DOI:
10.1039/D1RA00565K
(Paper)
RSC Adv., 2021,
11, 12200-12208
Effects of an additive (hydroxyapatite–bentonite–biochar) on Cd and Pb stabilization and microbial community composition in contaminated vegetable soil†
Received
22nd January 2021
, Accepted 19th March 2021
First published on 26th March 2021
Abstract
A two-year pot experiment was conducted with a pimiento–celery cabbage (Capsicum annuum L.–Brassica pekinensis) rotation in acidic soil contaminated with Cd and Pb, which was amended with 0.0, 1.0, 2.5, 5.0 and 10.0% (w/w) premixtures of hydroxyapatite, bentonite and biochar combinations (HTB, in a ratio of 1
:
2
:
2). The results showed that the application of HTB at 2.5–10.0% significantly increased soil pH and organic carbon by an average of 10.38–17.60% and 35.60–55.34% during the two years, respectively. Compared to the control treatment, 1.0–10.0% HTB decreased the available Cd and Pb concentrations by 40.92–77.53% and 41.60–82.79% on average, respectively. In addition, the diversity and richness of the soil bacterial community improved after the two-year application of HTB. The relative abundances of Acidobacteria, Bacteroidetes and Chloroflexi increased under the HTB treatments, while those of Proteobacteria and Actinobacteria decreased. Redundancy analysis (RDA) and regression analysis indicated that soil pH and Cd and Pb availability were important factors shaping the soil bacterial community. The Cd and Pb concentrations in the edible parts of pimiento and celery cabbage decreased as the HTB application rate increased and met the Food Quality Standard in each season when the HTB application rate was 5.0% or higher. Higher rates of HTB (5.0% and 10.0%) not only ensured the quality of vegetables, but also significantly promoted pimiento and celery cabbage growth. Overall, these results indicated that the application of HTB, especially at a rate of 5.0%, could be an effective way to immobilize Cd and Pb, improve soil quality and ensure vegetables produced in acidic contaminated soil are safe for human consumption.
1. Introduction
Pollution from Cd and Pb in China's arable land has been worsening along with the development of the economy and the acceleration of industry in recent years.1 China is facing great challenges in efforts to decrease heavy metal pollution and improve the quality of agricultural soils. Cd and Pb can be easily taken up from soils by crops and accumulate in edible parts.2 High contents of Cd and Pb in crops directly or indirectly cause adverse effects on human health and may lead to consequences such as cancer, kidney dysfunction, haematological alterations, and disorders in trace mineral profiles.3,4 However, the biotoxicity of Cd and Pb is not directly linked to the total amounts in soils but is notably related to their availabilities.1 Cd2+ and Pb2+ are the predominant forms of Cd and Pb that are taken up by plants.5 Consequently, it is essential to reduce soil levels of available Cd and Pb to guarantee food safety with respect to Cd and Pb pollution in soils.
Many remediation techniques have been carried out, such as soil replacement, solidification, electrokinetic, chemical immobilization, washing and phytoremediation measures, to minimize the availability and mobility of heavy metals in agricultural soils.6–9 Some of these techniques are expensive, time-consuming, and environmentally unfriendly; therefore, they are unfeasible for large scale applications.10 Immobilization of heavy metals is still the most effective and widely used technique for in situ remediation. Many studies have demonstrated that biochar, bentonite and hydroxyapatite as amendments can effectively increase soil pH while significantly decreasing the contents of available heavy metals in soils.7,11,12 Specifically, our previous study reported that the combined use of hydroxyapatite, bentonite and biochar (HTB) was more effective for the immobilization of Cd and Pb than the application of the three amendments alone.13 However, a successful immobilization remediation technique must maintain reasonably low bioavailability of heavy metals and improve soil ecological function with universality and long-term effects.14
Soil microbes play an important role in ecosystem functions and they are especially susceptible and respond rapidly to changes in the soil environment.15 Soil microbial activity and microbial community composition are usually regarded as indexes to monitor and evaluate soil quality.16 Decreases in microbial activity and changes in microbial community composition may destabilize the soil ecosystem.17 The combined application of organic and inorganic amendments can decrease heavy metal pollution, resulting in changes in soil microbial activity and soil microbial community composition.16 Based on our preliminary experiments, the application of HTB can effectively increase the ability of soil microorganisms to utilize carbon sources in contaminated soil planted with vegetables.13 However, the microbial community composition may show different responses according to the type and concentration of heavy metals and amendments.6 Therefore, it is necessary to further understand the impact of HTB on changes in soil microbial community composition, which may facilitate the evaluation the effect and efficiency of chemical immobilization used in the mitigation of soil heavy metal contamination.
To date, detailed information about the changes in soil microbial composition and structure with HTB application has not been reported. A detailed study on how these amendments affect the distribution of soil microbial community composition is required. Moreover, there is minimal information available on the effects of continuous application of HTB on vegetables. In the current study, two-year pot experiments with continuous applications of HTB were carried out under a pimiento–celery cabbage rotation to investigate (i) the changes in soil pH, nutrient contents, available Cd and Pb, and soil microbial community properties after continuous application of HTB; (ii) the correlations among soil microbial community diversity and richness and the physicochemical characteristics of the tested soil; and (iii) the effects of HTB application on pimiento and celery cabbage Cd and Pb accumulation and growth.
2. Methods and materials
2.1. Soil characteristics and soil amendments
Surface soils (0–20 cm in depth) were collected from vegetable-planted land in the suburbs of Nanjing, Jiangsu Province, China. The soil was classified as Ferri-Udic Argosols based on Chinese soil taxonomy or Alfisol according to US soil taxonomy. The soil samples were air-dried and sieved through a 10-mesh sieve for use in pot experiments. The physicochemical characteristics of the test soil were as follows: sand 13.42%, silt 54.11% and clay 32.47%, pH 5.55, soil organic carbon (SOC) 27.51 g kg−1, available nitrogen, available phosphorus and available potassium contents of 107.5, 15.34 and 62.46 mg kg−1, respectively. The total amounts of Cd and Pb were 1.63 and 486.5 mg kg−1, respectively.
The soil additive HTB consisted of hydroxyapatite, thiol-functionalized bentonite, and biochar mixed at a ratio of 1
:
2
:
2 according to a previous study.18 Hydroxyapatite and bentonite were directly obtained from a local company in Nanjing, Jiangsu Province, China. The bentonite was modified to obtain thiol-functionalized bentonite, and the specific modification method was described in a previous study.13 The biochar was derived from rice straw that was prepared according to Zhang et al.18
2.2. Pot experiments
A pimiento–celery cabbage (Capsicum annuum L.–Brassica pekinensis) rotation system was used in a greenhouse pot experiment from April 2018 to November 2019. Pimiento was sown in mid-April and harvested in mid-August. Celery cabbage was then sown and harvested at the beginning of November. December to March of the following year was a fallow period. The pimientos and celery cabbages were planted at a density of two plants per pot. The pot experiments were conducted in a greenhouse; hence, tap water was added to replenish water based on normal vegetable growth requirements. Plastic pots, to which the treated and untreated soils were added, were 25 cm in diameter and 25 cm in height. Five HTB treatments (i.e., 0.0, 1.0, 2.5, 5.0 and 10.0% (w/w) of HTB) were tested. Each treatment was performed in triplicate and arranged in a randomized block design. In each pot, four kilograms of dried soil was added. Urea, calcium magnesium phosphate and potassium chloride were applied as basal fertilizers, and the application rate of N was 300 mg kg−1, and the application rates of P and K were 200 mg kg−1 and 300 mg kg−1 for pimiento, respectively. For celery cabbage, the application rates of N, P and K were 200 mg kg−1, 100 mg kg−1 and 200 mg kg−1, respectively. HTB amendment and fertilizers were applied to the soils and mixed thoroughly each season.
2.3. Sample collection and analysis
2.3.1 Vegetable samples. After harvest of pimiento and celery cabbage, all edible parts from each pot were washed with running water, followed by distilled water, and air-dried. Freshly prepared edible parts were weighed per pot. These materials were homogenized by pulp refiner and stored in plastic bottles prior to analysis. The Cd and Pb concentrations in the edible parts of the plants were determined using inductively coupled plasma mass spectrometry (ICP-MS) (Agilent 7500, USA) after digestion using HNO3/H2O2 according to the Determination of Cadmium in Foods, National Food Safety Standard of China (GB/T 5009.15-2014). The digestion process was as follows: 0.5000 g sample was weighed into a polytetrafluoroethylene inner tank, 4 mL of pure HNO3 was added for incubation overnight, and then 3 mL of H2O2 (30%) was added prior to digestion. The inner cover was closed, and the stainless steel coat was tightened. Then, the samples were placed into a constant temperature dryer and kept for 4–6 h at 140 °C. After that, the samples were placed onto an electric plate and digested to near dry. The samples were then diluted to 25 mL with HNO3 (1%) and stored at 4 °C before Cd and Pb analyses. A plant-certification reference material (GBW10015, National Research Center for Certified Reference Materials, China) was used to ensure the precision of the analytical procedure. The recovery rates of vegetable Cd and Pb ranged from 93% to 107%.
2.3.2 Soil samples. Soil samples (0–20 cm in depth) were collected from each pot after harvest of celery cabbage in 2018 and 2019. Each soil sample was air-dried and passed through 10-mesh and 100-mesh sieves for soil pH, SOC, available N, P, K, available Cd and Pb analyses. In particular, soil Cd and Pb distributions and microbial properties were analysed after the 2019 celery cabbage harvest. The specific methods for determination of soil pH, SOC, available N, P and K contents were described in the ESI.† Soil samples were extracted with diethylene triamine pentaacetic acid (DTPA) solution to determine soil available Cd and Pb contents.19 The DTPA extraction solution was instantly prepared as a mixture of 0.005 mol L−1 DTPA, 0.1 mol L−1 TEA (triethanolamine) and 0.01 mol L−1 CaCl2 and adjusted to pH 7.3 with HCl. Five grams of soil was mixed with 25 mL DTPA–TEA–CaCl2 solution and shaken on a reciprocating oscillator at 180 rpm at room temperature for 2 h. Afterwards, the mixtures were centrifuged, filtered and stored at 4 °C before Cd and Pb analyses. Changes in the speciation and distribution of Cd and Pb in soil were determined according to the European Community Bureau of Reference (BCR) sequential extraction method.20 Four different fractions designated by this method included the acid soluble, reducible, oxidizable, and residual fractions, of which the first two were extracted with 0.11 mol L−1 HAc and 0.5 mol L−1 NH2OH·HCl, respectively. After the second step, residual washed soil was digested with 30% (m/v) H2O2 and then extracted by 1.0 mol L−1 NH4OAc to obtain the oxidizable fraction. The residual fraction was obtained by digesting the residue with a HNO3–HF–HClO4 mixture. The detailed procedures were presented in the ESI.† The Cd and Pb concentrations in the filtrates of the aforementioned sequential extraction and DTPA extraction were measured by ICP-MS (Agilent 7500, USA). A certification reference material (GBW07412, National Research Center for Certified Reference Materials, China) was used to ensure the precision of the analytical procedure. The recovery rates of soil Cd and Pb ranged from 95% to 106%.
2.4. Soil DNA extraction, PCR amplification and sequencing
Fresh soil samples (0.25 g) from each pot were used to extract soil DNA with the Fast DNA® Spin Kit (MP Biomedicals, Santa Ana, CA, USA). The V4 hypervariable regions of 16S rRNA genes were amplified with the universal primers 515F (5′-GTGCCAGCMGCCGCGGTAA-3′) and 806R (5′-GGACTACVVGGGTAT CTAATC-3′) using a 12 nt unique barcode. PCR (polymerase chain reaction) was carried out in a Gene Amp PCR System 9700 thermal cycler (Applied Biosystems Inc., Foster City, CA, USA) with an initial DNA denaturation step at 95 °C for 3 min, followed by 30 cycles of denaturation at 94 °C for 30 s, annealing at 56 °C for 45 s, and extension at 72 °C for 60 s, and a final extension step at 72 °C for 10 min.21 The AxyPrep DNA gel extraction kit was used to purify the PCR products before sequencing by the Illumina MiSeq platform (Sangon Biotechnology Co., Ltd, Shanghai, China).
Data were analysed per procedure according to Fierer et al.22 using the Quantitative Insights into Microbial Ecology (QIIME) pipeline.23 Sequence reads were removed from the dataset if their average quality score was <30 and read length was <250 bp. Sequences were clustered and assigned into operational taxonomic units (OTUs) using QIIME's pair-wise identity threshold at 97%. Singleton OTUs were screened, representative sequences were used to classify the OTUs at the lowest possible taxonomic level, and those OTUs were used for calculation of Chao1, Shannon and Simpson indexes.
2.5. Statistical analyses
The means (n = 3) and standard deviations (SD) of the soil physicochemical properties, soil available Cd and Pb, soil bacterial community diversity and richness, and availability of Cd and Pb for plant uptake were tabulated and presented. Statistical analysis of variance (ANOVA) was conducted using Statistical Package for the Social Sciences (SPSS) software (version 20.0 for Windows). The least significant difference (LSD) test at a 5% probability level was employed. The possible correlations among the soil microbial community and soil physicochemical properties were tested using redundancy analysis (RDA) (CANOCO 4.5).
3. Results and discussion
3.1. Effects of HTB on soil physicochemical properties and heavy metal availability
The changes in soil physicochemical properties following different HTB treatments are shown in Table 1. Soil pH was notably higher with an HTB application rate >1.0% than in the control group (p < 0.05) during the two years. Moreover, soil pH increased dramatically with increasing application rates of HTB. Compared to 2018, soil pH increased under all HTB treatments in 2019; the increments were 0.06, 0.15, 0.12 and 0.07, under 1.0, 2.5, 5.0 and 10.0% HTB amendments, respectively. The results indicated that the application of alkaline materials can effectively increase acidic soil pH, but continuous high inputs (>5.0%) may gradually reduce this increase. This may be related to the buffering actions of soil. There is a higher buffer capacity of soil in 5.0% and 10.0% HTB treatments, which can reduce the effect of released alkaline substances on soil pH and maintain soil pH within a range to avoid strong changes. Soil organic carbon (SOC) and available nutrients (N, P, K) are important indicators of soil quality. Generally, there were no significant changes in SOC and available nutrients (N, P, K) between the control group and the low input (1%) HTB group in 2018. SOC was notably altered (in comparison with the control group) by moderate input (2.5%) of HTB in 2019, while available N, P and K were significantly altered under all HTB treatments. However, there was no marked increase when the application rates of HTB exceeded 5.0% during the two years. Previous studies have indicated that application of biochar can significantly improve soil nutrients, which may be ascribed to the direct supplementation of these nutrients by biochar.24,25 Moreover, hydroxyapatite can accelerate the increase in soil phosphorus. Additionally, crop roots remained in the soil after harvest of the vegetables, resulting in an increase in organic carbon.
Table 1 Main soil physicochemical properties in the control and amended soilsa
Treatment (%) |
pH (1 : 2.5) |
SOC (g kg−1) |
N-available (mg kg−1) |
P-available (mg kg−1) |
K-available (mg kg−1) |
Values are presented as mean ± standard deviation. Different lowercase letters indicate significant difference among treatments at p < 0.05 (n = 3, LSD test). SOC: soil organic carbon; N-available: available nitrogen concentration; P-available: available phosphorus concentration; K-available: available potassium concentration. |
2018 year |
0 |
5.55 ± 0.03d |
27.66 ± 2.61b |
96.24 ± 5.68c |
12.78 ± 2.02c |
59.45 ± 7.58d |
1.0 |
5.62 ± 0.03d |
29.78 ± 1.85b |
95.28 ± 6.89c |
14.51 ± 2.17c |
64.17 ± 5.39cd |
2.5 |
6.04 ± 0.06c |
34.64 ± 2.54b |
103.5 ± 7.42bc |
19.63 ± 2.29b |
73.08 ± 6.21bc |
5.0 |
6.37 ± 0.07b |
37.19 ± 1.89a |
119.3 ± 10.65ab |
20.29 ± 1.95ab |
81.89 ± 5.83ab |
10.0 |
6.48 ± 0.04a |
39.57 ± 2.73a |
126.7 ± 13.28a |
24.56 ± 3.43a |
84.42 ± 7.04a |
2019 year |
0 |
5.53 ± 0.03e |
26.59 ± 2.18b |
78.29 ± 2.82d |
9.13 ± 1.04d |
49.53 ± 2.48d |
1.0 |
5.68 ± 0.04d |
31.25 ± 2.65b |
98.89 ± 5.16c |
15.22 ± 1.49c |
62.05 ± 3.39c |
2.5 |
6.19 ± 0.05c |
38.81 ± 2.98a |
111.3 ± 8.53bc |
22.41 ± 2.07b |
78.17 ± 3.07b |
5.0 |
6.49 ± 0.04b |
39.07 ± 2.74a |
124.8 ± 10.85ab |
26.48 ± 2.35a |
89.72 ± 4.83a |
10.0 |
6.55 ± 0.03a |
44.57 ± 3.71a |
139.6 ± 12.14a |
29.81 ± 2.99a |
94.79 ± 5.04a |
Soil available Cd and Pb were affected by the amount of HTB applied, but there was little change from 2018 to 2019 (Fig. 1). Compared to the control, significant decreases in available Cd and Pb were found with HTB application; the available Cd concentrations decreased 40.32–75.81% and 41.51–79.25% in the HTB-treated soils in 2018 and 2019, respectively. The available Pb concentrations were reduced by 39.65–82.62% and 43.55–82.96% in 2018 and 2019, respectively. Specifically, no marked difference was identified between the 5.0% and 10.0% HTB treatments. Soil available Cd and Pb concentrations sharply decreased, attributed in part to the increase in soil pH after HTB application, which may play the most vital role in the stabilization of soil Cd and Pb. Previous studies demonstrated that soil available heavy metal concentrations are negatively correlated to soil pH.26,27 A higher soil pH may promote the adsorption of positively charged heavy metal ions by soil colloids, increase the binding of ions to OH− groups and promote Cd precipitation as CdCO3 or Pb precipitation as Pb5(PO4)3OH and Pb(OH)2, resulting in a decrease in soil heavy metal mobility and availability.28,29 In addition, decreasing Pb availability may partly be caused by ionic exchange of hydroxyapatite; for Cd, isomorphic substitution with Ca2+ appears more significant than precipitation, and this process may be ascribed to the ionic radius of Cd2+ being very close to that of Ca2+.5,30 In addition, bentonite can exchange and adsorb a large amount of Cd2+ and Pb2+, and the organic matter in biochar promotes an increase in organically bound Cd and Pb in the soil.31,32 Another mechanism of Cd and Pb reduction may be the surface complexation of Cd and Pb via interaction with the functional groups on the surface of biochar,33,34 which also has important roles in decreasing Cd and Pb availability. An important process for Cd and Pb immobilization and mobility is the transformation of different forms in soils after the application of HTB. Sequential extraction has provided some evidence for the redistribution of Cd and Pb.35,36 The application of HTB changed the Cd and Pb distributions in the tested soil (Fig. 2). The acid solubility of Cd was decreased by 16.31–52.65% following the various HTB treatments compared with that under the control, and the residual amount of Cd in HTB-treated soils was 0.97–2.95 times higher than that in untreated soil. The results indicated that the application of HTB significantly changed Cd from an acid-soluble form to a more stable residual form. For Pb, HTB treatments dramatically increased the residual fraction of Pb by 51.49–84.92% compared to the control. Unlike Cd, the increase in the residual fraction of Pb was caused by the decrease in acid-soluble and reducible fractions of Pb. One reason was that the reducible fraction of Pb was the dominant fraction in the tested soil, and the application of HTB altered the soil conditions and facilitated the activation of Pb bound to Fe/Mn oxides, resulting in a decrease in the reducible fractions of Pb.13 On the other hand, hydroxyapatite could promote the formation of more stable complexes, mineral lattices, and precipitation with Pb, for example, hydroxyl-pyromorphite, which might be responsible for the transformation of the reducible fraction of Pb to the residual fraction.37 Moreover, the application of biochar increased soil organic matter, producing organic ligands and bind Pb ions to form stable complexes.7 A previous study demonstrated that there was a significant negative correlation between soil reducible fractions of Pb and organic matter.38 These results provide strong evidence to demonstrate that the risk associated with the availability and mobility of soil Cd and Pb declined after the application of HTB.
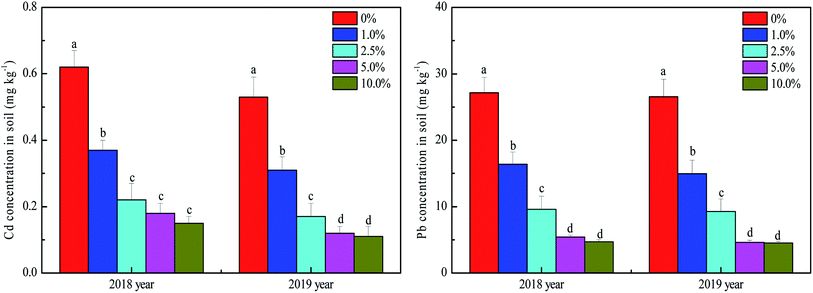 |
| Fig. 1 Available concentrations of Cd and Pb in soil amended with different application rates of HTB. Values are the mean ± SD, and different lowercase letters between bars indicate significant differences at p < 0.05 level (n = 3, LSD test). | |
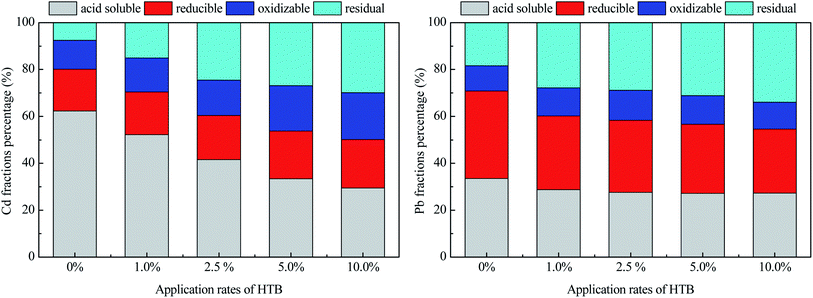 |
| Fig. 2 Proportions of Cd and Pb in different fractions following sequential extraction under different application rates of HTB. | |
3.2. Effects of HTB on soil microbial properties
3.2.1 Soil bacterial community diversity and richness. A total of 141
592 high-quality reads from 5 representative soil samples were obtained according to the sequencing-based analysis (Table 2). The same total number of sequences per sample (i.e., 26
846) was used and analysed to compare the richness and diversity of the soil bacterial community among all treatments. The coverage values of all treatments were more than 97%, and the highest value was observed in the 2.5% HTB amendment treatment. After the application of HTB, the average number of observed OTUs increased from 1763 in the control group to 2100 in the 10.0% HTB treatment. Soil bacterial community diversity and richness indexes, including Shannon, Simpson, Chao1 and ACE indexes, were compared among different HTB-treated soils. The values for Shannon's diversity and Chao1 richness in soils treated with 5.0% and 10.0% HTB-amended were higher than those in any other HTB-amended soil, and no difference was detected between the 5.0% and 10.0% HTB amendment treatments. The diversity and richness of soil bacterial community in the control and 1.0% HTB treatments were lower than those in the other three amendment treatments (Table 2). These results showed that an appropriate rate of HTB application was an effective way to increase soil bacterial community diversity and richness. The primary factors behind these increases may include higher soil pH (Table 1) and lower Cd and Pb availability (or both) (Fig. 1) under the HTB treatments. Regression analysis indicated that soil pH and SOC had pronounced positive correlations with richness (ACE, Chao1 and OTUs) and diversity (Shannon) indexes (p < 0.05), while a marked negative correlation (p < 0.05) was observed between bacterial community richness (OTUs, Chao1 and ACE) and available Cd or Pb concentration (Table 3). These results are consistent with findings by other researchers. Kim et al. demonstrated that soil pH was a key edaphic factor affecting the bacterial community diversity of greenhouse soils.39 Chodak et al.40 and Huang et al.41 indicated that high concentrations of heavy metals restrained the increase in diversity indexes. Shi et al.42 also found that higher soil pH and organic matter may cause marked differences in microbial community diversity. Therefore, we can infer that soil pH and heavy metal and SOC concentrations were the main driving factors that changed bacterial community richness and diversity in this study.
Table 2 Microbial community diversity and richness in tested soil amended with different application rates of HTBa
Treatment (%) |
Reads |
Coverage |
Observed OTUs |
Shannon |
Simpson |
Chao1 |
ACE |
Values are presented as mean ± standard deviation. |
0 |
26 846 ± 1325 |
0.9811 ± 0.0112 |
1763 ± 103 |
5.28 ± 0.08 |
0.9677 ± 0.0005 |
2272 ± 206 |
2291 ± 184 |
1.0 |
28 638 ± 1782 |
0.9798 ± 0.0123 |
1781 ± 115 |
5.53 ± 0.07 |
0.9648 ± 0.0007 |
2316 ± 175 |
2297 ± 201 |
2.5 |
30 062 ± 1649 |
0.9823 ± 0.0208 |
2039 ± 145 |
5.90 ± 0.11 |
0.9559 ± 0.0013 |
2628 ± 248 |
2605 ± 179 |
5.0 |
28 381 ± 1536 |
0.9749 ± 0.0157 |
2067 ± 137 |
5.97 ± 0.16 |
0.9591 ± 0.0011 |
2728 ± 181 |
2693 ± 225 |
10.0 |
27 665 ± 1860 |
0.9765 ± 0.0129 |
2100 ± 152 |
6.02 ± 0.09 |
0.9553 ± 0.0015 |
2733 ± 236 |
2708 ± 256 |
Table 3 Pearson correlation coefficients between soil physicochemical indexes and soil bacterial diversity indexesa
|
pH |
SOC |
N-available |
P-available |
K-available |
Cd-available |
Pb-available |
SOC: soil organic carbon; N-available: available nitrogen concentration; P-available: available phosphorus concentration; K-available: available potassium concentration; Cd-available: available cadmium concentration; Pb-available: available lead concentration. * Correlation is significant at the 0.05 level. |
OTUs |
0.821* |
0.729* |
0.652* |
0.575 |
0.306 |
−0.825* |
−0.653* |
Shannon |
0.703* |
0.694* |
0.183 |
0.204 |
0.175 |
−0.527 |
−0.412 |
Simpson |
−0.568 |
−0.307 |
−0.198 |
−0.225 |
−0.273 |
−0.344 |
−0.426 |
Chao1 |
0.715* |
0.898* |
0.572 |
0.635 |
0.254 |
−0.787* |
−0.647* |
ACE |
0.687* |
0.859* |
0.476 |
0.614 |
0.192 |
−0.823* |
−0.704* |
3.2.2 Shift in the soil bacterial community composition. HTB amendments modified the soil bacterial community composition, although a noticeable difference was not identified in the bacterial community composition between the control and various treatments, the relative abundance of phyla differed among the five treatments. Among the 15 phyla, the predominant phyla were Proteobacteria, Acidobacteria, Bacteroidetes, Actinobacteria and Chloroflexi, accounting for 81.45% of the total bacterial count (Fig. 3A). Proteobacteria accounted for 26.08–41.45% (i.e., minimum of 26.08% in the 1.0% HTB treatment and maximum of 41.45% in the control group) of the total relative abundance, followed by Acidobacteria (i.e., minimum of 16.91% in the control group and maximum of 23.67% in the 5.0% HTB treatment). The relative abundance of Bacteroidetes increased after HTB application, from 15.60% in the control to 22.56% in the 10.0% HTB amendment.
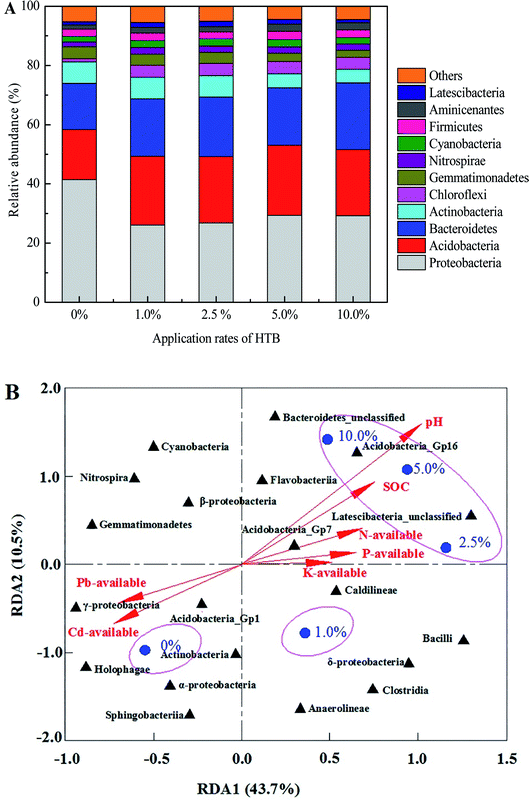 |
| Fig. 3 Effects of different application rates of HTB on soil bacterial community composition. (A) The relative abundance of the dominant soil bacterial phyla. (B) The correlation among the most abundant classes, soil physicochemical characteristics and soil treatments by redundancy analysis (RDA). SOC: soil organic carbon; N-available: available nitrogen concentration; P-available: available phosphorus concentration; K-available: available potassium concentration; Cd-available: available cadmium concentration; Pb-available: available lead concentration. | |
Proteobacteria, Acidobacteria, Bacteroidetes and Actinobacteria were the most dominant phyla in the control and HTB-treated soils, which is consistent with other observations.43 The possible reason may be that these phyla have the highest abundance of Cd–Pb resistance genes and can produce some extracellular polymeric substance, leading to highly tolerant to Cd and Pb contamination.44 The relative abundances of Proteobacteria and Actinobacteria decreased in the HTB-treated soil compared with those of the control, which may be ascribed to the higher pH caused by the amendment treatments. Similarly, Huang et al. indicated that unamended heavy metal-contaminated soil showed an increase in Proteobacteria and Actinobacteria compared to amended soil.41 However, some researchers also indicated that the relative abundance of Proteobacteria increased with pH in paddy soil.42 This may be the result of different responses between soil pH and the relative abundance of Proteobacteria. We also found that the relative abundances of Acidobacteria and Bacteroidetes were improved in the HTB treatments, which was probably caused by the higher nutrient contents, soil pH and lower Cd and Pb concentrations; this result was also reported by Kielak et al.45
3.2.3 Relationships between bacterial community composition and soil physicochemical properties. Soil microorganisms are very sensitive to environmental disturbance; in particular, they are affected by soil physical and chemical properties. Redundancy analysis (RDA) was carried out to explore the link between soil physicochemical properties and bacterial community composition (Fig. 3B). The results demonstrated that statistical significance was found on the two axes (p < 0.05). The first and second axes explained 43.7% and 10.5% of the variation, respectively. The HTB-amended soils were distinguished from the control group by the first axis, which was positively related to soil pH, SOC, available N, P and K. The control treatment was a separate group and related to soil available Cd and Pb, according to the bacterial community analysis. According to the regression analysis (Table S1†), we can further infer that soil pH, SOC, Cd and Pb availability had significant effects on bacterial community composition (p < 0.05). Soil microbes such as α-Proteobacteria, γ-Proteobacteria and Actinobacteria were negatively correlated with pH (p < 0.05) and positively correlated with the available Cd and Pb contents (p < 0.05). Acidobacteria_Gp7, Acidobacteria_Gp16 and Flavobacteria were significantly positively correlated with pH and SOC (p < 0.05) and negatively correlated with the available Cd and Pb contents (p < 0.05). The results also showed that soil pH was the strongest factor among the soil physicochemical characteristics, which is in accordance with Ghosh et al.46 Therefore, HTB application could increase soil pH and decrease Cd and Pb concentrations, resulting in the growth of soil bacteria and a change in relative abundances in the bacterial community.
3.3. Effects of HTB on vegetable quality and yield
Heavy metal bioavailability in the edible parts of crops and crop yield are both important indicators for assessing the efficiency of in situ immobilization.47,48 In this study, Cd and Pb concentrations (fresh weight) in edible parts of pimiento and celery cabbage were significantly reduced with HTB application compared to the control during the two years (p < 0.05) (Fig. 4). The Cd concentrations in pimiento and celery cabbage met the maximum limits (i.e., 0.05 mg kg−1 and 0.2 mg kg−1, respectively) listed in the Chinese food quality standard (GB2762-2017) when the HTB application rate was 2.5–10.0%. The Pb concentrations under the 5.0% and 10.0% HTB treatments were below the maximum limits (i.e., 0.1 mg kg−1 for pimiento and 0.3 mg kg−1 celery cabbage). Therefore, pimiento and celery cabbage can be considered safe when the application rate of HTB is at 5.0% or above. HTB amendment can effectively decrease the accumulation of Cd and Pb in pimiento and celery cabbage due to the stabilization of Cd and Pb in the tested soil (Fig. 1). Previous studies also demonstrated that the soil availability of heavy metals is a reliable indicator for evaluating the phytoavailability of heavy metals.14,49
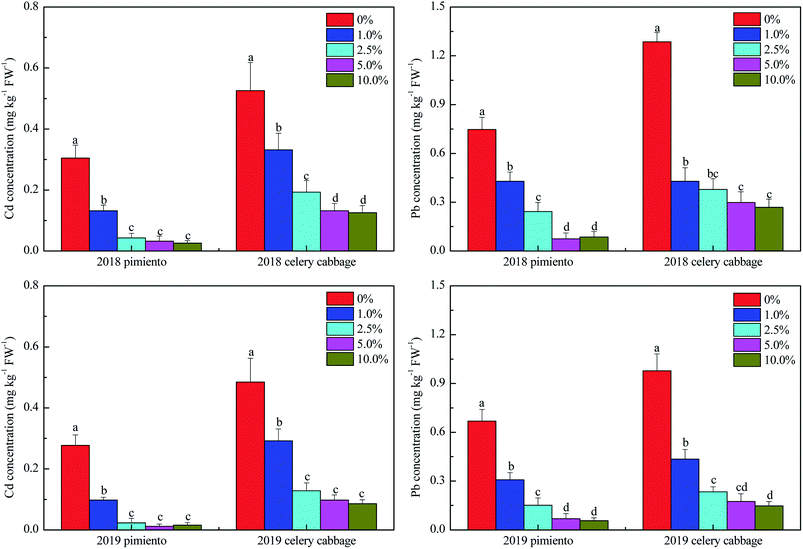 |
| Fig. 4 Concentrations of Cd and Pb in the edible parts of pimiento and celery cabbage under different application rates of HTB. The Cd and Pb concentrations in vegetables were based on fresh weight. Values are the mean ± SD, and different lowercase letters between bars indicate significant differences at p < 0.05 level (n = 3, LSD test). | |
A higher application rate of HTB (i.e., 5.0% and 10.0%) yielded significantly higher biomasses for pimiento and celery cabbage than those of the control (Fig. S1†). The reason for the increase in yield was that HTB decreased Cd and Pb accumulation in vegetables, and thus helped reduce Cd and Pb stress. Another reason was that HTB applications can effectively increase soil organic carbon and available nutrients (Table 1) and thereby enhance soil fertility. Moreover, when the application rate of HTB was 5.0% or 10.0%, the microbial community diversity and richness were much higher than those under the other treatments (Table 2). These factors were beneficial for improving soil quality to promote pimiento and celery cabbage growth.
4. Conclusions
The application of HTB increased soil pH, nutrient contents as well as alleviated Cd and Pb availability in soil and vegetables. In particular, higher rates of HTB (5.0% and 10.0%) not only ensured the quality of vegetables but also significantly promoted vegetable growth. The results also preliminarily indicated that HTB amendments modified the soil bacterial community composition, and soil pH and heavy metal availability were important factors shaping the soil bacterial community. Future studies are necessary to focus on the stabilization of Cd and Pb after HTB application under field conditions, to confirm these findings.
Conflicts of interest
The authors declare that they have no conflicts of interest.
Acknowledgements
This research was supported by the Natural Science Foundation of the Jiangsu Higher Education Institutions of China (19KJB610005) and the Scientific Research Project of Nanjing Xiaozhuang University (2018NXY52).
References
- J. F. Gu, H. Zhou, H. L. Tang, W. T. Yang, M. Zeng, Z. M. Liu, P. Q. Peng and B. H. Liao, Ecotoxicol. Environ. Saf., 2019, 171, 451–459 CrossRef CAS PubMed.
- L. Zeng, X. Lin, F. Zhou, J. Qin and H. Li, Ecotoxicol. Environ. Saf., 2019, 167, 520–530 CrossRef CAS PubMed.
- A. G. Boim, L. C. Melo, F. N. Moreno and L. R. Alleoni, J. Environ. Manage., 2016, 170, 21–27 CrossRef CAS PubMed.
- S. Moitra, J. Ghosh, J. Firdous, A. Bandyopadhyay, M. Mondal, J. K. Biswas, S. Sahu, S. Bhattacharyya and S. Moitra, Toxicol. Ind. Health, 2018, 34, 908–921 CrossRef CAS PubMed.
- Z. Rahman and V. P. Singh, Environ. Monit. Assess., 2019, 191, 419 CrossRef PubMed.
- J. Cheng, Y. Li, W. Gao, Y. Chen, W. Pan, X. Lee and Y. Tang, Biol. Fertil. Soils, 2018, 54, 373–383 CrossRef CAS.
- D. Y. He, J. Cui, M. Gao, W. Q. Wang, J. Zhou, J. Yang, J. J. Wang, Y. Li, C. S. Jiang and Y. Peng, Sci. Total Environ., 2019, 654, 1364–1371 CrossRef CAS PubMed.
- V. Suthar, K. S. Memon and M. Mahmood-ul-Hassan, Environ. Monit. Assess., 2014, 186, 3957–3968 CrossRef CAS PubMed.
- D. Zhang, A. F. Ding, T. Li and X. X. Wu, Pol. J. Environ. Stud., 2021, 30, 1903–1912 CrossRef.
- D. Houben, L. Evrard and P. Sonnet, Chemosphere, 2013, 92, 1450–1457 CrossRef CAS PubMed.
- C. Liu, L. Wang, J. Yin, L. Qi and Y. Feng, Bull. Environ. Contam. Toxicol., 2018, 100, 581–587 CrossRef CAS PubMed.
- R. J. Sun, J. H. Chen, T. T. Fan, D. M. Zhou and Y. J. Wang, Environ. Sci. Pollut. Res. Int., 2018, 25, 73–80 CrossRef CAS PubMed.
- D. Zhang and A. Ding, Bull. Environ. Contam. Toxicol., 2019, 103, 98–105 CrossRef CAS PubMed.
- F. Y. Guo, C. F. Ding, Z. G. Zhou, G. Y. Huang and X. X. Wang, Ecotoxicol. Environ. Saf., 2018, 148, 303–310 CrossRef CAS PubMed.
- D. Zhang, X. G. Li and X. X. Wang, Int. J. Agric. Biol., 2015, 17, 929–936 CrossRef CAS.
- K. Xue, J. Zhou, J. Van Nostrand, M. Mench, C. Bes, L. Giagnoni and G. Renella, Environ. Pollut., 2018, 242, 229–238 CrossRef CAS PubMed.
- X. Y. Xiao, M. W. Wang, H. W. Zhu, Z. H. Guo, X. Q. Han and P. Zeng, Ecotoxicol. Environ. Saf., 2017, 142, 200–206 CrossRef CAS PubMed.
- D. Zhang and A. F. Ding, J. Agro-Environ. Sci., 2018, 37, 2718–2726 Search PubMed.
- D. Luo, H. Zheng, Y. Chen, G. Wang and F. Ding, J. Environ. Manage., 2010, 91, 2248–2253 CrossRef CAS PubMed.
- M. S. Rizwan, M. Imtiaz, M. A. Chhajro, G. Huang, Q. Fu, J. Zhu, O. Aziz and H. Hu, Environ. Technol., 2016, 37, 2679–2686 CrossRef CAS PubMed.
- X. G. Li, C. F. Ding, T. L. Zhang and X. X. Wang, Soil Biol. Biochem., 2014, 72, 11–18 CrossRef CAS.
- N. Fierer, M. Hamady, C. L. Lauber and R. B. Jackson, Proc. Natl. Acad. Sci. U.S.A, 2008, 105, 17994–17999 CrossRef CAS PubMed.
- J. G. Caporaso, J. Kuczynski, J. Stombaugh, K. Bittinger, F. D. Bushman and E. K. Costello, Nat. Methods, 2010, 7, 335 CrossRef CAS PubMed.
- A. Enders, K. Hanley, T. Whitman, S. Joseph and J. Lehmann, Bioresour. Technol., 2012, 114, 644–653 CrossRef CAS PubMed.
- F. Liang, G. T. Li, Q. M. Lin and X. R. Zhao, J. Integr. Agric., 2014, 13, 525–532 CrossRef CAS.
- Y. Liu, Y. Wang, H. Lu, L. Lonappan, S. K. Brar, L. He, J. Chen and S. Yang, J. Soils Sediments, 2018, 18, 2511–2519 CrossRef CAS.
- X. Yang, J. J. Liu, K. McGrouther, H. G. Huang, K. P. Lu, X. Guo, L. Z. He, X. M. Lin, L. Che, Z. Q. Ye and H. L. Wang, Environ. Sci. Pollut. Res. Int., 2016, 23, 974–984 CrossRef CAS PubMed.
- X. Cao, L. Ma, Y. Liang, B. Gao and W. Harris, Environ. Sci. Technol., 2011, 45, 4884–4889 CrossRef CAS PubMed.
- Y. Xue, B. Gao, Y. Yao, M. Inyang, M. Zhang, A. R. Zimmerman and K. S. Ro, Chem. Eng. J., 2012, 200–202, 673–680 CrossRef CAS.
- Z. Yang, L. Liang, W. Yang, W. Shi, Y. Tong, L. Chai, S. Gao and Q. Liao, Environ. Sci. Pollut. Res. Int., 2018, 25, 11970–11980 CrossRef CAS PubMed.
- W. Y. Shi, H. Li, S. Du, K. B. Wang and H. B. Shao, Appl. Clay Sci., 2013, 85, 103–108 CrossRef CAS.
- A. H. Fahmi, A. W. Samsuri, H. Jol and D. Singh, R. Soc. Open Sci., 2018, 5, 181328 CrossRef CAS PubMed.
- S. Bashir, J. Zhu, Q. Fu and H. Hu, Environ. Sci. Pollut. Res. Int., 2018, 25, 11875–11883 CrossRef CAS PubMed.
- T. Y. Jiang, J. Jiang, R. K. Xu and Z. Li, Chemosphere, 2012, 89, 249–256 CrossRef CAS PubMed.
- J. F. Gu, H. Zhou, W. T. Yang, P. Q. Peng, P. Zhang, M. Zeng and B. H. Liao, Environ. Sci. Pollut. Res. Int., 2018, 25, 8608–8619 CrossRef CAS PubMed.
- J. Liang, Z. X. Yang, L. Tang, G. M. Zeng, M. Yu, X. D. Li, H. P. Wu, Y. Y. Qian, X. M. Li and Y. A. Luo, Chemosphere, 2017, 181, 281–288 CrossRef CAS PubMed.
- Y. F. Wang, R. Z. Li, W. Z. Liu, L. Cheng, Q. Jiang and Y. Zhang, Environ. Sci. Pollut. Res. Int., 2019, 26, 26674–26684 CrossRef CAS PubMed.
- C. Xu, H. X. Chen, Q. Xiang, H. H. Zhu, S. Wang, Q. H. Zhu, D. Y. Huang and Y. Z. Zhang, Environ. Sci. Pollut. Res., 2018, 25, 1147–1156 CrossRef CAS PubMed.
- J. M. Kim, A. S. Roh, S. C. Choi, E. J. Kim, M. T. Choi, B. K. Ahn, S. K. Kim, Y. H. Lee, J. H. Joa, S. S. Kang, S. A. Lee, J. H. Ahn, J. Song and H. Y. Weon, J. Microbiol., 2016, 54, 838–845 CrossRef CAS PubMed.
- M. Chodak, M. Gołębiewski, J. Morawska-Płoskonka, K. Kuduk and M. Niklińska, Appl. Soil Ecol., 2013, 64, 7–14 CrossRef.
- L. M. Huang, G. W. Yu, F. Z. Zou, X. X. Long and Q. T. Wu, Appl. Soil Ecol., 2018, 130, 104–119 CrossRef.
- L. Shi, Z. Guo, C. Peng, X. Xiao, W. Feng, B. Huang and H. Ran, Ecotoxicol. Environ. Saf., 2019, 171, 425–434 CrossRef CAS PubMed.
- H. Kaplan, S. Ratering, T. Hanauer, P. Felix-Henningsen and S. Schnell, Biol. Fertil. Soils, 2013, 50, 735–744 CrossRef.
- Y. Chen, Y. M. Jiang, H. Y. Huang, L. C. Mou, J. L. Ru, J. H. Zhao and S. Xiao, Sci. Total Environ., 2018, 637–638, 1400–1412 CrossRef CAS PubMed.
- A. M. Kielak, C. C. Barreto, G. A. Kowalchuk, J. A. van Veen and E. E. Kuramae, Front. Microbiol., 2016, 7, 744 Search PubMed.
- S. Ghosh, M. Moitra, C. J. Woolverton and L. G. Leff, Can. J. Microbiol., 2012, 58, 1316–1326 CrossRef CAS PubMed.
- Y. Sun, Y. Xu, Y. Xu, L. Wang, X. Liang and Y. Li, Environ. Pollut., 2016, 208, 739–746 CrossRef CAS PubMed.
- F. J. Zhao, Y. Ma, Y. G. Zhu, Z. Tang and S. P. McGrath, Environ. Sci. Technol., 2015, 49, 750–759 CrossRef CAS PubMed.
- L. M. Huang, G. W. Yu, X. Cai and X. X. Long, Int. J. Environ. Res., 2017, 11, 425–437 CrossRef CAS.
Footnote |
† Electronic supplementary information (ESI) available. See DOI: 10.1039/d1ra00565k |
|
This journal is © The Royal Society of Chemistry 2021 |