533, 251–258 CrossRef PubMed.
- G. Liao, Y. Gong, L. Zhang, H. Gao, G. J. Yang and B. Fang, Semiconductor polymeric graphitic carbon nitride photocatalysts: the “holy grail” for the photocatalytic hydrogen evolution reaction under visible light, Energy Environ. Sci., 2019, 12, 2080–2147 RSC.
- G. Liao, F. He and Q. Li, et al., Emerging graphitic carbon nitride-based materials for biomedical applications, Prog. Mater. Sci., 2020, 112, 100666 CrossRef CAS.
- C. Fei, Y. Wenjing, C. Wenbo, W. Feiyan, D. Baoqing and H. Xuefeng, The construction and enhanced photocatalytic performance of binary composite S/g-C3N4, Mater. Sci. Semicond. Process., 2018, 87, 1–6 CrossRef.
- B. Natkritta, W. Natda, P. Sukon, W. David, S. Peter, N. Andrew, C. Jun and I. Burapat, Enhanced visible-light photocatalytic activity of g-C3N4/TiO2 films, J. Colloid Interface Sci., 2014, 417, 402–409 CrossRef PubMed.
- W. Yaping, L. Yike, Z. Jingli, W. Jianshe and L. Zhongjun, g-C3N4/B doped g-C3N4 quantum dots heterojunction photocatalysts for hydrogen evolution under visible light, Int. J. Hydrogen Energy, 2018, 44, 618–628 Search PubMed.
- L. Al-Hajji, A. I. Adel, M. F. Atitar, I. Abdelfattah and E.-T. Ahmed Mohamed, Construction of mesoporous g-C3N4/TiO2 nanocrystals with enhanced photonic efficiency, Ceram. Int., 2018, 45, 1265–1272 CrossRef.
- X. Wu, L. Cao, J. Song, Y. Si and B. Ding, Thorn-like flexible Ag2C2O4/TiO2 nanofibers as hierarchical heterojunction photocatalysts for efficient visible-light-driven bacteria-killing, J. Colloid Interface Sci., 2020, 560, 681–689 CrossRef CAS PubMed.
- X. Liu, S. Gu, X. Zhang, X. Li and W. Li, The production discipline and mechanism of hydroxyl radical by investigating the Ln2O3-Bi2MoO6 heterojunction photocatalysts, J. Alloys Compd., 2021, 158894 CrossRef CAS.
- K. I. Katsumata, R. Motoyoshi, N. Matsushita and K. Okada, Preparation of graphitic carbon nitride (g-C3N4)/WO3 composites and enhanced visible-light-driven photodegradation of acetaldehyde gas, J. Hazard. Mater., 2013, 260, 475–482 CrossRef CAS PubMed.
- Y. Junqing, W. Huan, C. Hong, Z. Yunxia, Z. Fuxiang and L. Shengzhong Frank, Fabrication of TiO2/C3N4 heterostructure for enhanced photocatalytic Z-scheme overall water splitting, Appl. Catal., B, 2016, 16, 130–137 Search PubMed.
- J. Wang and W. D. Zhang, Modification of TiO2 nanorod arrays by graphite-like C3N4 with high visible light photoelectrochemical activity, Electrochim. Acta, 2012, 71, 10–16 CrossRef CAS.
- G. Yang, L. Jinhai, G. Zhanqi, Z. Xin, L. Ying, W. Zhongbo, Z. Wei and S. Cheng, A simple and effective method for fabricating novel p–n heterojunction photocatalyst g-C3N4/Bi4Ti3O12 and its photocatalytic performances, Appl. Catal., B, 2016, 192, 57–71 CrossRef.
- L. Chunmei, Y. Siyu, D. Hongjun, L. Chunbo, W. Haijun, C. Huinan and C. Gang, Z-scheme mesoporous photocatalyst constructed by modification of Sn3O4 nanoclusters on g-C3N4 nanosheets with improved photocatalytic performance and mechanism insight, Appl. Catal., B, 2018, 238, 284–293 CrossRef.
- C. Auttaphon, P. Watcharapong, L. Tawanwit, T. Titipun, T. Somchai, K. Sila and K. Sulawan, Enhanced photocatalytic degradation of methylene blue by a direct Z-scheme Bi2S3/ZnIn2S4 photocatalyst, Mater. Res. Bull., 2019, 111, 53–60 CrossRef.
- R. A. Geioushy, S. M. El-Sheikh, B. A. Ahmed, S. Bahaa Ahmed and M. E.-D. Farida, One-pot fabrication of BiPO4/Bi2S3 hybrid structures for visible-light driven reduction of hazardous Cr(VI), J. Hazard. Mater., 2020, 381, 120955 CrossRef CAS PubMed.
- P. B. Yagna, M. Dibyananda, D. Krishnendu and G. M. Braja, Visible Light Assisted Photocatalytic Degradation of Phenolic Compounds Using Bi2S3/Bi2W2O9 Heterostructure Materials as Photocatalyst, Chemistryselect, 2019, 4, 3423–3431 CrossRef.
- A. Xiaoqiang, C. Y. Jimmy, W. Feng, L. Chuanhao and L. Yecheng, One-pot synthesis of In2S3 nanosheets/graphene composites with enhanced visible-light photocatalytic activity, Appl. Catal., B, 2013, 129, 80–88 CrossRef.
- W. Zhong, S. Shen, S. Feng, Z. Lin, Z. Wang and B. Fang, Facile fabrication of alveolate Cu2−x Se microsheets as a new visible-light photocatalyst for discoloration of rhodamine B, CrystEngComm, 2018, 20, 7851–7856 RSC.
- L. Guangfu, F. Jiasheng, L. Qing, L. Sihan, X. Zushun and F. Baizeng, Ag-based Nanocomposites: Synthesis and Applications in Catalysis, Nanoscale, 2019, 11, 7062–7096 RSC.
- M. F. R. Samsudin, A. Dumas, R. Bashiri, N. M. Mohammed and S. J. Sufian, Development of the g-C3N4/BiVO4 Microflower Photocatalyst for Photocatalytic Degradation of Amoxicillin and Hydrogen Production, Malaysian Journal of Microscopy, 2020, 16, 180–187 Search PubMed.
- H. Jinshan, Z. Pengfei, C. Jifang, A. Weijia, L. Li, L. Yinghua, Y. Qingbin, Y. Hongjun and C. Wenquan, High-efficiency removal of phenol and coking wastewater via Photocatalysis-Fenton synergy over a Fe-g-C3N4 graphene hydrogel 3D structure, J. Ind. Eng. Chem., 2020, 84, 305–314 CrossRef.
- F. Jing, C. Tingting, L. Shenna, Z. Qihang, R. Yueming, L. Yanzhuo and F. Zhuangjun, Improvement of g-C3N4 photocatalytic properties using the Hummers method, J. Colloid Interface Sci., 2016, 479, 1–6 CrossRef.
- Y. Xingzhong, J. Longbo, L. Jie, P. Yang, Z. Jin, W. Hou, L. Lijian, W. Zhibin, G. Renpeng and Z. Guangming, In situ synthesis of 3D microsphere-like In2S3/InVO4 heterojunction with efficient photocatalytic activity for tetracycline degradation under visible light irradiation, Chem. Eng. J., 2019, 356, 371–381 CrossRef.
- H. Qiang, X. Ci'an, H. Yongming, C. Daimei, L. Yiwen, W. Wei and N. Bing-Jie, Accelerated separation of photogenerated charge carriers and enhanced photocatalytic performance of g-C3N4 by Bi2S3 nanoparticles, Chin. J. Catal., 2020, 41, 249–258 CrossRef.
- Z. Xue Song, H. Jian-Yang and J. Hong, Facile modification of a graphitic carbon nitride catalyst to improve its photoreactivity under visible light irradiation, Chem. Eng. J., 2014, 256, 230–237 CrossRef.
- Y. Min Quan, W. Bo and X. Yi-Jun, Synthesis of In2S3–CNT nanocomposites for selective reduction under visible light, J. Mater. Chem. A, 2013, 2, 1710–1720 Search PubMed.
- L. Jinze, M. Yue, Y. Zhefei, Z. Mingjun, W. Huiqin, M. Changchang, W. Dongdong, H. Pengwei and Y. Yongsheng, Fast electron transfer and enhanced visible light photocatalytic activity using multi-dimensional components of carbon quantum dots@3D daisy-like In2S3/single-wall carbon nanotubes, Appl. Catal., B, 2017, 204, 224–238 CrossRef.
- W. Yanyan, D. Xin, Z. Ping, W. Qi, Z. Kang, C. Lin, D. Jianjun, T. Xingyou and Z. Xian, Convenient and Recyclable TiO2/g-C3N4 Photocatalytic Coating: Layer-by-layer Self-assembly Construction on Cotton Fabrics Leading to Improved Catalytic Activity under Visible Light, Ind. Eng. Chem. Res., 2019, 58(10), 3978–3987 CrossRef.
- D. Guanglan, Z. Zhiliang, H. Qinghui, Z. Hua, Z. Jianyao, Q. Yanling, Y. Daqiang and Z. Jianfu, Targeted modulation of g-C3N4 photocatalytic performance for pharmaceutical pollutants in water using ZnFe-LDH derived mixed metal oxides: Structure-activity and mechanism, Sci. Total Environ., 2018, 650, 1112–1121 Search PubMed.
- P. Guiming, X. Lidan, B. Jesús, V. Michael and S. Menny, Frontispiz: A General Synthesis of Porous Carbon Nitride Films with Tunable Surface Area and Photophysical Properties, Angew. Chem., 2018, 130, 1200–1206 CrossRef.
- Y. Huang, W. Fan, B. Long, H. Li, F. Zhao, Z. Liu, Y. Tong and H. Ji, Visible light Bi2S3/Bi2O3/Bi2O2CO3 photocatalyst for effective degradation of organic pollutions, Appl. Catal., B, 2016, 185, 68–76 CrossRef CAS.
- L. Chao, W. Lili, L. Xiaogang, Z. Lu, L. Xuefang and S. Jinsheng, An efficient inverse opal (IO)-TiO2-MoO3−x for photocatalytic H2 evolution and RhB degradation – The synergy effect of IO structure and plasmonic MoO3−x, Appl. Surf. Sci., 2020, 527, 146726 CrossRef.
- L. Xiaoxiao, L. Qiang, L. Shihao, L. Rui, L. Hong, Z. Min, C. Chaopeng, Z. Guangping, C. San and L. Changhao, Fabrication of a novel BiOI/KTaO3 p–n heterostructure with enhanced photocatalytic performance under visible-light irradiation, RSC Adv., 2020, 10, 10921–10931 RSC.
- S. Zhangfeng, Z. Qiulin, Y. Chaochuang, K. Shifei, J. Hongyan, L. Xing, L. Xi, W. Yangang and C. Lifeng, Facile synthesis of 3D flower-like mesoporous Ce–ZnO at room temperature for the sunlight-driven photocatalytic degradations of RhB and phenol, J. Colloid Interface Sci., 2019, 556, 726–733 CrossRef.
- Y. Tingjiang, T. Jun, G. Wenfei, Q. Zheng, L. Wenjuan, Y. Jinmao and H. Baibiao, Ultra-low loading of Ag3PO4 on hierarchical In2S3 microspheres to improve the photocatalytic performance: the cocatalytic effect of Ag and Ag3PO4, Appl. Catal., B, 2017, 202, 84–94 CrossRef.
Footnote |
† Electronic supplementary information (ESI) available. See DOI: 10.1039/d1ra00729g |
|
This journal is © The Royal Society of Chemistry 2021 |