DOI:
10.1039/D1RA00415H
(Review Article)
RSC Adv., 2021,
11, 6517-6525
New advances on Au–magnetic organic hybrid core–shells in MRI, CT imaging, and drug delivery
Received
17th January 2021
, Accepted 20th January 2021
First published on 5th February 2021
Abstract
Magnetic nanoparticles have been widely studied for various scientific and technological applications such as magnetic storage media, contrast agents for magnetic resonance imaging (MRI), biolabelling, separation of biomolecules, and magnetic-targeted drug delivery. A new strategy on Au–magnetic nano-hybrid core–shells was applied in MRI, CT imaging, and drug delivery, which has been received much attention nowadays. Herein, the designing of different magnetic core–shells with Au in MRI and cancer treatment is studied.
1. Introduction
There are various nanoparticles for medical applications, including carbon dots1,2 and organic core/shell nanoparticles. Among all these materials, Fe3O4 (ref. 3) and MnFe2O4 (ref. 4) gain the first step in terms of usage. However, TiO2,5 SnO2,6 Ag,7 Au,8 S,9 BaTiO3,10 and ZnO11 are known for their electrical applications. Through the coating process, various materials such as polyaniline (PA),12 polystyrene (PS), graphene,6 oleic acid, polyvinylpyrrolidone (PVP), hyperbranched aromatic polyamide (HBP),10 poly(methylmethacrylate) (PMMA), are differently used, and the provided materials are used extensively for batteries, optical sensors, and magnetic imaging. The metal–organic frameworks (MOFs) have received much attention in terms of bioimaging in living cells, as porous functional materials, which have large surface areas, high porosity, fluorescence functionalities, and good biocompatibility. Organic or inorganic fluorescent materials such as fluorescent dyes, quantum dots, and metal nanoclusters can also be applied in medicine for bioimaging.
The importance of magnetite nanoparticles (Fe3O4 NPS) was extensively studied due to their many biomedical uses in cancer cells, magnetic resonance imaging (MRI) as a contrast agent, drug delivery, and hyperthermia treatment. In terms of toxicity and susceptibility, magnetite NPs are safe and have superparamagnetic applications.13–16 For improving the stability and dispersing the NPs in water, Fe3O4 NP was coated with Au NPs to improve the stability and dispersion of the NPs in aqueous media as efficient materials due to their biocompatibility, stability, resistance, which provided the potential medical requests.17–20 Gold nanoparticles (AuNPs) have received much attention because of their unique physicochemical activities such as biological, chemical, and biomedical implementations. AuNPs have been used for sensing, catalysis, imaging and diagnosis, and therapy (Fig. 1).21
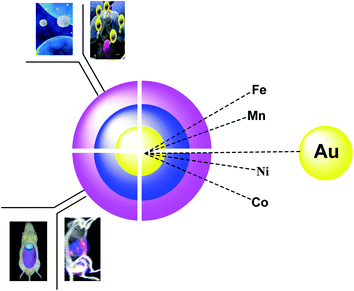 |
| Fig. 1 The application of the different nanoparticles as core–shells. | |
Core–shell (CS) nanostructures have received much attention due to the known constituents and configuration.22 In this area, the immunization of Au on Fe3O4@ promoted the optical properties of computed tomography (CT) and MRI, which increased the resolution in 3D visual images in this process.23,24 Through the importance of bioimaging in living cells, metal–organic frameworks (MOFs) were used which organic or inorganic fluorescent compounds like quantum dots, fluorescent dyes, nanosheets, and metal nanoclusters can provide modified MOFs to give fluorescent nano-composites for an imaging agent.25 In continuing our previous work,26,55–59 we decided to introduce another application of core shells, including Au–magnetic organic hybrid in MRI, CT imaging, and drug delivery.
2. Au–magnetic organic hybrid core–shells
2.1. Fe3O4@SiO2@Au NPs
Fe3O4 NP was provided by ferrous (Fe2+) and ferric (Fe3+) through the co-precipitation method27 with sodium hydroxide (NaOH) under inert nitrogen gas at room temperature. To obtain the Fe3O4 @Au CSNPs, the Fe3O4 was added to the HAuCl4 solution in Na3C6H5O7 and sodium citrate (CS) under sonicated conditions. According to the result, Fe3O4@Au CSNPs are suitable for CT and MRI imaging. The importance of this method is to give Fe3O4@Au CSNPs through the rapid sonochemical synthesis (Scheme 1).28
 |
| Scheme 1 The preparation of Fe3O4@Au by sonochemical method. | |
2.2. Fe3O4@SiO2@Au NPs
Cisplatin (cPt) was doped on Fe3O4@SiO2@Au to cure cancer, which was detected through MRI images. Hydrophobic Fe3O4 NPs were synthesized by a thermal decomposition method29,30 using FeCl3·6H2O and sodium oleate in ethanol/hexane. In the next step, Fe3O4@SiO2 was obtained through the reverse microemulsion method.31 In this method, the mixture of Igepal CO-520 in cyclohexane and ammonium hydroxide was added to Fe3O4 NPs, followed by the addition of tetraethoxysilane (TEOS) and (3-aminopropyl)triethoxysilane (APTES) to yield Fe3O4@SiO2 core–shell nanostructures, which were added to Au NPs32 to obtain Fe3O4@SiO2@Au NPs. In the next step, 16-mercaptohexadecanoic acid (16-MHDA) as a linker with 16 carbon atoms containing thiol and carboxyl group was linked to the Au NPs surface and cPt, respectively (Scheme 2).33 The functionalized Fe3O4@SiO2@Au NPs are assessed in photothermal cancer therapy by the irradiation of two colon cancer cell lines (SW480 and SW620) with a laser (λ = 808 nm, W = 100 mW cm−2). It is found that laser irradiation showed the mortality of cancer cells. Because of the synergic effect of cPt and Au NPs and laser irradiation, the functionalized Fe3O4@SiO2@Au NPs are used for potential (MRI)-guided stimulated chemo-photothermal treatment of cancer.
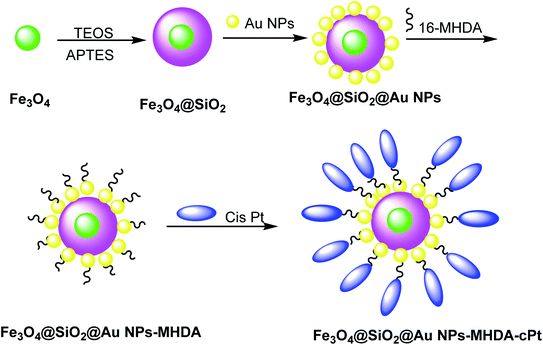 |
| Scheme 2 The preparation of Fe3O4@SiO2@Au NPs–MHDA–cPt. | |
2.3. Core–shell iron–gold (Fe@Au)
Core–shell iron–gold (Fe@Au) structures were used for MRI imaging and targeted drug delivery. They were obtained through the reverse micelle method. In this method, the iron–gold nanoparticles were coated with polyglycerol, thiol, and polymerized glycidol. Spherical core particles of iron with a thin layer of gold shell were decorated with 2-mercaptoethanol, which was linked to Au from S head to yield the Au–S on the core–shell surfaces (Scheme 3).34
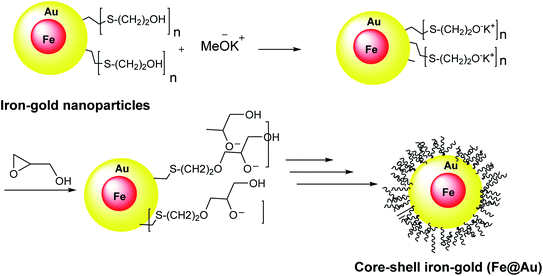 |
| Scheme 3 The preparation of core–shell iron–gold (Fe@Au). | |
2.4. Fe3O4@SiO2PrNH2@Au
Keshtkar et al.37 designed Fe3O4@Au by magnetic iron oxide nanoparticles through the co-precipitation process,35 followed by silica according to the Stöber method.36 Consequently, Fe3O4 nanoparticles were functionalized by (3-aminopropyl)triethoxysilane (APTES) to provide Fe3O4@SiO2PrNH2, which was added to the suspension of gold nanoparticles in H2O to produce Fe3O4@SiO2PrNH2@Au. Then, 3-(4,5-dimethylthiazol-2-yl)-2,5-diphenyltetrazolium bromide as human hepatocellular carcinoma was immobilized on the surface of Fe3O4@SiO2PrNH2@Au to give Fe3O4@SiO2PrNH2@Au + drugs, which was applied as MRI and CT agent (Scheme 4). Fe3O4@SiO2PrNH2@Au was provided through the synthetic strategy according to the laser ablation in liquid (LAL) as a green route to achieve NPs in one step (Scheme 5).38–41
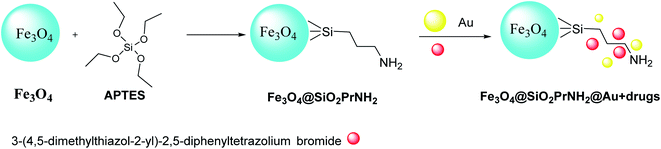 |
| Scheme 4 The preparation of Fe3O4@SiO2PrNH2@Au + drugs. | |
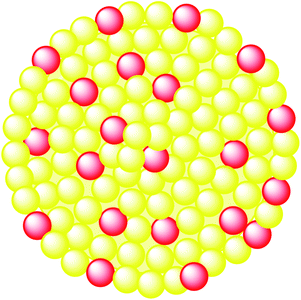 |
| Scheme 5 The structure of designed Fe3O4@Au. | |
2.5. Fe/Fe3O4PrNH2@Au
Iron/iron oxide nanoparticles Fe/FexOy@Au were provided through the electric arc discharge method. Then, particles were mixed with (3-aminopropyl)trimethoxysilane (APTMS) under sonicated conditions to provide Fe/Fe3O4PrNH2, which was functionalized by gold nanoparticles42 to provide Fe/Fe3O4PrNH2@Au. The designed Fe/Fe3O4PrNH2@Au nanoparticles as bifunctional magnetic plasmonic nanostructures were used in magnetic resonance imaging and magneto-optical thermal therapies (Scheme 6).43
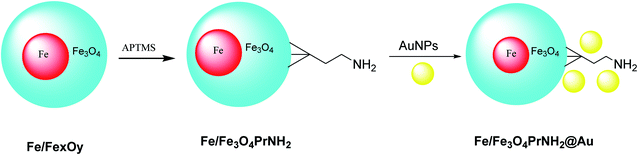 |
| Scheme 6 The preparation Fe/Fe3O4PrNH2@Au nanoparticles. | |
2.6. Au@Bi2S3 NBs
Au@Bi2S3–PVP nano bone, which can be used as a multimodal imaging agent for PT/PA/CT image, was reported by Ouyang et al.45 In the first step, Au NPs were synthesized44 and mixed with L-ascorbic acid (AA), hexamethylenetetramine, and thioacetamide (TAA) to give Au NR Au@Bi2S3 NBs as the theranostic agent in cancer therapy, which was added to bismuth acetate to give Au@Bi2S3 core–shell NBs, followed by mixing with PVP to produce Au@Bi2S3–PVP NBs nano bone as an imaging agent for applying in the tumor cells (Scheme 7). The structure can be used as a nanocarrier for anticancer drugs (DOX) to be released at a special pH.
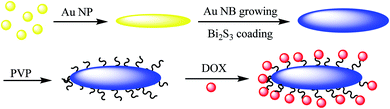 |
| Scheme 7 The preparation of NR Au@Bi2S3 NBs. | |
2.7. D-Au@Gd&RGD
Gold nanoparticles was provided to be functionalized by mitochondria-targeting group (Mito-S), rhodamine B derivative (RH-S), fluorescein derivative (Flu-S), tetraaza macrocyclic compounds (TAMC-S), cyclic arginine–glycine–aspartic acid peptide (cRGD-S) to yield a mono-sensitive compound. The latter was treated with Gd2+ to provide D-Au@Gd&RGD as the dual-sensitive structure. The application of the synthesized compound was used in MR and fluorescence imaging for a tumor in mice for tumor imaging and penetrate the blood–brain barrier (BBB) for central nervous system (CNS) problems (Scheme 8).46
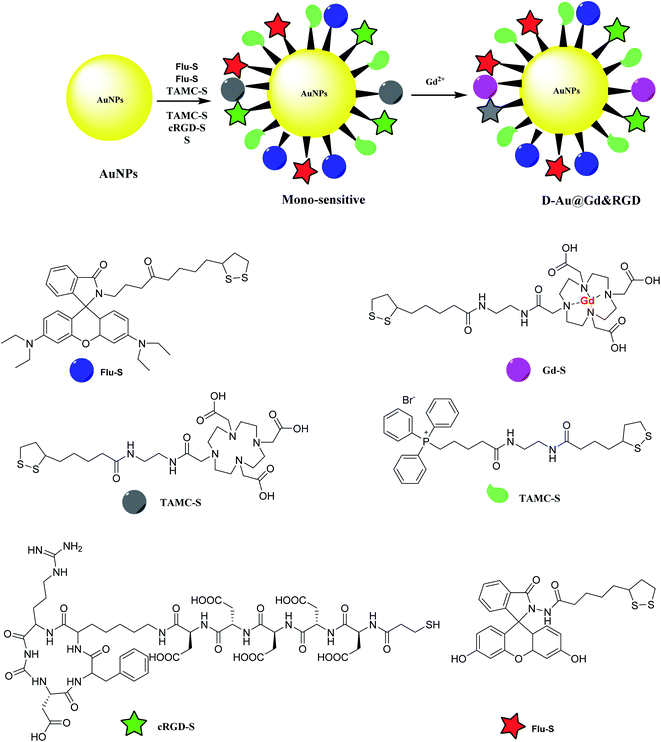 |
| Scheme 8 The preparation of D-Au@Gd&RGD. | |
2.8. APG@OVA NPs
The multifunctional gadolinium-doped Au@Prussian blue nanoparticles (Au@PB–Gd@OVA) were designed as MR/SERS bimodal agents. Prussian blue nanoparticles (PB) as cyanide (CN)-bridged coordination polymer were immobilized onto the AuNP core to give a background-free surface-enhanced Raman scattering (SERS) signal. The presence of doped Gd3+ provided a sensitive agent for MRI. Through the coating of ovalbumin (OVA) physically, APG@OVA NPs were provided.47 First step: The Au NPs were prepared through the classic sodium citrate reduction process using sodium citrate and HAuCl4 solutions. Second step: K4[Fe(CN)6] and FeCl3 were applied as the Prussian blue nanoparticles (PB) agent, which was reacted with GdCl3. These two solutions were mixed to provide APG NPs, which was added to the OVA solution to yield Au@Prussian blue-Gd@ovalbumin nanoparticles (APG@OVA NPs) (Scheme 9).48
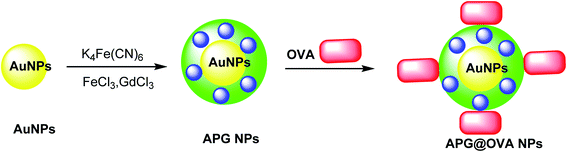 |
| Scheme 9 The preparation of APG@OVA NPs. | |
2.9. Au@MnO2
In this study, a gold@manganese dioxide (Au@MnO2) as core–shell structure was synthesized, which was functionalized by glutathione GSH as a theranostic agent in photoacoustic and magnetic resonance study. The GSH-triggered Au@MnO2 was applied in photoacoustic and MRI as a smart theranostic nanostructure for cancer diagnosis and treatment (Scheme 10).49
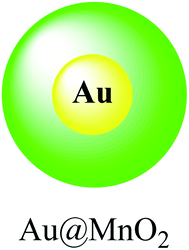 |
| Scheme 10 The preparation of Au@MnO2. | |
The nanostructure of AuPd@PVP is important due to their similar magnitudes to the biomolecules, which was used for in biotechnology and medicine. The chloroauric acid hydrated solution was added to the Au precursor; and then disodium tetrachloropalladate was mixed with ascorbic acid as a reducing agent to give AuPd (Scheme 11).50 The resulting product AuPd was mixed with polyvinyl pyrrolidone (PVP) as a surfactant to give AuPd@PVP nanoparticles for biocompatibility in vivo and in vitro study in photothermal therapy (PTT) and radiotherapy (RT). Therefore, PTT and RT could be applied for cancer therapy. The AuPd@PVP NPs have photothermal therapy (PTT) activity under NIR laser irradiation at a low power. Moreover, the NPs could be applied in radiotherapy (RT) as the sensitizer agent. Through PTT and RT, AuPd@PVP core–shell nanoparticles could be efficient for cancer therapy.
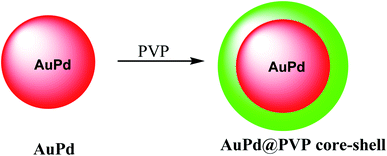 |
| Scheme 11 The preparation of AuPd@PVP core–shell nanoparticles. | |
2.10. Fe3O4@Au
The manganese dioxide-encapsulated gold nanoparticle (Au@MnO2 NP) was decorated by polyethylene glycol (PEG) to provide Au@MnO2–PEG and decomposed into the naked Au NPs and Mn2+ in acidic media. The resulting structure was absorbed by biomolecules to give a stimuli-responsive surface-enhanced Raman scattering (SR-SERS) nanoprobe (Scheme 12). The SR-SERS probes determined the difference between tumor and normal tissues by accuracy and even in different growth steps.
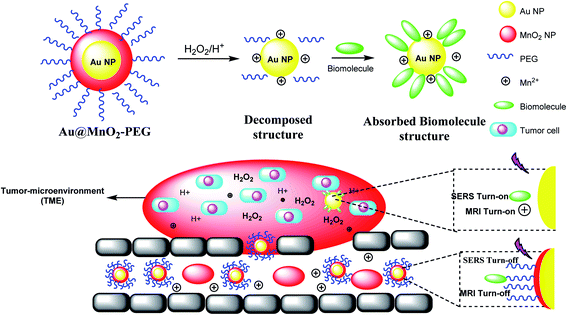 |
| Scheme 12 The preparation of SR-SERS nanoprobe. | |
A stimulus-responsive surface-enhanced Raman scattering (SR-SERS) nanoprobe was designed to diagnose the tumor cells. The SR-SERS probes were activated by the tumor microenvironment (TME); however, in other tissues, which were highly selective to tumors, there was no activation. As a result, TME-triggered exposure with Au NPs and biomolecules could meaningfully increase; moreover, the Raman fingerprints show the complete information, compared with the traditional molecular method. The importance of the SR-SERS probes is to make a difference between tumors and healthy tissues, which might be applicable for the treatment of cancers (Scheme 12).46 In fact, Au@MnO2 nanoparticles were produced through a layer-by-layer approach. Thus, the obtained Au@MnO2-PEG nanoparticles treated with H2O2 in the tumor to provide Mn2+ for improving T1-weighted MR imaging to create O2 for removing the cancer cells to X-rays. Therefore, Au nanoparticles increase the X-ray energy in tumor cells, and MnO2 reacts with endogenous tumor H2O2 to create O2 in hypoxia-associated RT resistance. Through the combination of gold nanoparticles and O2 generation by MnO2 shells, Au@MnO2-PEG core–shell nanoparticles, there is a good tumor therapeutic effect.
2.11. EGaIn–Au NPs
To give nano-composites through liquid metals (LM), a mixture of gallium indium–Au nanostructures (EGaIn–Au NPs) was used for providing radio-photothermal cancer treatment. In this process, Au nanodots were reduced onto the mixture of gallium indium (EGaIn) NPs surface to achieve EGaIn–Au NPs. This strategy might open a new door to a LM-based nano-composite. The EGaIn NPs were designed through the probe-sonicating method in xanthan gum solution after centrifuging the spherical EGaIn NPs, and HAuCl4 solution was mixed with EGaIn NPs for growth of Au NPs by the interfacial galvanic replacement reactions to give EGaIn–Au nano-composites (Scheme 13).51 It was proved that the EGaIn–Au nano-composite was used to respond the X-ray and NIR (near-infrared laser) irradiation. The nano-composite with photothermal conversion and radiosensitization ability destroy cancer cells to cure. However, healthy tissues are damaged, they used for decreasing the growth of tumor tissues by NIR, and X-ray treatment in photothermal therapy and radiotherapy.
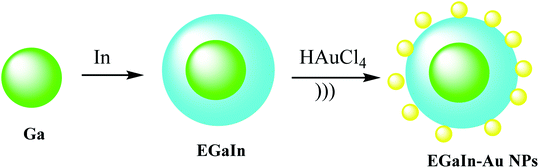 |
| Scheme 13 The preparation of EGaIn–Au NPs. | |
2.12. I–Pd@Au–PEG–FA nanosheets
Pd@Au–PEG–FA nanosheets were used as CT image contrast agents. The Pd@Au nanosheets were synthesized according to previous reports.52–54 FA–NHS was obtained from folic acid (FA), N-hydroxysuccinimide (NHS), and dicyclohexylcarbodiimide (DCC) in DMSO in the dark to provide FA–NHS, which was added to the NH2–PEG–SH to provide Pd@Au–PEG–FA nanosheets, and then it was mixed with FA–PEG–SH to provide Pd@Au–PEG–FA nanosheets. Preparation of I–Pd@Au–PEG–FA nanosheets was accomplished through the reaction of Pd@Au–PEG–FA with radioiodine at room temperature, which was used to load radioiodine by 125Na131I at room temperature (Schemes 14 and 15).51 I–Pd@Au–PEG–FA nanosheets were then applied on detecting the plaques by reforming the 2D multifunctional structure by FA on the surface and evaluate the target specificity for the activated macrophages; the targeted probes show that 2D Pd@Au nanosheets have superior pharmacokinetic to achieve the cure effect.
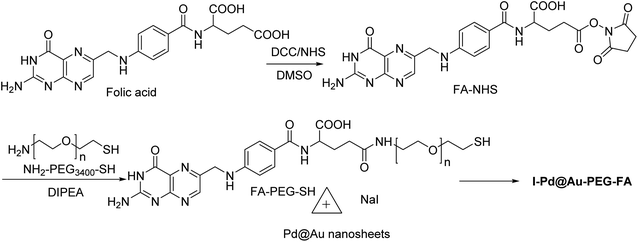 |
| Scheme 14 The preparation of core–shell I–Pd@Au–PEG–FA. | |
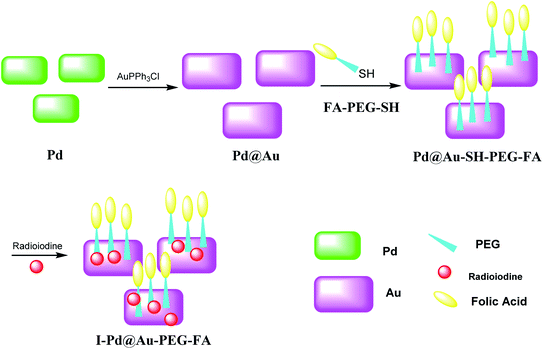 |
| Scheme 15 The preparation of I–Pd@Au–PEG–FA nanosheets. | |
3. Conclusion
Au nanoparticles received much attention for cancer treatment and MRI due to their high chemical stability, biocompatibility, and affinity for binding with thiol terminal groups of various organic compounds. Moreover, these mixtures give the magnetic and plasmonic properties to nanoparticles for diagnostics and therapeutic applications. However, the currently available synthesis methods for these nanoparticles are based on organic compounds.
Conflicts of interest
The authors declare that there is no declaration of competing interest in this paper.
Acknowledgements
The authors thank the research support council of Alzahra University and University of Tehran.
References
- L. Ai, Y. Yang, B. Wang, J. Chang, Z. Tang, B. Yang and S. Lu, Sci. Bull., 2020 DOI:10.1016/j.scib.2020.12.015.
- Y. Xu, B. Wang, H. Zhang, X. Qu, M. Zhang, X. An, S. Lu and S. Zhang, Blood, 2019, 134, 941 CrossRef.
- N. A. D. Burke, H. D. H. Stöver and F. P. Dawson, Chem. Mater., 2002, 14, 4752–4761 CrossRef CAS.
- C. R. Vestal and Z. J. Zhang, J. Am. Chem. Soc., 2002, 124, 14312–14313 CrossRef CAS.
- A. Maliakal, H. Katz, P. M. Cotts, S. Subramoney and P. Mirau, J. Am. Chem. Soc., 2005, 127, 14655–14662 CrossRef CAS.
- Z. Du, X. Yin, M. Zhang, Q. Hao, Y. Wang and T. Wang, Mat. Lett., 2010, 64, 2076–2079 CrossRef CAS.
- T. R. Dhakal, S. R. Mishra, Z. Glenn and B. Rai, J. Nanosci. Nanotechnol., 2012, 12, 6389–6396 CrossRef CAS.
- T. Kim, H. Kang, S. Jeong, D. J. Kang, C. Lee, C.-H. Lee, M.-K. Seo, J.-Y. Lee and B. J. Kim, ACS Appl. Mater. Interfaces, 2014, 6, 16956–16965 CrossRef CAS.
- H. Chen, W. Dong, J. Ge, C. Wang, X. Wu, W. Lu and L. Chen, Sci. Rep., 2013, 3, 1910 CrossRef.
- L. Xie, X. Huang, Y. Huang, K. Yang and P. Jiang, J. Phys. Chem., 2013, 117, 22525–22537 CAS.
- P. Liu, Colloids Surf., A, 2006, 291, 155–161 CrossRef CAS.
- J. Deng, C. He, Y. Peng, J. Wang, X. Long, P. Li and A. S. C. Chan, Synth. Met., 2003, 139, 295–301 CrossRef CAS.
- P. M. Khaniabadi, D. Shahbazi-Gahrouei, A. M. S. A. Majid, M. S. Jaafar, B. M. Khaniabadi and S. Shahbazi-Gahrouei, Iran. Biomed. J., 2017, 21, 360–368 Search PubMed.
- H. Nosrati, M. Salehiabar, M. Fridoni, M.-A. Abdollahifar, H. K. Manjili, S. Davaran and H. Danafar, Sci. Rep., 2019, 9, 1–10 CrossRef CAS.
- M. Y. Badi, A. Azari, H. Pasalari, A. Esrafili and M. Farzadkia, J. Mol. Liq., 2018, 261, 146–154 CrossRef.
- A. Mesdaghinia, A. Azari, R. N. Nodehi, K. Yaghmaeian, A. K. Bharti, S. Agarwal, V. K. Gupta and K. Sharafi, J. Mol. Liq., 2017, 233, 378–390 CrossRef CAS.
- P. M. Khaniabadi, D. Shahbazi-Gahrouei, M. S. Jaafar, A. M. S. A. Majid, B. M. Khaniabadi and S. Shahbazi-Gahrouei, Avicenna J. Med. Biotechnol., 2017, 9, 181–188 Search PubMed.
- N. Othman, P. M. Khaniabadi, M. S. Jameel, M. A. Dheyab and I. Amiri, Plasmonics, 2020, 15, 123–133 CrossRef.
- M. A. Rabeea, M. N. Owaid, A. A. Aziz, M. S. Jameel and M. A. Dheyab, J. Environ. Chem. Eng., 2020, 8, 103841 CrossRef CAS.
- M. N. Owaid, M. A. Rabeea, A. A. Aziz, M. S. Jameel and M. A. Dheyab, Meas. Monit. Manag. Control, 2019, 12, 100270 Search PubMed.
- M. Falahati, F. Attar, M. Sharifi, A. A. Saboury, A. Salihi, F. M. Aziz, I. Kostova, C. Burda, P. Priecel and J. A. Lopez-Sanchez, Biochim. Biophys. Acta, Gen. Subj., 2020, 1864, 129435 CrossRef CAS.
- M. Baziar, A. Azari, M. Karimaei, V. K. Gupta, S. Agarwal, K. Sharafi, M. Maroosi, N. Shariatifar and S. Dobaradaran, J. Mol. Liq., 2017, 241, 102–113 CrossRef CAS.
- D.-K. Kim, J.-W. Kim, Y.-Y. Jeong and S.-Y. Jon, Bull. Korean Chem. Soc., 2009, 30, 1855–1857 CrossRef CAS.
- J. K. Lim, S. A. Majetich and R. D. Tilton, Langmuir, 2009, 25, 13384–13393 CrossRef CAS.
- M. Liu, X. Ren, X. Meng and H. Li, Chin. J. Chem., 2020 DOI:10.1002/cjoc.202000410.
- G. Mohammadi Ziarani, P. Mofatehnia, F. Mohajer and A. Badiei, RSC Adv., 2020, 10, 30094–30109 RSC.
- A. Magdalena, I. Silva, R. Marques, A. Pipi, P. Lisboa-Filho and M. Jafelicci Jr, J. Phys. Chem. Solids, 2018, 113, 5–10 CrossRef CAS.
- M. A. Dheyab, A. A. Aziz, M. S. Jameel, O. A. Noqta, P. M. Khaniabadi and B. Mehrdel, Mater. Today Commun., 2020, 25, 101368 CrossRef CAS.
- J. Park, K. An, Y. Hwang, J.-G. Park, H.-J. Noh, J.-Y. Kim, J.-H. Park, N.-M. Hwang and T. Hyeon, Nat. Mater., 2004, 3, 891–895 CrossRef CAS.
- M. V. Kovalenko, M. I. Bodnarchuk, R. T. Lechner, G. Hesser, F. Schäffler and W. Heiss, J. Am. Chem. Soc., 2007, 129, 6352–6353 CrossRef CAS.
- H. L. Ding, Y. X. Zhang, S. Wang, J. M. Xu, S. C. Xu and G. H. Li, Chem. Mater., 2012, 24, 4572–4580 CrossRef CAS.
- D. G. Duff, A. Baiker, I. Gameson and P. P. Edwards, Langmuir, 1993, 9, 2310–2317 CrossRef CAS.
- A. Maximenko, J. Depciuch, N. Łopuszyńska, M. Stec, Ż. Światkowska-Warkocka, V. Bayev, P. M. Zieliński, J. Baran, J. Fedotova and W. P. Węglarz, RSC Adv., 2020, 10, 26508–26520 RSC.
- T. Jafari, A. Simchi and N. Khakpash, J. Colloid Interface Sci., 2010, 345, 64–71 CrossRef CAS.
- S. Beyaz, H. Kockar and T. Tanrisever, J. Optoelectron. Adv. M., 2009, 1, 447–450 Search PubMed.
- W. Stöber, A. Fink and E. Bohn, J. Colloid Interface Sci., 1968, 26, 62–69 CrossRef.
- M. Keshtkar, D. Shahbazi-Gahrouei and A. Mahmoudabadi, J. Medical Signals Sens., 2020, 10, 201–207 Search PubMed.
- S. J. Ratnakar, T. C. Soesbe, L. L. Lumata, Q. N. Do, S. Viswanathan, C.-Y. Lin, A. D. Sherry and Z. Kovacs, J. Am. Chem. Soc., 2013, 135, 14904–14907 CrossRef CAS.
- K. Li, J.-T. Hou, J. Yang and X.-Q. Yu, ChemComm, 2017, 53, 5539–5541 RSC.
- L.-L. Li, K. Li, M.-Y. Li, L. Shi, Y.-H. Liu, H. Zhang, S.-L. Pan, N. Wang, Q. Zhou and X.-Q. Yu, Anal. Chem., 2018, 90, 5873–5878 CrossRef CAS.
- V. Torresan, D. Forrer, A. Guadagnini, D. Badocco, P. Pastore, M. Casarin, A. Selloni, D. Coral, M. Ceolin and M. B. Fernández van Raap, ACS Nano, 2020, 14, 12840–12853 CrossRef CAS.
- S. L. Westcott, S. J. Oldenburg, T. R. Lee and N. J. Halas, Langmuir, 1998, 14, 5396–5401 CrossRef CAS.
- E. Kheradmand, R. Poursalehi and H. Delavari, Appl. Nanosci., 2020, 10, 1083–1094 CrossRef CAS.
- X. Ye, L. Jin, H. Caglayan, J. Chen, G. Xing, C. Zheng, V. Doan-Nguyen, Y. Kang, N. Engheta, C. R. Kagan and C. B. Murray, ACS Nano, 2012, 6, 2804–2817 CrossRef CAS.
- R. Ouyang, P. Cao, P. Jia, H. Wang, T. Zong, C. Dai, J. Yuan, Y. Li, D. Sun, N. Guo, Y. Miao and S. Zhou, Bioact. Mater., 2021, 6, 386–403 CrossRef.
- C. Zhang, X. Cui, J. Yang, X. Shao, Y. Zhang and D. Liu, Chem. Sci., 2020, 6111–6120 RSC.
- C. Zhang, Z. Xu, H. Di, E. Zeng, Y. Jiang and D. Liu, Theranostics, 2020, 10, 6061–6071 CrossRef CAS.
- Y. Li, C. H. Li and D. R. Talham, Nanoscale, 2015, 7, 5209–5216 RSC.
- H. Wang, L. An, C. Tao, Z. Ling, J. Lin, Q. Tian and S. Yang, Nanoscale, 2020, 12, 5139–5150 RSC.
- Y. Xiang, X. Peng, X. Kong, Z. Tang and H. Quan, Colloids Surf., A, 2020, 124652 CrossRef CAS.
- Z. Guo, L. Yang, M. Chen, X. Wen, H. Liu, J. Li, D. Xu, Y. An, C. Shi, J. Li, X. Su, Z. Li, T. Liu, R. Zhuang, N. Zheng, H. Zhu and X. Zhang, Nano Res., 2020, 13, 173–182 CrossRef.
- M. Chen, S. Tang, Z. Guo, X. Wang, S. Mo, X. Huang, G. Liu and N. Zheng, Adv. Mater., 2014, 26, 8210–8216 CrossRef CAS.
- M. Chen, Z. Guo, Q. Chen, J. Wei, J. Li, C. Shi, D. Xu, D. Zhou, X. Zhang and N. Zheng, Sci. Adv. Mater., 2018, 9, 4268–4274 CAS.
- Z. Guo, M. Chen, C. Peng, S. Mo, C. Shi, G. Fu, X. Wen, R. Zhuang, X. Su and T. Liu, Biomaterials, 2018, 179, 134–143 CrossRef CAS.
- V. Fathi Vavsari, G. Mohammadi Ziarani and A. Badiei, RSC Adv., 2015, 5(111), 91686–91707 RSC.
- G. Mohammadi Ziarani, M. Malmir, N. Lashgari and A. Badiei, RSC Adv., 2019, 9(43), 25094–25106 RSC.
- G. Mohammadi Ziarani, Z. Hassanzadeh, P. Gholamzadeh and S. Asadi, RSC Adv., 2016, 6(26), 21979–22006 RSC.
- M. Mehdi, A. Badiei and G. Mohammadi Ziarani, RSC Adv., 2015, 5(46), 36530–36539 RSC.
- G. Mohammadi Ziarani, S. Rohani, A. Badiei and A. Ziarati, RSC Adv., 2018, 8, 41048–41100 RSC.
|
This journal is © The Royal Society of Chemistry 2021 |