DOI:
10.1039/D1RA00197C
(Paper)
RSC Adv., 2021,
11, 6188-6200
Synthesizing and evaluating the photodynamic efficacy of asymmetric heteroleptic A7B type novel lanthanide bis-phthalocyanine complexes†
Received
9th January 2021
, Accepted 20th January 2021
First published on 3rd February 2021
Abstract
In this study heteroleptic A7B type novel Lu(III) and Eu(III) lanthanide phthalocyanines (LnPc(Pox)[Pc′(AB3SH)]) with high extinction coefficients have been synthesized as candidate photosensitizers with reaction yields higher than 33%. The singlet oxygen quantum yields of LuPc(Pox)[Pc′(AB3SH)] and EuPc(Pox)[Pc′(AB3SH)], respectively, were measured 17% and 1.4% by the direct method in THF. The singlet oxygen quantum yield of LuPc(Pox)[Pc′(AB3SH)] in THF is the highest among lutetium(III) bis-phthalocyanine complexes to date. The photodynamic efficacy of the heteroleptic lanthanide phthalocyanines was evaluated by measuring cell viabilities of A549 and BEAS-2B lung cells, selected to representing in vitro models for testing cancer and normal cells against potential drugs. The cell viabilities demonstrated concentration dependent behavior and were varied by the type of phthalocyanines complexes. Irradiation of the cells for 30 minutes with LED array at 660 nm producing flux of 0.036 J cm−2 s−1 increased cell death for LuPcPox-OAc, LuPc(Pox)[Pc′(AB3SH)] and ZnPc. The IC50 concentrations of LuPc(Pox)[Pc′(AB3SH)] and ZnPc were determined to be below 10 nM for both cell lines, agreeing very well with the singlet oxygen quantum yield measurements. These findings suggest that LuPc(Pox)[Pc′(AB3SH)] and particularly LuPcPox-OAc are promising drug candidates enabling lowered dose and shorter irradiation time for photodynamic therapy.
Introduction
Sandwich-type phthalocyanine complexes of rare-earth elements (Ln(III)bisPcs) are well-studied classes of coordinating compounds with tetrapyrrole ligands.1–4 Owing to characteristic overlapping of ligand with π-orbitals,5 which is supplemented with a specific interaction of two f-electronic systems in the case of triple-decker complexes,6,7 these compounds possess a wide range of unique properties determining a variety of applications.8–11 The structural modifications in sandwich-type phthalocyanines referring synthesis of mixed-ligand and heteronuclear derivatives,12 effectively control the intramolecular interactions and tailor materials' properties.13,14
Homoleptic Ln(III)bisPcs are made of two identical phthalocyanine rings whereas heteroleptic complexes are composed of two different phthalocyanine rings. Bis-phthalocyanines are naturally neutral organic radicals in which one macrocycle is negatively charged and the other macrocycle is deprotonated π-radical while the oxidation state of the central metal ion is (+3). In heteroleptic Ln(III)Pcs family, synthesis of asymmetric heteroleptic Ln(III)bisPcs functionalized in only one of the eight isoindole subunits of the bis- are rarely reported because it is a highly challenging in terms of chemical synthesis. In general, there are four alternative synthetic approaches for the preparation of heteroleptic bis-complexes depending on the type of reactants involved in the reactions: (1) two different metal-free Pc ligands reacting with a rare-earth salt, (2) reaction of the mono rare-earth complexes with phthalonitrile or its analogs, (3) reaction of two different phthalonitrile in the presence of a rare-earth salt and (4) mono rare-earth complexes and metal-free or alkali metal macrocycles reaction.
Photodynamic therapy (PDT) is a cancer treatment method receiving increased attention recently. PDT is a binary therapy (hopefully curative) that requires combination of preferably near-infrared light and a photosensitizer (PS). Each component is harmless by itself, but, when they are combined that they lead to the generation of reactive oxygen species (ROS) causing oxidative cell damage and eventually kill cells. Phthalocyanines (Pcs) are among promising second-generation photosensitizers having extended four benzo rings on the pyrrole units resulting in enhanced absorption in the far-red/infrared region. Phthalocyanines are emerging as PS-candidates with a strong light absorption ability and capable of generating higher numbers of singlet oxygen initiating death of cancer cells triggered by light. The optical properties of candidate PSs can be finely tuned in many ways through modifying type of coordinating metal15,16 and structure of ligand moieties.17 These capabilities make synthesis of novel Pc metal complexes including the lanthanides attractive.
Among the new generation Pc complexes, lanthanide(III) phthalocyanines are of a high interest because of possible coordination of two or more Pc macrocyclic units per metal atom forming LnPc2, or Ln2Pc3.18–20 Lutetium ion (Lu3+) has high interest due to its ability to coordinate two or more Pc-molecules per one Lu ion.21,22 These lanthanide bis-phthalocyanine derivatives exhibit high intrinsic electrical conductivity with exciting electrochemical and electrochromic properties.4 The optical properties of LnPc2 such as higher extinction coefficient in the far-red region (>670 nm) with an optimal singlet oxygen quantum yield (>0.3) and red-shifted fluorescence (>680 nm) are favorable for PDT applications. The presence of the heavy lanthanide central metal23,24 enhances the rate of intersystem crossing (ISC) to the triplet state.25 Therefore, LnPc2 complexes are expected to have higher excited state absorption in the triplet state compared to that in the singlet state due to enhanced intersystem crossing (ISC). The well-known lutetium complexes used in PDT are texaphyrin derivatives which are clinically well accepted for radiation and photodynamic therapy in oncology.26,27 The second metal ion for formation of LnPc2 complex, which is explored in the present study, is europium(III). Similar to Lu, Eu ions tend to form bis-structures having a high yield of singlet oxygen (1O2) generation.28,29
To the best of our knowledge there is no report on synthesis of the A7B target asymmetric heteroleptic Eu(III) and Lu(III)bisPcs with a polar thiol group for the “B” part, and for A part hydrophobic polyoxyethylene chains in the PDT application. In this study, we present synthesis methods allowing expeditious access to these target derivatives, schematically shown in Fig. 1, which can be described as A7B complexes. The significance of this study is thus devoted to developing synthesis methods for A7B targeted asymmetric heteroleptic Pc complexes, and evaluating biological responses of cells towards these novel phthalocyanine complexes as candidate photosensitizers in PDT. The Pc rings are substituted with polyoxyethylene substituents at the peripheral positions to enhance the solubility. Introduction of mercaptohexanol on the eighth isoindole subunit of the Pc ring is to ease potential conjugation of these Pcs to noble metal nanoparticles.
 |
| Fig. 1 Structures of the target heteroleptic thiol functionalized lanthanide bis-phthalocyanine complexes: Ln = Eu(III) or Lu(III). | |
Photosensitizers can be activated with specific light irradiation and produce reactive oxygen species (ROS) such as singlet oxygen, oxygen free radicals that may be produced only in the irradiated area. Therefore, it is crucial to evaluate toxic influence of PSs by measuring cell viability and to determine IC50 values in vitro conditions. We demonstrated that LuPcPox(OAc) among the Pcs tested offers higher photodynamic efficacy for A549 and BEAS-2B cells in vitro conditions.
Experimental section
Materials
All solvents and reagents were of reagent grade quality, and obtained commercially from Aldrich, Fluka or Merck. Comprehensive synthesis methods and characterizations of phthalodinitrile FN-Pox, FN-OH and mono-Ln-phthalocyanine derivatives (H2PcAB3OH, LnPcPox(OAc); Ln: Lu(III) or Eu(III)) as starting materials were provided in the ESI (Scheme S1 and Fig. S1–S24).†
Synthesis of asymmetric heteroleptic A7B type bis-phthalocyanines
Hydroxylated asymmetric heteroleptic bis-phthalocyanines [LnPc(Pox)[Pc′(AB3OH)] (Ln = Lu(III) or Eu(III)).
General procedure. In a reaction flask 1 eq. of H2PcAB3OH and 1 eq. (0.19 g, 0.087 mmol) for LuPcPox, or 1 eq. (0.16 g, 0.087 mmol) for EuPcPox were dissolved in 5 mL dry o-DCB/octanol mixture and 2–3 drops of organic base DBU was added at 190 °C under argon atmosphere and achieved for 6 h. The reaction was cooled to room poured in 50 mL hexane solution. For purification silica gel column chromatography was used by dichloromethane/ethanol (15
:
1) as an eluent mixture. LuPc(Pox)[Pc′(AB3OH)] and EuPc(Pox)[Pc′(AB3OH)] molecular formula C168H240LuN16O43S15 and C168H240EuN16O43S15, molecular weight was calculated 3827.70 and 3804.70 g mol−1 respectively. Yield: 0.15 g (45%) and 0.21 g (55%), respectively.
LuPc(Pox)[Pc′(AB3OH)]. FT-IR (ATR): νmax (cm−1), 3478.2 (OH), 2913–2869.7 (CH2, CH3), 1593 (C–N), 1324, 1281, 1197, 1101–1028 (C–O–C). MALDI-TOF (matrix: DIT): calculated C168H240LuN16O43S15 [M]+ 3827.70 m/z, founded [M]+ 3827.69 m/z. UV-Vis-NIR (CHCl3), λmax/nm: 315, 535, 631, 699, 949, 1356–1973.
EuPc(Pox)[Pc′(AB3OH)]. FT-IR (ATR): νmax (cm−1), 3502.5 (OH), 2914–2864.8 (CH2, CH3), 1592.4 (C–N), 1314.3, 1281.3, 1198.3, 1103.3–1027.8 (C–O–C). MALDI-TOF (matrix: DIT): calculated C168H240EuN16O43S15 [M]+ 3804.70 m/z, founded [M + 7H]+ 3813.97 m/z. UV-Vis-NIR (CHCl3), λmax/nm: 317, 573, 638, 710, 942, 1402–1896.
Mesylated asymmetric heteroleptic bis-phthalocyanines (LnPc(Pox)[Pc′(AB3Mes)], Ln: Lu(III) or Eu(III)).
General procedure. In an ice bath ∼0 °C, LnPc(Pox)[Pc′(AB3OH)] (0.15 g, 0.04 mmol for Lu complex or 0.25 g, 0.05 mmol for Eu complex) was dissolved in dichloromethane (150 mL) in the presence of trimethylamine (2.5 mL, excess). 2.5 mL (excess of) methane sulfonyl chloride was added dropwise to the solution during 1 h. The mixture was allowed to warm to room temperature and stirred for 4 h. The reaction was followed by thin-layer chromatography (TLC) using a 15
:
1 dichloromethane
:
ethanol solvent mixture as an eluent. When the starting complexes were completely consumed, first 200 mL saturated NaHCO3 solution was added to the solution and stirred vigorously for 5 min, then 200 mL diluted HCl solution was added. The two phases resulting mixture was extracted with more dichloromethane, organic phase was dried with anhydrous sodium sulphate and evaporated dichloromethane. The crude products mesylated Ln-complexes were purified by preparative TLC (silica gel) using a 15
:
1 dichloromethane
:
ethanol solvent system as an eluent. Yield: Lu(Pox)Pc(AB3Mes)Pc′ 0.11 g (75%), Eu(Pox)Pc(AB3Mes)Pc′ 0.16 g (72%) respectively.
LuPc(Pox)[Pc′(AB3Mes)]. FT-IR (ATR): νmax (cm−1), 2870.6 (CH2, CH3), 1590 (C–N), 1330.9–1194.2 (–S
O), 1248, 1071 (C–O–C), 942.5. MALDI-TOF (matrix: DIT): calculated C168H242LuN16O45S16 [M]+ 3905 m/z, founded [M]+ 3905.79 m/z. EuPc(Pox)[Pc′(AB3Mes)]: FT-IR (ATR): νmax (cm−1), 2860.7–2929.6 (CH2, CH3), 1590.7 (C–N), 1313.3–1197.7 (–S
O), 1280.1, 1097.3, 1024.8 (C–O–C). MALDI-TOF (matrix: DIT): calculated C169H242EuN16O45S16 [M]+ 3882.79 m/z, founded [M + H]+ 3883.87 m/z.
Thiol substituted asymmetric heteroleptic bis-phthalocyanines (LnPc(Pox)Pc′(AB3SH)], Ln: Lu(III) or Eu(III)).
General procedure. In a reaction flask LnPc(Pox)[Pc′(AB3Mes)] (0.11 g, 0.028 mmol) for Lu or 0.16 g, 0.04 mmol for Eu complex was dissolved in deoxygenated (sonicated for 30 min using an ultrasonic bath) tetrahydrofuran (THF)/ethanol (EtOH) mixture (75
:
25 mL). The solution was refluxed under an argon atmosphere in the dark. Then, thiourea (17 mg, excess) was added during reflux over the night. After the starting Ln complexes were consumed, argon was bubbled through the reaction mixture. Aqueous sodium hydroxide solution (20%, 12 mL) which was previously deoxygenated by sonication for 30 min was added and the mixture was refluxed for 2 h. When the reaction was complete (monitoring with TLC), the resulting mixture was poured into a mixture of dilute hydrochloric acid and ice and extracted with dichloromethane. The organic phase was extracted and dried with anhydrous sodium sulphate. The crude products were purified by preparative TLC (silica gel) using a 15
:
1 dichloromethane
:
ethanol solvent system as an eluent. Yield: 0.052 g (33%) for LuPc(Pox)[Pc′(AB3SH)] or 0.058 g (38%) for EuPc(Pox)[Pc′(AB3SH)].
LuPc(Pox)[Pc′(AB3SH)]. FT-IR (ATR): νmax (cm−1), 2867.1 (CH2, CH3), 2577.7 (S–H), 1591.5 (C–N), 1283.1, 1100–1028.3 (C–O–C). MALDI-TOF (matrix: DIT): calculated C168H240LuN16O42S16 [M]+ 3843.76 m/z, founded [M]+ 3843.58 m/z. UV-Vis-NIR (CHCl3), λmax/nm: 315, 533, 631, 700, 753, 953, 1467–1993.
EuPc(Pox)[Pc′(AB3SH)]. FT-IR (ATR): νmax (cm−1), 3502.5 (OH), 2914–2864.8 (CH2, CH3), 1592.4 (C–N), 1314.3, 1281.3, 1198.3, 1103.3–1027.8 (C–O–C). MALDI-TOF (matrix: DIT): calculated C169H240EuN16O46S16 [M]+ 3820.76 m/z, founded [M − H]+ 3819.68 m/z. UV-Vis-NIR (CHCl3), λmax/nm: 317, 574, 637, 703, 942, 1652–1890.
Cell culture
A549 cells (human non-small lung carcinoma cell lines) and BEAS-2B cells (non-tumorigenic human bronchial epithelium cells) were purchased from American Type Culture Collection. A549 cells were maintained in DMEM/F-12 (Biological Industries), supplemented with 10% FBS (fetal bovine serum) (GIBCO), 1% penicillin (Biological Industries) and 1% L-glutamine (Biological Industries). BEAS-2B cells were grown in bronchial epithelial cell growth medium (BEGM) bullet kit from Lonza. Both cell cultures were seeded in 96-well plates and kept at 37 °C in a 5% CO2 humidified incubator.
Irradiation of cells with LED array emitting at 660 nm
The cells seeded in the 96-wells were irradiated with red light at 660 nm using a light emitting diode array (LED). The distance between the LED source and the 96-well plate was set to 15 cm. The number of incoming red photons was 2000 μmol m−2 s−1, measured with a calibrated PAR meter (Apogee SQ-520). Light irradiation flux of 0.036 J cm−2 s−1 was used throughout the experiments. The flux and the temperature were not varied during the irradiation and continuously monitored. Variations in flux and temperature were determined to be about ±1%, ensuring unchanged conditions for the cells during the irradiation.30
The irradiation procedure is as follow: the 96-well plates were seeded with 1500 cells per well and incubated for 24 hours before adding the phthalocyanine complexes to the wells. The cells were incubated for 24 and 48 hours with various amounts (0.01 to 50 μM) of the phthalocyanine complexes. Before the LED irradiation, the cell medium was replaced with a fresh medium, eliminating the phthalocyanines that were not internalized. The irradiation time was set to 30 min.
Cell viability analysis
Cell viabilities in the dark and light conditions were evaluated by the MTT (3-(4,5-dimethylthiazol-2-yl)-2,5-diphenyltetrazolium bromide) (purchased from Sigma Aldrich) assay. The Pcs were dissolved in DMSO. All Pcs were transferred to cell culture media DMEM + FBS 10% or RPMI + FBS 10%. The final DMSO concentration was <0.001% (v/v). The control group of cells (A549 or BEAS-2B) had DMEM without DMSO in the viability test (MTT). The final concentration of the DMSO was considered negligible. The cells were incubated for further 24 and 48 hours following the treatment (light irradiation and dark conditions). MTT (0.5 μg mL−1) was added into each well and the plates were additionally incubated for 4 hours at 37 °C. The formazan crystals were solubilized by addition of DMSO at the end of the incubation period. The change in MTT absorbance was measured by a Varioskan spectrophotometer at both 570 nm and 720 nm, to determine the cell viability.
IC50 values were determined by applying curve fitting procedure to the cell viability data. We fitted a sigmoidal equation (reported in the ESI†) to obtain a dose–response curve reporting the IC50 value by using Origin software.
Results and discussion
Design and synthesis
For synthesis of asymmetric heteroleptic bis-Pcs it is a challenging work to control the number and location of the functional groups on the phthalocyanine ring which requires the synthesis of asymmetrically substituted derivatives. However, the increasing scientific interest of such mono-functional heteroleptic derivatives is evidenced by new research reports. For this special design most easy but less selective method belongs to reaction of two different phthalonitrile (PNs) in boiling solvent results with formation of single-decker, triple-decker, and open-chain oligomeric products, and separation of such reaction mixture seems to be very challenging.31–36 Recently, promising alternative one-step method was reported by Alpugan and co-workers37 to access mono functionalized heteroleptic lanthanide bis complexes. In their report, a mixture of different phthalonitrile in different proportions was used and the maximum yield (37%) for A7B heteroleptic bis-phthalocyanines and minimum for other complexes were achieved in the ratio of 10
:
1 in the presence of Eu(OAC)3 in refluxing dry pentanol. A reaction between rare-earth mono-Pc and PN represents better selective approach for the preparation of asymmetric heteroleptic bis-complexes.38 To reduce formation of not desired mono-Pcs, another approach for the preparation of asymmetric heteroleptic complex requires synthesis of A3B type macrocycle using a statistical template condensation of two different PNs and then its reaction with lanthanide salts and unsubstituted phthalocyanine.39–43 However, not surprisingly, overall yield of the target complexes are quite low.
In our study, the preparation of A7B type rare-earth asymmetric heteroleptic bis-Pc complexes operates interactions between lanthanide (Lu and Eu) mono-Pc complex and a metal-free asymmetric Pc macrocycle in a protic or an aprotic solvent44 which is a method 4 known as the “raise-by-one-story” method45 that is mentioned in the introduction part. Starting synthon 4-(6-mercaptohexan-1-ol)phthalonitrile (FN-OH) was synthesized according to literature reports,46 for production of 4,5-bis(4,7,10-trioxaundecan-1-sulfonyl)phthalonitrile (FN-Pox) simple solvent modifications47 was done. Metal free phthalocyanines H2PcAB3OH and H2PcPox were obtained by cyclic tetramerization of the phthalonitrile (FN-Pox and FN-OH) in refluxing solvent. For symmetric H2PcPox synthesis, two methods were applied. In method A; the first step was production of ZnPc first then second step by removing Zn metal to produce free Pc with reaction yield 72%. Even the reaction yield was higher than method B, time consuming two steps reactions and difficulty of purification of ZnPcPox directed us following method B. In method B which is based on in situ two reactions; Li-template assisted tetramerization, and in situ removal of the lithium ions by acidic treatments. In method B H2PcPox was obtained as a byproduct during synthesis of asymmetric phthalocyanine H2PcAB3OH. Our advantage in this method B is that the A4 metal free phthalocyanine derivative H2PcPox (yield 63%) which was obtained during the synthesis of asymmetric A3B type H2PcAB3OH (yield 35%) (Scheme S1†), it was reused to form mono-Ln(III)Pc metal complexes. Thus, two different symmetric and asymmetric phthalocyanine products that formed as a result of a single reaction were used again for formation of Lu(III) and Eu(III) bis-phthalocyanine complexes (Scheme 1). The substituted groups on asymmetric A7B type Ln(III)bisPcs are crucial for design of PDT active asymmetric heteroleptic bisPcs as a PS candidate.
In this context, our aim was not only herein to combine different substituted mono-Pc complex and a metal-free Pc macrocycle by using multiple reaction steps. Also a new synthetic approach have been developed to obtain targeted A7B type asymmetric heteroleptic Pcs containing only one thiol group (Scheme S1†). Ln(III)bisPcs containing SH group were prepared from LnPc(Pox)[Pc′(AB3OH) in two steps: mesylation and nucleophilic substitution by thiourea, with an overall yield of practically max. 38% (Scheme 1).48,49 The structures of novel compounds were characterized by a combination of IR, UV-Vis, MS and if possible 1H NMR, 13C NMR spectral data (Fig. S1–S42†). All results of the complexes are consistent with the assigned formulations (Schemes 1 and S1†). To mention, although satisfactory results could not be obtained, 1H NMR spectral experiments were carried out with reduced form of Lu and Eu bis-complexes.50
 |
| Scheme 1 Synthesis of asymmetric Lu(III) and Eu(III) bis-phthalocyanine complexes (Ln = Lu, Eu) (i) o-DCB/octanol, DBU, 190 °C, under argon; (ii) methane sulfonyl chloride, DCM, 0 °C; (iii) thiourea, THF/EtOH. | |
Ground state electronic absorption spectra
In accordance with their electronic structure, Pcs present intense π–π bands in the visible (Q band) and UV (B or Soret band) spectral regions.51 Absorption spectrum with typical spectral pattern of metallo phthalocyanines shows sharp and intense band in lowest energy region (674 nm) corresponding a π–π* transition, referred to as the Q band. The broad band in the 300–400 nm region consists of more than one component, including B1 and B2 bands, and referred to as Soret region.52 Several components observed at the foot of the Q band are considered to be of vibronic origin.53 The 500–600 nm area of the spectrum is called an optical window, where there is almost no light absorption. When additional bands are observed in the window region, for example due to charge transfer transitions between the central metal ion and the π ring, the color of the complex changes.54 The spectral properties of the lanthanide complexes are more complicated than for the metal phthalocyanines with only one Pc-ring. Furthermore, oxidation and reduction of the Pc ring produces π-cationic or π-anionic species, which have both of them characteristic spectroscopic properties.54 In our study, the Lambert–Beer law indicating linear relationship between absorbance and concentrations were observed for all lanthanide monomer and double decker complexes in DMSO and CHCl3 in the whole concentration measurement range (Fig. S37–S42†). The UV-NIR spectrum of neutral Ln(III)bisPcs show a relatively intense absorption at 631 nm and 662 nm for LuPc(Pox)[Pc′(AB3SH)] and EuPc(Pox)[Pc′(AB3SH)] complexes, respectively due to the phthalocyanine Q band. The broad band at 380 and 378 nm is attributed to the phthalocyanine Soret band. In addition, the intramolecular charge transfer (ICT) between two Pc rings of neutral Ln(III)bisPcs show broad and intense absorption regions in the infrared region appearing between 1400 and 1900 nm. The two n-radical anion bands appeared at 535(Lu), 571(Eu) nm (BV) and 943(Lu), 936(Eu) nm (RV). The position of the ICT band for the LnPc2 is dependent on the size of central rare earths.55 These results indicate that the interaction between the two rings becomes stronger as the ring-to-ring separation decreases along with the lanthanide contraction. More accurately, the blue-shift is due to the decreased distance between the Pc rings. The ionic radii of the lanthanides are in the order Lu −2.65 pm < Eu −2.84 pm.56 Consequently, the blue-shift is due to the decreased distance between the Pc rings. It can be correlated with the radius of the lanthanide(III). Our results are consistent with the reports showing that for Ln(Pc)2.10,57 The spectral changes due to chemical oxidation in the UV-Vis-NIR spectra of Ln bis-phthalocyanines are illustrated in Fig. 2 and 3. The oxidation of Ln(III)bisPcs was exploited by adding iodine in CHCl3 (40 μL, 0.1 M) to 10 mL of a chloroform solution of the Pc (1 × 10−5 M). For Lu complex LuPc(Pox)[Pc′(AB3SH)], the strongest absorption Q band at 695 nm loses intensity and exhibits slightly red shift to 699 nm, also shows that intensity of band at 511 nm and 1045 nm, corresponding to radical π–π*transition, significantly increase. In addition, one electron which causes the intermolecular transition that was prominent in the neutral form complexes, is removed from the complex, all bands corresponding to those transitions disappeared. Same results were observed for Eu complex EuPc(Pox)[Pc′(AB3SH)] as well which are summarized in Table 1. The reduced forms of the compounds were obtained by adding 4 mg of NaBH4 to 10 mL of a solution of the Pc (1 × 10−5 M) in THF under argon atmosphere. The anionic complex (Pc2Lu−) displays slightly blue shifted a splitted Q band absorbing at 651 nm and 732 nm. The intervalence transition is absent due to addition of the second electron by reduction. Moreover, the band at 1045 nm, which is linked to the radical part of the complex, also disappeared by reduction of complex. The electronic absorption spectra of the reduced form of EuPc(Pox)[Pc′(AB3SH)] complex are similar to reduced form of LuPc(Pox)[Pc′(AB3SH)] derivative and the electronic spectral data are summarized in Table 1.
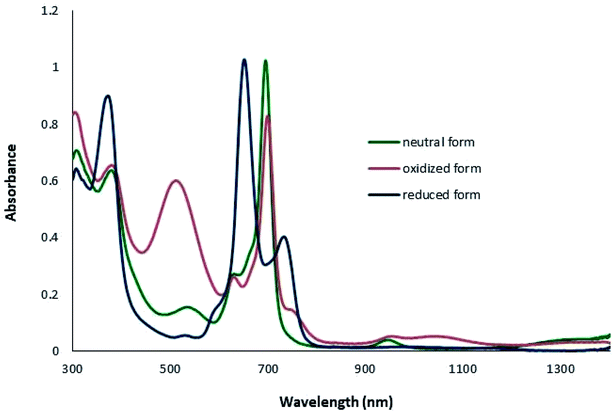 |
| Fig. 2 UV-Vis-NIR spectra of neutral, oxidized and reduced form of LuPc(Pox)[Pc′(AB3SH)] (1 × 10−5 M) in CHCl3. | |
 |
| Fig. 3 UV-Vis-NIR spectra of neutral, oxidized and reduced form of EuPc(Pox)[Pc′(AB3SH)] (1 × 10−5 M) in CHCl3, reduced in THF, oxidation in CHCl3. | |
Table 1 UV-NIR spectral data of neutral, oxidized and reduced forms of Lu and Eu bis-phthalocyanines (1 × 10−5 M). B: Soret band, BV and RV: radical transition bands, ICT: intramolecular charge transfer band
Compound |
Form |
λmax (nm) |
B |
BV |
Q |
RV |
ICT |
LuPc(Pox)[Pc′(AB3SH)] |
Neutral |
380 |
535 |
631, 695 |
943 |
1467–1993 |
Oxidized |
380 |
511 |
630, 699, 760 |
1045 |
— |
Reduced |
372 |
530 |
651, 732 |
— |
— |
EuPc(Pox)[Pc′(AB3SH)] |
Neutral |
378 |
571 |
662, 705 |
936 |
1652–1890 |
Oxidized |
381 |
506 |
706, 755 |
1146 |
|
Reduced |
373 |
550 |
659, 708 |
— |
— |
Photophysics and photochemistry
The photophysical (fluorescence quantum yield) and photochemical (singlet oxygen generation) properties of Bis-Ln-Pc2 were investigated in comparison with unsubstituted zinc(II)phthalocyanine (ZnPc) in DMSO. DMSO was used as a solvent due to nontoxic property of DMSO for biological media. All of the data discussed below are summarized in Table 2.
Table 2 Photophysical and photochemical parameters of mono and bis Ln(III) phthalocyanine complexes
Compound |
λmax (nm) |
log ε |
λex (nm) |
λemmax (nm) |
λexmax (nm) |
ΔλST (nm) |
ΦF |
Indirect ΦΔ in DMSO |
Direct ΦΔ in THF |
LuPcPox(OAc) |
709 |
5.01 |
640 |
717 |
715 |
2 |
0.004 |
0.300 |
0.270 |
LuPc(Pox)[Pc′(AB3SH)] |
695 |
5.02 |
640 |
716 |
707 |
9 |
0.002 |
0.120 |
0.170 |
EuPcPox(OAc) |
709 |
5.22 |
673 |
738 |
736 |
2 |
0.010 |
0.050 |
0.090 |
EuPc(Pox)[Pc′(AB3SH)] |
705 |
5.18 |
665 |
699 |
697 |
2 |
0.005 |
0.007 |
0.014 |
ZnPc |
672 |
5.14 (ref. 63) |
643 |
682 |
673 |
9 |
0.180 (ref. 64) |
0.670 (ref. 65) |
0.530 (ref. 66) |
Photophysical properties
The fluorescence quantum yield (ΦF) is the ratio of photons emitted through fluorescence to number of photons absorbed. The fluorescence quantum yields were determined in DMSO, using a comparative method. The values were all lower than 0.01 for LuPc(Pox)[Pc′(AB3SH)] and EuPc(Pox)[Pc′(AB3SH)] complexes. Even monomer complex derivatives did not show any fluorescence emission in DMSO (Table 2). The absorption, excitation and emission spectra for all complexes are shown in Fig. S47–S50,† respectively. The emission signals for complexes are weak and broad and not comparable to the excitation and absorption spectrum. The low ΦF values for all complexes could be attributed to the high atomic number of lutetium and europium which are coordinating atoms in the cavity of these phthalocyanines may facilitate the intersystem crossing to its excited triplet state after excitation.
Photochemical properties
The singlet oxygen quantum yield (ΦΔ) is described as the number of molecules of singlet oxygen generated per number of photons absorbed by the sensitizer. A number of different techniques for the determination of ΦΔ measurement of efficiency have been developed over the five decades.58–60 In our work, we had applied a direct method in THF. Singlet-oxygen phosphorescence spectra for all compounds excited with a xenon-arc source at their respective absorption maxima were recorded by a near-IR-sensitive detector. Singlet oxygen phosphorescence spectra of Pc derivatives in THF at equal absorbances (0.23) were obtained to directly determine ΦΔ. ΦΔ for lutetium(III) mono LuPcPox(OAc) and bis phthalocyanines LuPc(Pox)[Pc′(AB3SH)] derivatives respectively are 0.27 and 0.17 (Table 2). We determined the singlet oxygen quantum yield 0.17 which is the greatest value in THF for A7B type heteroleptic lutetium(III) bis-phthalocyanine complex to date. Table 2 shows ΦΔ values of lutetium complexes in THF along with mono and bis EuPcPox(OAc) and EuPc(Pox)[Pc′(AB3SH)] derivatives (Fig. 4). The lower ΦΔ values observed for europium complexes were showing inefficient molecular oxygen energy transfer.
 |
| Fig. 4 Singlet oxygen phosphorescence spectra of the Pc derivatives in THF at equal absorbances (0.23) at the maximum absorption wavelength. | |
The ΦΔ values were also evaluated by indirect method in DMSO, a solvent in which the phthalocyanine is not aggregated. No aqueous system can entirely mimic biological media, whose complexity is likely to affect the solubility/aggregation of phthalocyanines. As indirect methods, chemical trapping and O2 consumption methods exist which can be suited for use in both organic and aqueous media.61,62 In our study, the measurements were performed by examining the disappearance of the absorption band of 1,3-diphenylisobenzofuran (DPBF) upon irradiation and subsequent quenching by the singlet oxygen generated was monitored by UV-Vis spectroscopy (Fig. S43–S46†). ΦΔ are higher for mono-lutetium LuPcPox(OAc) and mono-europium EuPcPox(OAc) complexes in DMSO. The higher values for mono complexes could be due to red shifts of the Q-bands of these complexes. There were no changes in the DPBF spectra (Fig. S45 and S46†) even after long period of light exposure in the presence of europium complexes. It indicated that DPBF was not degraded because of lack of singlet oxygen production by these complexes.
Electrochemical determination
Although both complexes, Ln(III) mono and bis-Pcs has the same metal atom but their molecular structures are different. They exhibit different electronic interactions which can affect their voltametric behaviors. In our study, electrochemical characterizations of lanthanide series bis-Pcs having Lu3+ (Bis-LuPc2), Eu3+ (Bis-EuPc2) metal centers have been carried out to investigate the effects of metal center on the electrochemical responses of LnPc2 type complexes. Fig. 5, 6, S51 and S52† represent the CV and SWV responses of mono and their sandwich complexes LuPc(Pox)[Pc′(AB3SH)] and EuPc(Pox)[Pc′(AB3SH)] in DCM/TBAP respectively.
 |
| Fig. 5 (a) CVs of LuPcPox(OAc) at various scan rates on Pt in DCM/TBAP. (b) SWV of LuPcPox(OAc), SWV parameters: pulse size = 100 mV; step size: 5 mV; frequency: 25 Hz. | |
 |
| Fig. 6 (a) CVs of LuPc(Pox)[Pc′(AB3SH)] at various scan rates on Pt in DCM/TBAP. (b) SWV of LuPc(Pox)[Pc′(AB3SH)], SWV parameters: pulse size = 100 mV; step size: 5 mV; frequency: 25 Hz. | |
In Fig. 5 mono phthalocyanine LuPcPox(OAc) gives one oxidation redox couple, O1 at 0.64 V, and two reduction couples, R1 at −0.91 V, R2 at −1.25 V. In Fig. 6, mono EuPcPox(OAc) showed similar electrochemical responses with only one oxidation–reduction couple O1 at 0.77 V, and R1 at −0.86 V. ΔE1/2 values of mono Lu and Eu complexes (1.55 and 1.62 V) reflect the energy band gap which define the energy necessary for the transition of an electron from the highest occupied molecular orbital (HOMO) to the lowest unoccupied molecular orbital (LUMO), are in harmony with those of MPc having redox inactive metal center and mono Lu and Eu Pc complexes.67,68
The Ln(III) bis-Pc2 complexes studied here, their radical structures are well reflected by their electrochemical behaviors since radical complexes are easily reduced or oxidized69 Bis-Lu phthalocyanine LuPc(Pox)[Pc′(AB3SH)] gives two oxidation redox couples, O2 at 0.71 V, O1 at 0.44 V, and four reduction couples, R1 at 0.03 V, R2 at −1.02 V, R3 at −1.31 V and R4 at −1.65 V. Also, Bis-Eu phthalocyanine EuPc(Pox)[Pc′(AB3SH)] gives two oxidation redox couples, O2 at 0.80 V, O1 at 0.62 V, and four reduction couples, R1 at 0.16 V, R2 at −0.95 V, R3 at −1.18 V and R4 at −1.50. Due to the π–π interaction of phthalocyanine rings around the lutetium and europium core in Ln(III) bis-Pc2 complexes, oxidation–reduction peak separation (ΔE1/2) decreased to 0.42 and 0.46 V that easier electron transition reactions between two Pc rings closed to each other.
From CV and SWV data represented on Table 3, it is seen that the effect of the central metal on the redox reactions is reflected by the peak potentials for second and first oxidations and also first reduction peak potentials which are size-sensitive. The highest negative potential shift was observed with 0.03 V for LuPc(Pox)[Pc′(AB3SH)] first reduction. We can conclude, EuPc(Pox)[Pc′(AB3SH)] complex reduction easier than LuPc(Pox)[Pc′(AB3SH)] derivative which is in good agreement with literature and can be explained by the decreasing ΔE1/2 value of Ln(Pc)2 with decreasing rare earth ionic radius.69–71
Table 3 The electrochemical data for mono and bis Ln(III) phthalocyanines. All electrochemical data were given versus SCE in DCM. HOMO and LUMO energy level values determined from experimental cyclic voltammetry (CV)
Complex |
|
O2 |
O1 |
R1 |
R2 |
R3 |
R4 |
dΔE1/2 (V) |
fHOMO (eV) |
gLUMO (eV) |
E1/2 = (Epa + Epc)/2 at 0.100 V s−1. ΔEp = |Epa − Epc|. Ipa/Ipc for reduction, Ipc/Ipa for oxidation processes at 0.100 V s−1 scan rate. ΔE1/2 = E1/2 (first oxidation) − E1/2 (first reduction) = HOMO–LUMO gap for MPc having an electro inactive metal center. This value is derived from SWV. EHOMO = −[(Eox − E1/2(ferrocene)) + 4.8]. ELUMO = −[(Ered − E1/2(ferrocene)) + 4.8]. |
LuPcPox(OAc) |
aE1/2 (V) |
— |
0.64 |
−0.91 |
−1.25 |
— |
— |
1.55 |
−4.97 |
−3.42 |
bΔEp (mV) |
— |
48 |
88 |
31 |
— |
— |
— |
— |
— |
cIpa/Ipc |
— |
0.95 |
0.73 |
0.69 |
— |
— |
— |
— |
— |
LuPc(Pox)[Pc′(AB3SH)] |
aE1/2 (V) |
0.71 |
0.44 |
0.03 |
−1.02 |
−1.31 |
−1.65e |
0.42 |
−4.76 |
−4.35 |
bΔEp (mV) |
— |
130 |
158 |
150 |
161 |
— |
— |
— |
— |
cIpa/Ipc |
— |
0.74 |
0.94 |
0.98 |
0.96 |
— |
— |
— |
— |
EuPcPox(OAc) |
aE1/2 (V) |
— |
0.77 |
−0.86 |
— |
— |
— |
1.62 |
−5.10 |
−3.48 |
bΔEp (mV) |
— |
102 |
57 |
— |
— |
— |
— |
— |
— |
cIpa/Ipc |
— |
0.90 |
0.16 |
— |
— |
— |
— |
— |
— |
EuPc(Pox)[Pc′(AB3SH)] |
aE1/2 (V) |
0.80 |
0.62 |
0.16e |
−0.95 |
−1.18 |
−1.50 |
0.46 |
−4.95 |
−4.49 |
bΔEp (mV) |
— |
136 |
38e |
65 |
63 |
119 |
— |
— |
— |
cIpa/Ipc |
— |
0.88 |
0.89e |
0.83 |
0.96 |
0.77 |
— |
— |
— |
To be able to decide that a redox reaction is reversible, peak potential should not change with scan rate and the ratio of anodic and cathodic peak currents should be equal to 1. The reaction is not reversible if these conditions are not fit. We have more expansive peaks on both anodic and cathodic directions at 500 mV s−1 scan rates (Fig. 5a, 6a, S51a and S52a†). The potential shifts at higher scan rates are indicated that redox reactions have quasi-reversible systems. In the forward scan, formed species occur in the oxidation reactions, and in the backward scan, formed species are observed in the reduction reaction. The reversibility of the complex was also checked with the square wave voltammogram (SWV) given in Fig. 5b, 6b, S51b and S52b.† While the first reduction and the first oxidation couples of all monomeric LnPc complexes are reversible, the second oxidation and reductions are quasi-reversible with respect to ΔEp and Ip,a/Ip,c values. Peak analyses of the complexes could not be performed very well for mono LnPc complexes as expected. We believe that broadening of the reduction processes may be due to the aggregation of the complexes in DCM which is non-polar and non-coordinating solvent. For Ln(III) bis-Pc2 complexes, as shown in Fig. 5a and 6a, not only the oxidation couple O1 and the first reduction couple R1 but also oxidation couple O2 and the second reduction couple R2 are reversible with respect to ΔEp, ΔEp − Ep/2, Ipa/Ipc values. All couples were monitored clearly with CV measurements (Fig. 6a and S52a†) and SWV measurements as well, except R4 couples could not be analyzed properly and thus, were ill-defined with SWV measurements (Fig. S52b†) for EuPc(Pox)[Pc′(AB3SH)] complex.
Evaluating cytotoxicity and determining IC50 values
The cytotoxicity of the A549 and BEAS-2B cell lines incubated with EuPc(Pox)[Pc′(AB3SH)], LuPc(Pox)[Pc′(AB3SH)] and EuPcPox(OAc) with a concentration range of 0.1–50.0 μM were evaluated for a time period of 24 and 48 hours. LuPcPox(OAc) and ZnPc complexes in the same incubation conditions but varying the concentration from 0.01 to 1.00 μM were used for the assessment of cytotoxicity. Fig. 7 represents the cell viabilities of A549 cells for a period of 24 hours. The viability graphs for A549 and BEAS-2B cells after 48 hours of Pc treatment were represented in ESI Part as Fig. S53 and S54.†
 |
| Fig. 7 Cell viabilities of A549 cells incubated with LnPcs in the dark and under the light irradiation. The light dose was 0.036 J cm−2 s−1 for 30 min of irradiation (n = 4). | |
The type of phthalocyanine complexes was found to be significant for the biological response of the A549 cells. The cells were mostly alive (viability > 60%) for EuPc(Pox)[Pc′(AB3SH) regardless of the Pc concentrations and light irradiation. The light irradiation did not increase toxicity of the cells in the presence of EuPc(Pox)[Pc′(AB3SH). When the cells were incubated with LuPc(Pox)[Pc′(AB3SH)] and then irradiated the cell viability was reduced 10-fold with concentration. The effect of irradiation on the viability was insignificant (statistically same) when the cells were incubated with EuPcPox(OAc) . The viability of the cells incubated with ZnPc was gradually decreased with increased concentrations and the light irradiation caused high toxicity in the sub-μM range. The most dramatic effect was observed for LuPcPox(OAc): the cells were severely killed when they were exposed to the light in the presence of LuPcPox(OAc). At 10 nM almost all of the cells were killed. Interestingly, the cells remained alive under the irradiation (the total dose rate of 0.036 J cm−2 s−1) when they were not treated with LuPcPox(OAc) in the concentration range. This finding suggests that the red-light irradiation alone up to 65 J cm−2 is not capable of inducing toxicity in the cells. The cytotoxicity response of the A549 cells incubated with LuPc(Pox)[Pc′(AB3SH)], EuPcPox(OAc) and ZnPc are concentration dependent.
By using these viability data, IC50 values were determined by a nonlinear curve fitting. We used a dose–response sigmoidal fitting (provided in the ESI Fig. S55†) to obtain the IC50 values. The IC50 values were tabulated in Table 4. IC50 graphs were provided in ESI Part as Fig. S56–S65† for both cell lines. EuPc(Pox)[Pc′(AB3SH)] and EuPcPox(OAc) complexes have IC50 values above 25 μM and not changing with the light irradiation. When ZnPc is used the IC50 is about 0.25 μM when the cells are irradiated. The IC50 is reduced to below 0.01 μM for LuPcPox(OAc) with the irradiation. When the A549 cells were treated with LuPcPox(OAc) complex, we microscopically observed that the cells remained on the plate were loosely attached to the plate surfaces (data not provided), suggesting that LuPcPox(OAc) is severely cytotoxic to A549 cells when irradiated with red-light, confirming the IC50 value (<0.01 μM). This is unprecedented for lanthanide phthalocyanines to the best of our knowledge.
Table 4 IC50 values of all Pcs used to treat A549 and BEAS-2B cells and singlet oxygen quantum yields
Compound |
ΦΔ (%) |
A549 |
BEAS-2B |
24 hours |
48 hours |
24 hours |
48 hours |
Indirect in DMSO |
Direct in THF |
Dark |
Light |
Dark |
Light |
Dark |
Light |
Dark |
Light |
LuPcPox(OAc) |
30 |
27 |
1.34 |
<0.01 |
0.93 |
<0.01 |
0.35 |
<0.01 |
0.49 |
<0.01 |
LuPc(Pox)[Pc′(AB3SH)] |
12 |
17 |
59.63 |
7.59 |
44.59 |
8.64 |
51.97 |
3.35 |
44.74 |
3.31 |
EuPcPox(OAc) |
5 |
9 |
44.15 |
27.64 |
58.75 |
47.68 |
33.99 |
39.86 |
19.72 |
16.52 |
EuPc(Pox)[Pc′(AB3SH)] |
0.7 |
1.4 |
136.07 |
60.25 |
39.68 |
42.8 |
32.39 |
42.19 |
42.78 |
45.74 |
ZnPc |
67 |
53 |
0.95 |
0.20 |
0.88 |
0.38 |
0.11 |
<0.01 |
1.28 |
0.28 |
Fig. 8 shows the cytotoxic responses of the BEAS-2B cells in the dark and under the irradiation. The trend for the BEAS-2B cell viability has similar trend compared to the response of the A549 cells, but the BEAS-2B cells are much more sensitive. The BEAS-2B cells were not responding to the light irradiation in the presence of EuPc(Pox)[Pc′(AB3SH)] with concentrations up to 50 μM. However, the viability was significantly reduced with the light when the BEAS-2B cells were incubated with LuPc(Pox)[Pc′(AB3SH)] with concentration above 2.5 μM. ZnPc complex was found to be toxic for the concentrations below 1.0 μM. The irradiation reduced the viability of the BEAS-2B cells when ZnPc with 0.25 μM or above concentration was used. The BEAS-2B cells incubated with LuPc(OAc) complexes were highly sensitive to the light irradiation: the viability reduced well below 0.01 μM of LuPc(OAc) complex used.
 |
| Fig. 8 Cell viabilities of BEAS-2B cells incubated with Pc complexes in the dark and light irradiations. The cells were irradiated with flux of 0.036 J cm−2 s−1 for 30 min (n = 4). | |
The BEAS-2B cells are more sensitive to the light and the phthalocyanines complexes compared to the A549 cells. The IC50 values of BEAS-2B cells are smaller compared to those for the A549 cells and tabulated in the Table 4. The IC50 values for EuPcPox(OAc) and EuPc(Pox)[Pc′(AB3SH)] were determined to be about 33 μM. LuPc(Pox)[Pc′(AB3SH)] and LuPcPox(OAc) were highly toxic compared to Eu-complexes: 0.01 μM of LuPcPox(OAc) with the light was enough for killing almost all the cells. The ZnPc was more toxic for the BEAS-2B cells compared to the A549 cells: 0.11 μM of ZnPc was plentiful for cell death even in the dark (Table 4). It implies that ZnPc may not be considered as a good candidate photosensitizer because it is just toxic in the absence of light. These findings show that the endurance of the normal cells exposed to light is much lower. These results further imply that we need to deliver these Pc-complexes to cancer cells with higher specificity exploring the difference between cancer and normal healthy cells. In general, the expression level of certain receptors such as folate and epidermal growth factor receptors is higher on cancer cell membranes compared to normal healthy cells. This difference may be explored to direct the phthalocyanines complexes conjugating with antibodies or peptides recognizing certain receptors specifically. Thus, more phthalocyanines may be accumulated in cancer cells.
The singlet oxygen generation quantum yields are in good agreement with the viability (MTT assay) findings. LuPcPox(OAc) and ZnPc generate higher amount of singlet oxygen and induce cell death at nanomolar concentrations. While the singlet oxygen generation is measured in silico, it is still a good indicator related to generation of radicals in vitro conditions. Since MTT assay measures mitochondrial activity, suggesting that these complexes producing reactive oxygen species produced by the light may interfere with mitochondrial processes. More work should be conducted to decipher cell death mechanism(s) in the dark and under the light irradiation. Nevertheless, the significance of this work is to present synthesis methods to prepare novel asymmetric heteroleptic Lu(III) complexes as promising photosensitizers that may offer lower concentration and a shorter exposure time with reduced irradiation that make faster and effective phototherapy feasible.
Conclusion
We synthesized novel bis-lanthanide Lu(III) and Eu(III) phthalocyanine complexes as candidate photosensitizers in PDT. The synthetic strategy to get A7B type LuPc(Pox)[Pc′(AB3SH)] and EuPc(Pox)[Pc′(AB3SH)] involved in preparation of one polyoxo group 4,5-bis(4,7,10-trioxaundecan-1-sulfonyl) substituted Pc ring and one unsymmetrical group possessing a single functional thiol reactive substituent. Challenging multi step reactions produced LuPc(Pox)[Pc′(AB3SH)] and EuPc(Pox)[Pc′(AB3SH)] with reaction yields of 33% (0.052 g) and 38% (0.058 g) respectively. These reaction yields encouraged us that these newly synthesized complexes may be considered as attractive species for both fundamental studies and practical applications. The Ln(III) bis-Pc2 sandwich complexes may be classified radicals that are well characterized by electrochemical measurements. We determined that the reduction of EuPc(Pox)[Pc′(AB3SH)] is easier than LuPc(Pox)[Pc′(AB3SH)] because of decreased distance between the Pc rings as a consequence of smaller radius of Eu ions. For photodynamic efficacy, the quantum yields of singlet oxygens generated by LuPc(Pox)[Pc′(AB3SH)], LuPcPox(OAc) and ZnPc are favorable. These complexes induce concentration dependent cell killing and respond to light irradiation reducing the IC50 values down to nanomolar. LuPcPox(OAc) is determined as the best candidate photosensitizer among five phthalocyanine complexes developed in this study.
Conflicts of interest
There are no conflicts of interest to declare.
Acknowledgements
We are thankful to the Scientific and Technological Research Council of Turkey (TÜBİTAK) for financial support (project number: 116Z337).
References
- R. Weiss and J. Fischer, The Porphyrin Handbook, Elsevier Science, San Diego, USA, 2003 Search PubMed.
- K. Kadish, K. M. Smith and R. Guilard, The porphyrin handbook: bioinorganic and bioorganic chemistry, Academic Press, 2000 Search PubMed.
- N. Kobayashi, Coord. Chem. Rev., 2002, 227, 129–152 CrossRef CAS.
- Y. Bian, Y. Zhang, Z. Ou and J. Jiang, Handbook of Porphyrin Science: With Applications to Chemistry, Physics, Materials Science, Engineering, Biology and Medicine, 2011 Search PubMed.
- N. Ishikawa, T. Okubo and Y. Kaizu, Inorg. Chem., 1999, 38, 3173–3181 CrossRef CAS.
- N. Ishikawa, T. Iino and Y. Kaizu, J. Am. Chem. Soc., 2002, 124, 11440–11447 CrossRef CAS.
- N. Ishikawa, T. Iino and Y. Kaizu, J. Phys. Chem. A, 2002, 106, 9543–9550 CrossRef CAS.
- M. M. Nicholson, Phthalocyanines: Properties and Applications, 1993 Search PubMed.
- J. Jiang, K. Kasuga and D. P. Arnold, Supramolecular Photo-sensitive and Electro-active Materials, 2001 Search PubMed.
- M. M. Ayhan, A. Singh, C. Hirel, A. G. Gürek, V. Ahsen, E. Jeanneau, I. Ledoux-Rak, J. Zyss, C. Andraud and Y. Bretonnière, J. Am. Chem. Soc., 2012, 134, 3655–3658 CrossRef CAS.
- H. Al-Sagur, S. Komathi, M. A. Khan, A. G. Gurek and A. Hassan, Biosens. Bioelectron., 2017, 92, 638–645 CrossRef CAS.
- D. K. P. Ng and J. Jiang, Chem. Soc. Rev., 1997, 26, 433–442 RSC.
- W. Huang, H. Xiang, Q. Gong, Y. Huang, C. Huang and J. Jiang, Chem. Phys. Lett., 2003, 374, 639–644 CrossRef CAS.
- S. Casilli, M. De Luca, C. Apetrei, V. Parra, Á. A. Arrieta, L. Valli, J. Jiang, M. L. Rodríguez-Méndez and J. A. De Saja, Appl. Surf. Sci., 2005, 246, 304–312 CrossRef CAS.
- A. C. Tedesco, J. C. Rotta and C. N. Lunardi, Curr. Org. Chem., 2003, 7, 187–196 CrossRef CAS.
- M. Durmuş and T. Nyokong, Photochem. Photobiol. Sci., 2007, 6, 659–668 RSC.
- A. G. Gürek and Ö. Bekaroǧlu, J. Chem. Soc., Dalton Trans., 1994, 1419–1423, 10.1039/DT9940001419.
- A. L. Thomas, Phthalocyanine Research and Applications, Taylor & Francis, 1990 Search PubMed.
- T. Sokolova, T. Lomova, V. Morozov and B. Berezin, Koord. Khim., 1994, 20, 637–640 CAS.
- I. Kalashnikova, S. Nefedov, L. Tomilova and N. Zefirov, Russ. Chem. Bull., 2007, 56, 2426–2432 CrossRef CAS.
- M. Durmuş, S. Yeşilot, B. Çoşut, A. G. Gürek, A. Kılıç and V. Ahsen, Synth. Met., 2010, 160, 436–444 CrossRef.
- R. Zugle, C. Litwinski and T. Nyokong, Polyhedron, 2011, 30, 1612–1619 CrossRef CAS.
- L. Chen, R. Hu, J. Xu, S. Wang, X. Li, S. Li and G. Yang, Spectrochim. Acta, Part A, 2013, 105, 577–581 CrossRef CAS.
- R. Rousseau, R. Aroca and M. Rodriguez-Mendez, J. Mol. Struct., 1995, 356, 49–62 CrossRef CAS.
- J. R. Darwent, P. Douglas, A. Harriman, G. Porter and M.-C. Richoux, Coord. Chem. Rev., 1982, 44, 83–126 CrossRef CAS.
- J. L. Sessler and R. A. Miller, Biochem. Pharmacol., 2000, 59, 733–739 CrossRef CAS.
- S. W. Young, K. W. Woodburn, M. Wright, T. D. Mody, Q. Fan, J. L. Sessler, W. C. Dow and R. A. Miller, Photochem. Photobiol., 1996, 63, 892–897 CrossRef CAS.
- C. Hou, L. Zhao, F. Geng, D. Wang and L.-H. Guo, Anal. Bioanal. Chem., 2016, 408, 8795–8804 CrossRef CAS.
- P. Chen, Y.-F. Huang, G.-Y. Xu, J.-P. Xue and J.-J. Chen, J. Porphyrins Phthalocyanines, 2019, 23, 619–627 CrossRef CAS.
- E. Dube, D. O. Oluwole, N. Nwaji and T. Nyokong, Spectrochim. Acta, Part A, 2018, 203, 85–95 CrossRef CAS.
- L. G. Tomilova, Y. G. Gorbunova, M. L. Rodriguez-Mendez and J. A. De Saja, Mendeleev Commun., 1994, 4, 127–128 CrossRef.
- N. Ishikawa and Y. Kaizu, Chem. Lett., 1998, 27, 183–184 CrossRef.
- N. Ishikawa and Y. Kaizu, J. Phys. Chem. A, 2000, 104, 10009–10016 CrossRef CAS.
- Y. Liu, K. Shigehara, M. Hara and A. Yamada, J. Am. Chem. Soc., 1991, 113, 440–443 CrossRef CAS.
- N. Ishikawa and Y. Kaizu, Coord. Chem. Rev., 2002, 226, 93–101 CrossRef CAS.
- I. Alcón, M. Gonidec, M. R. Ajayakumar, M. Mas-Torrent and J. Veciana, Chem. Sci., 2016, 7, 4940–4944 RSC.
- S. Alpugan, Ü. İşci, F. Albrieux, C. Hirel, A. G. Gürek, Y. Bretonnière, V. Ahsen and F. Dumoulin, Chem. Commun., 2014, 50, 7466–7468 RSC.
- N. Sheng, R. Li, C.-F. Choi, W. Su, D. K. Ng, X. Cui, K. Yoshida, N. Kobayashi and J. Jiang, Inorg. Chem., 2006, 45, 3794–3802 CrossRef CAS.
- D. Pernin, K. Haberroth and J. Simon, J. Chem. Soc., Perkin Trans. 1, 1997, 1265–1266, 10.1039/A701305A.
- S. Kyatskaya, J. R. Galán Mascarós, L. Bogani, F. Hennrich, M. Kappes, W. Wernsdorfer and M. Ruben, J. Am. Chem. Soc., 2009, 131, 15143–15151 CrossRef CAS.
- B. Ballesteros, G. de la Torre, A. Shearer, A. Hausmann, M. Á. Herranz, D. M. Guldi and T. Torres, Chem.–Eur. J., 2010, 16, 114–125 CrossRef CAS.
- M. Urdampilleta, S. Klyatskaya, J. P. Cleuziou, M. Ruben and W. Wernsdorfer, Nat. Mater., 2011, 10, 502–506 CrossRef CAS.
- V. E. Pushkarev, V. V. Kalashnikov, A. Y. Tolbin, S. A. Trashin, N. E. Borisova, S. V. Simonov, V. B. Rybakov, L. G. Tomilova and N. S. Zefirov, Dalton Trans., 2015, 44, 16553–16564 RSC.
- V. E. Pushkarev, L. G. Tomilova and V. N. Nemykin, Coord. Chem. Rev., 2016, 319, 110–179 CrossRef CAS.
- N. Ishikawa and Y. Kaizu, Chem. Phys. Lett., 1993, 203, 472–476 CrossRef CAS.
- M. N. Yaraşir, M. Kandaz, A. Koca and B. Salih, Polyhedron, 2007, 26, 1139–1147 CrossRef.
- S. S. Erdem, I. V. Nesterova, S. A. Soper and R. P. Hammer, J. Org. Chem., 2009, 74, 9280–9286 CrossRef CAS.
- N. Nombona, E. Antunes, C. Litwinski and T. Nyokong, Dalton Trans., 2011, 40, 11876–11884 RSC.
- T. P. Mthethwa, S. Tuncel, M. Durmuş and T. Nyokong, Dalton Trans., 2013, 42, 4922–4930 RSC.
- D. O. Oluwole, A. V. Yagodin, N. C. Mkhize, K. E. Sekhosana, A. G. Martynov, Y. G. Gorbunova, A. Y. Tsivadze and T. Nyokong, Chem.–Eur. J., 2017, 23, 2820–2830 CrossRef CAS.
- Handbook of Porphyrin Science, ed. K. M. Kadish, K. M. Smith and R. Guilard, World Scientific Publishing Company, 2011 Search PubMed.
- T. Nyokong, Z. Gasyna and M. J. Stillman, Inorg. Chem., 1987, 26, 1087–1095 CrossRef CAS.
- A. B. P. Lever, in Advances in Inorganic Chemistry and Radiochemistry, ed. H. J. Emeléus and A. G. Sharpe, Academic Press, 1965, vol. 7, pp. 27–114 Search PubMed.
- M. J. Stillman and T. J. Nyokong, in Phthalocyanines: Properties and Applications, ed. C. C. Leznoff and A. B. P. Lever, VCH, New York, 1996, ch. 9, vol. 4 Search PubMed.
- F.-L. Lu, Polyhedron, 2007, 26, 3939–3946 CrossRef CAS.
- S. Cotton, in Lanthanide and Actinide Chemistry, 2006, pp. 9–22 Search PubMed.
- J. W. Buchler, P. Hammerschmitt, I. Kaufeld and J. Loffler, Chem. Ber., 1991, 124, 2151–2159 CrossRef CAS.
- A. A. Gorman, I. Hamblett, C. Lambert, A. L. Prescott, M. A. J. Rodgers and H. M. Spence, J. Am. Chem. Soc., 1987, 109, 3091–3097 CrossRef CAS.
- M. Niedre, M. S. Patterson and B. C. Wilson, Photochem. Photobiol., 2002, 75, 382–391 CrossRef CAS.
- R. Schmidt, C. Tanielian, R. Dunsbach and C. Wolff, J. Photochem. Photobiol., A, 1994, 79, 11–17 CrossRef CAS.
- W. Spiller, H. Kliesch, D. Wöhrle, S. Hackbarth, B. Röder and G. Schnurpfeil, J. Porphyrins Phthalocyanines, 1998, 2, 145–158 CrossRef CAS.
- I. Kraljić and S. E. Mohsni, Photochem. Photobiol., 1978, 28, 577–581 CrossRef.
- I. Gürol, M. Durmuş, V. Ahsen and T. Nyokong, Dalton Trans., 2007, 3782–3791 RSC.
- P. Jacques and A. M. Braun, Helv. Chim. Acta, 1981, 64, 1800–1806 CrossRef CAS.
- N. A. Kuznetsova, N. S. Gretsova, O. A. Yuzhakova, V. M. Negrimovskii, O. L. Kaliya and E. A. Luk'yanets, Russ. J. Gen. Chem., 2001, 71, 36–41 CrossRef CAS.
- L. Kaestner, M. Cesson, K. Kassab, T. Christensen, P. D. Edminson, M. J. Cook, I. Chambrier and G. Jori, Photochem. Photobiol. Sci., 2003, 2, 660–667 RSC.
- A. B. P. Lever and E. Milaeva, in The Phthalocyanines, Properties and Applications, ed. C. C. Leznoff, Wiley, 1992, p. 162 Search PubMed.
- M. Arıcı, C. Bozoğlu, A. Erdoğmuş, A. L. Uğur and A. Koca, Electrochim. Acta, 2013, 113, 668–678 CrossRef.
- P. Zhu, F. Lu, N. Pan, D. P. Arnold, S. Zhang and J. Jiang, Eur. J. Inorg. Chem., 2004, 510–517 CrossRef CAS.
- E. B. Orman, A. Koca, A. R. Özkaya, İ. Gürol, M. Durmuş and V. Ahsen, J. Electrochem. Soc., 2014, 161, H422 CrossRef CAS.
- V. E. Pushkarev, A. Y. Tolbin, N. E. Borisova, S. A. Trashin and L. G. Tomilova, Eur. J. Inorg. Chem., 2010, 5254–5262 CrossRef CAS.
Footnote |
† Electronic supplementary information (ESI) available. See DOI: 10.1039/d1ra00197c |
|
This journal is © The Royal Society of Chemistry 2021 |
Click here to see how this site uses Cookies. View our privacy policy here.