DOI:
10.1039/D0RA10864B
(Paper)
RSC Adv., 2021,
11, 5947-5957
A Co-MOF-derived Co9S8@NS-C electrocatalyst for efficient hydrogen evolution reaction†
Received
27th December 2020
, Accepted 15th January 2021
First published on 3rd February 2021
Abstract
The exploitation of efficient hydrogen evolution reaction (HER) electrocatalysts has become increasingly urgent and imperative; however, it is also challenging for high-performance sustainable clean energy applications. Herein, novel Co9S8 nanoparticles embedded in a porous N,S-dual doped carbon composite (abbr. Co9S8@NS-C-900) were fabricated by the pyrolysis of a single crystal Co-MOF assisted with thiourea. Due to the synergistic benefit of combining Co9S8 nanoparticles with N,S-dual doped carbon, the composite showed efficient HER electrocatalytic activities and long-term durability in an alkaline solution. It shows a small overpotential of −86.4 mV at a current density of 10.0 mA cm−2, a small Tafel slope of 81.1 mV dec−1, and a large exchange current density (J0) of 0.40 mA cm−2, which are comparable to those of Pt/C. More importantly, due to the protection of Co9S8 nanoparticles by the N,S-dual doped carbon shell, the Co9S8@NS-C-900 catalyst displays excellent long-term durability. There is almost no decay in HER activities after 1000 potential cycles or it retains 99.5% of the initial current after 48 h.
1. Introduction
Due to the energy crisis and environmental problems, sustainable utilization of renewable energy has shown rapid development in the world. As high efficiency and clean energy, hydrogen energy is giving rise to a new dawn in the future energy systems and is well on the way.1,2 Thus, exploring numerous HER electrocatalysts to generate sufficient H2 by water-splitting is an effective and efficient tactic.1,2 It is know that noble metal Pt is by far the most efficient and benchmark HER electrocatalyst; however, it suffers from resource exhaustion, which blocks its widespread applications.3,4 Therefore, various alternative noble-metal-free catalysts, such as transition metal derived nanomaterials (Mo, W, Co, Fe, and Ni),5–10 N, S, P heteroatom-doped carbon materials,11 and their composites,12 have been scrutinized due to their high HER electrocatalytic activities, other important energy storage and conversion features,13 and low cost. Of note, Co(II)-based species are a type of high-profile candidates whose enhanced electrocatalytic activities have recently been noticed.7,8,14,15 Among them, cobalt and sulfide-rich Co9S8 have been applied in both electrocatalytic ORR and various batteries but scarcely in HER due to its low conductivity and easy aggregation.14,15 To improve these defects, encapsulating metal sulfides into N or S doped carbon materials to generate hybrids gave us a profound inspiration.11 The heteroatom-doped carbon materials not only offer improved electrical conductivity, long-term durability and high selectivity but also can be used as shells to protect metal sulfides from aggregation. More specifically, N,S-dual doped carbon materials themselves have been employed as attractive HER electrocatalysts.11 Therefore, combining Co9S8 nanoparticles and N,S-dual doped carbon to prepare new composite materials and further improve their HER electrocatalytic activities is indeed an appealing task.15
In recent years, metal–organic framework (MOF)-derived hybrids are demonstrated as promoting electrocatalysts for energy storage and conversion.16,17 By pyrolysis of MOF precursors, the advanced features of MOFs, such as heteroatom-doped carbon skeletons, active metal nodes, adjustable structure components, robust frameworks, and periodic porosities, can be endowed or transferred into the derived hybrids with advantages toward electrocatalysis.16,17 To promise highly active electrocatalysts, one can also encapsulate small organic molecules containing heteroatoms to introduce and increase multiple active sites. Herein, we incorporated a small thiourea molecule with both N and S into a crystalline Co-MOF (LCU-105)18 to manage multi-component composites for HER. The skeleton ligands of Co-MOF and thiourea offer N,S-dual doped carbon substrates, which can not only favour electrical conductivity and improve potential active sites but can also embed Co(II) species to increase stability. As a result, Co9S8 nanoparticles wrapped in the N,S-dual doped carbon composite (abbreviated as Co9S8@NS-C-900) were fabricated. The Co9S8@NS-C-900 composite displays efficient HER electrocatalytic activities and long-term durability. It showed a small overpotential of −86.4 mV at a 10.0 mA cm−2 current density, a small Tafel slope of 81.1 mV dec−1, and a large exchange current density (J0) of 0.40 mA cm−2, performance comparable to Pt/C.
2. Experimental section
2.1. Chemicals and material characterization
All chemicals were purchased commercially and used as received. The powder X-ray diffraction (PXRD) was obtained on a D/MAX-rA (Rigaku) diffractometer with Cu Kα radiation (λ = 1.542 Å) at a scan rate of 4° min−1. Raman spectrum was collected using Monovista CRS500. X-ray photoelectron spectra (XPS) were collected using ESCALAB Xi+. N2 adsorption experiments were performed on a Micrometrics ASAP 2020M instrument. FT-IR spectra were recorded on a NICOLET 6700F-IR spectrometer using the KBr disc method in the range of 400–4000 cm−1. Electron microscopy measurements were performed on a field-emission scanning electron microscope (SEM, JSM-6360). Transmission electron microscopy (TEM) was performed on a JEM-2100 at 200 kV.
2.2. Syntheses and structure of the Co-MOF precursor
The pure single crystals of the Co-MOF (LCU-105) precursor were synthesized according to our recently reported reference and for the detailed synthesis process see ESI.†18 The pure phase was confirmed by single-crystal X-ray diffraction (Table S1, ESI†) and PXRD (Fig. S2, ESI†) measurements. The Co-MOF structure displays a three-dimensional (3D) microporous framework (see Scheme 1 and Fig. S1†), which can well enclose small organic molecules, such as thiourea, presenting it as an excellent candidate as a precursor to fabricate multi-component composites. The crystallographic data of Co-MOF are listed in Table S1 (ESI†). The synthesis details of Co9S8@NS-C composites can be found in Scheme 1 and are discussed below.
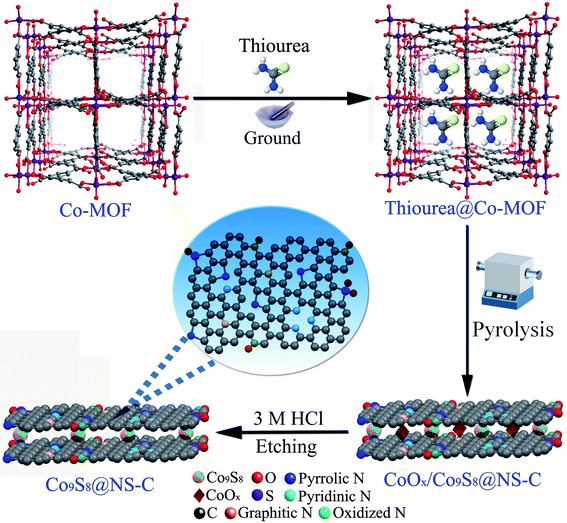 |
| Scheme 1 The fabrication of Co9S8@NS-C composites by pyrolysis and etching. | |
2.3. Fabrication of Co9S8@NS-C composites
The Co9S8@NS-C composites were synthesized using the above Co-MOF as a precursor via the pyrolysis route in Ar atmosphere, followed by the acid etching treatment (Scheme 1): (i) a mixture of crystalline Co-MOF and thiourea was carefully ground in an agate mortar to obtain the thiourea@Co-MOF precursor. (ii) The precursor was transferred to a tube furnace and carbonized under an Ar atmosphere at different temperatures (1000, 900 and 800 °C) for 3 h. The N,S-dual doped carbon composite wrapping both the CoOx and Co9S8 nanoparticles was formed. (iii) The obtained three samples were thoroughly etched with 3 M HCl solutions 3 times to remove the residual CoOx (Scheme 1), and then repeatedly rinsed in ultra-pure water and ethanol. After drying at 60 °C in a vacuum for 8 h, black catalyst powder was synthesized (Scheme 1). These samples are abbreviated as Co-MOF-1000, Co-MOF-900 and Co-MOF-800. The calcined Co-MOF-900 (or named as Co9S8@NS-C-900) sample displays good HER performance and was selected to study its other characteristics.
2.4. Electrochemical measurements
The HER electrocatalytic properties including EIS (electrochemical impedance spectroscopy) were evaluated using the Gamry references 3000 electrochemical workstation. A typical experimental procedure was performed as follows: 2.0 mg of the Co9S8@NS-C-900 composite catalyst (or 20% Pt/C) was dispersed in 1 mL ultra-pure H2O under ultrasound to obtain a homogeneous catalyst ink. Then, 20 μL of the catalyst ink was dropped on the glassy carbon electrode (0.196 cm2). Afterwards, 5 μL of Nafion aqueous solution (0.5 wt%) was dropped on the electrode and naturally air-dried. A three-electrode cell system was worked out using glassy carbon as the working electrode, Ag/AgCl (4 M) as the reference electrode, graphite rod as the counter electrode, and 70 mL KOH (1 M) as the electrolyte. Linear sweep voltammogram (LSV) tests were performed at a scan rate of 10 and 5 mV s−1, respectively. The long-term durability test was performed using chronopotentiometric measurements. All the potentials were calibrated to a reversible hydrogen electrode (RHE) using the equation ERHE = EAg/AgCl + 0.205 V + 0.0591 pH and without an IR-correction.
3. Results and discussions
3.1. Structural characterizations of the Co9S8@NS-C-900 composite
The phase composition of the as-prepared Co9S8@NS-C-900 was detected via PXRD. As shown in Fig. 1a, the characteristic peaks are well assigned to the cubic Co9S8 phase (PDF#73-1442). The peaks at ca. 2θ = 15.43°, 17.84°, 29.80°, 31.81°, 39.52°, 40.61°, 44.66°, 52.06°, 54.62° and 55.5° are ascribed to the (111), (200), (311), (222), (331), (420), (422), (440), (531) and (600) diffraction planes of cubic Co9S8, respectively. Therefore, the Co9S8 nanoparticles embedded in NS-C was confirmed by the PXRD results. Raman spectra (Fig. 1b) of the Co9S8@NS-C-900 catalyst also displayed the characteristic C–C absorption signals at 1449 and 1624 cm−1, indexing to D (defects or amorphous of graphitic carbon) and G (ordered sp2 bonded graphitic carbon) bands of carbon materials, respectively. Therefore, the small ID/IG confirms that NS-C is partially graphitized and generates multiple defects as active sites by N,S-dual doping modification.19a These features of carbon-based catalysts may not only effectively improve the electronic conductivity and anti-corrosion, but also enhance the electrocatalytic efficiency.11,12 Moreover, a broad peak with a maximum value at 2573 cm−1 further proved the evidence of the 2D N,S-dual doped carbon materials. The peaks at 863, 1165 and 1300 cm−1 can be attributed to the Co9S8 species.19b,c,d Raman spectroscopy results also further demonstrate the formation of the Co9S8@NS-C-900 composite. The chemical model of Co9S8 by the combination of PXRD and Raman is demonstrated in Fig. 1b.
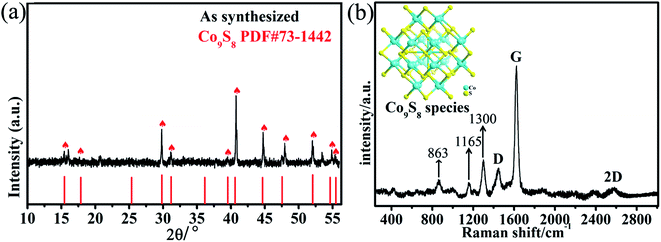 |
| Fig. 1 The PXRD pattern (a) and (b) Raman spectrum of the Co9S8@NS-C-900 composite (inset: the chemical model of Co9S8). | |
As the porous framework of Co-MOF can be easily transmitted to its derived material, the porous feature of the Co9S8@NS-C-900 composite was evaluated by N2 adsorption studies (Fig. 2a). The N2 adsorption capacity rises rapidly at the beginning, afterward it grows slowly to reach a maximum of 90.7 cm3 g−1. The isotherm displays a clear reversible adsorption behaviour, which shows a typical type-I porous feature.20 The corresponding BET (Brunner–Emmet–Teller) was calculated as ca. 239 m2 g−1 using the above N2 adsorption data.20 This high BET surface area supplies the exposure of additional active sites, which are beneficial for the HER activity. The diameters of pores are between 4.4 and 19 Å, and the pore maximum is at 7.9 Å, indicating the easy transportation of H2 in the electrocatalytic process appropriately.
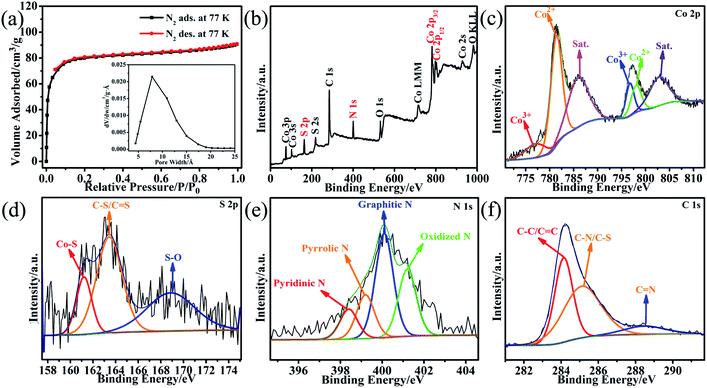 |
| Fig. 2 (a) The N2 sorption isotherm (inset: the pore size distribution). (b) The XPS survey spectrum. High-resolution XPS: (c) Co 2p. (d) S 2p. (e) N 1s. (f) C 1s of the Co9S8@NS-C-900 composite. | |
The chemical composition of the Co9S8@NS-C-900 composite was further checked via XPS (Fig. 2b–f). The survey spectrum displays that the composite consists of Co, S, N, C and O elements (Fig. 2b). The Co 2p high-resolution spectrum after fitting displays that it comprises four typical characteristic peaks (Fig. 2c): the peaks at 777.04 and 796.75 eV are assigned to Co3+; the peaks at 781.40 and 798.03 eV are ascribed to Co2+. However, the peaks at 785.94 eV and 802.97 are indexed to satellite peaks.21,22 The S 2p high-resolution spectrum is deconvoluted into three peaks at 161.20, 163.44 and 168.86 eV (Fig. 2d), assigning to Co–S, C–S/C
S, and C–S–O, respectively.21,22 These peaks also verify the existence of the Co9S8 and N,S-dual doped carbon substance. The N 1s high-resolution spectrum contains four peaks (Fig. 2e). The peak at 398.4 eV is assigned to the pyridinic-N species, showing the formation of CoNx active sites. The peak located at 399.2 eV corresponds to the pyrrolic-N species with another mode of CoNx active sites. The peak at 400.1 eV is indexed to the graphitic-N species. The peak located at 401.2 eV is regarded as the oxidized-N species.21,22 According to the literature, both pyridinic-N and pyrrolic-N can effectively regulate HER active sites.21,22 The C 1s high-resolution spectrum is also divided into three peaks located at 284.14, 285.16 and 288.46 eV (Fig. 2f), illustrating the coexistence of C
C/C–C, C–S/C–N, and C
N, respectively.21,22 These XPS analyses are in agreement with the above PXRD and Raman results.19a Such CoNx and N,S-dual-doped carbon often offer plentiful electrochemically active sites for HER and are further responsive to better HER performance and long-term durability.7,8,15,21,22
FT-IR was performed to identify the chemical bonds and groups present in the Co9S8@NS-C-900 composite, and it confirmed the above compositional analysis results. As shown in Fig. S4 (ESI†), the weak peak at ca. 456 cm−1 and the moderate peak at 580 cm−1 are ascribed to Co–S and Co–N bonds, indicating the existence of Co9S8 and CoNx active sites in the catalyst, respectively. A broad peak at 1039 cm−1 is probably the overlap bending vibrations of C–N, N–H and C–S bonds. The peak at 1402 cm−1 is ascribed to C–N and C–H groups. The spike peak at 1630 cm−1 and the broad strong peak at 3422 cm−1 are both identified as the bending vibrations of N–H bonds. The small peaks at 2848 and 2915 cm−1 are ascribed to the C–H bonds stretching vibrations.19d,23 These results all confirm the formation of the Co9S8@NS-C-900 composite by encapsulating Co9S8 nanoparticles in the porous N,S-dual doped carbon substrate.
3.2. Morphological analysis of the Co9S8@NS-C-900 composite
The morphology and microstructure of the Co9S8@NS-C-900 composite were evaluated using SEM and TEM, respectively. The SEM morphology of the Co9S8@NS-C-900 composite displays interconnected aggregates composed of nanoflakes, and their sizes were within about 10–40 μm (Fig. 3a–c). These carbon nanoflakes intertwine each other to generate porous aggregates, providing transport channels for HER. From Fig. 3c, it can be seen that Co9S8 nanoparticles are clearly embedded into the N,S-dual-doped nanoflakes. Furthermore, the EDS mapping proves the coexistence of Co, S, N and C elements in these carbon nanoflakes (Fig. 3d), and they are uniformly doped in the entire carbon structure. From the EDS mapping of Co and S elements, Co9S8 nanoparticles can be brightly shown by highlights. TEM was also performed to further characterize the detailed structural features of the as-obtained Co9S8@NS-C-900 composite (Fig. 4a–i). It can be observed that highly crystalline block-like Co9S8 nanoparticles are wrapped by the amorphous N,S-dual-doped carbon nanoflakes (Fig. 4b). High-resolution TEM images also indicate that the composite co-exists in two types of planes: one is the Co9S8 crystalline phase and the other is the N,S-dual doped carbon amorphous phase (Fig. 4c,d). From Fig. 4c and d, a characteristic lattice plane distance ca. 0.497 nm was observed, which can be ascribed to the (200) plane of the cubic Co9S8 phase.14,15 This analysis coincides with PXRD results. Moreover, from the HAADF (high-angle annular dark-field) image and EDS mappings displayed in Fig. 4e–i, it can be seen that Co, S, N and C elements are uniformly distributed in the N,S-dual-doped carbon nanoflakes. From both HAADF image and EDS mappings of Co and S elements (Fig. 4e–g), Co9S8 nanoparticles are also clearly visible by highlights. Based on SEM and TEM results, it can be concluded that Co9S8 nanoparticles are successfully embedded in N,S-dual-doped carbon nanoflakes.
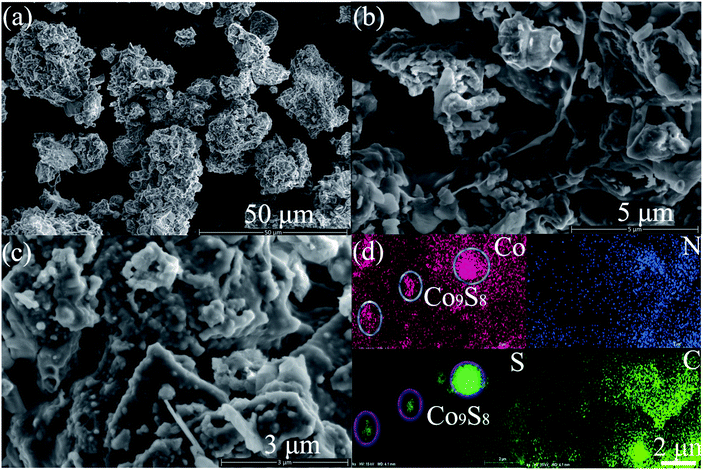 |
| Fig. 3 (a–c) The SEM images of the Co9S8@NS-C-900 composite under different magnifications. (d) The EDS mapping images of selected regions: Co, S, N, and C. | |
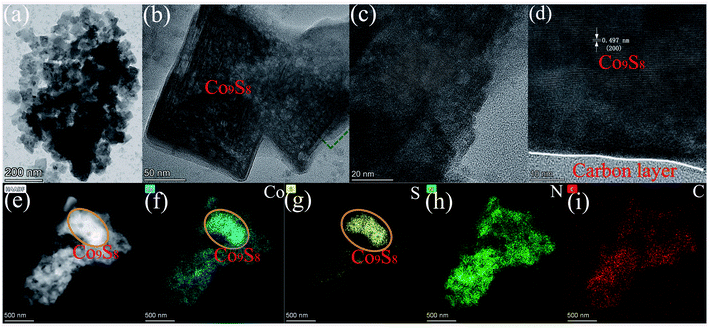 |
| Fig. 4 (a–d) The TEM images of the Co9S8@NS-C-900 composite under different magnifications. (e–i) The HAADF and EDS mapping images of selected regions: Co, S, N, and C. | |
3.3. HER electrocatalytic activity of the Co9S8@NS-C-900 composite
As the porous feature of the Co9S8@NS-C-900 composite is suitable for electrolyte transportation, HER electrocatalytic activities of the as-prepared sample were tested in the KOH (1 M) solution. HER polarization curves of various catalysts (including benchmark 20% Pt/C, Co-MOF-1000, Co-MOF-900 and Co-MOF-800) were obtained from LSV (5 mV s−1). From Fig. 5a, it can be seen that Co9S8@NS-C-900 shows the smallest onset potential (Eonset) of ca. −4.8 mV at 1 mA cm−2 current density, smaller than that of Co-MOF-1000 (Eonset = −54 mV) and Co-MOF-800 (Eonset = −108 mV), and close to Pt/C (Eonset = 0 mV). To deliver a 10.0 mA cm−2 current density, the operating overpotential of Co9S8@NS-C-900 is −86.4 mV. This is lower than that for Co-MOF-1000 (−176 mV), Co-MOF-800 (−292 mV), several reported noble-metal-free HER catalysts,5–12,15,17 and also close to most Pt/C catalysts (−41.6 mV). To evaluate the HER kinetics of these catalysts for comparing with the benchmark Pt/C, Tafel slopes were extracted from the LSV curves. From Fig. 5b, it can be seen that the Tafel slopes obey the following sequence: Pt/C (44.8 mV dec−1) < Co9S8@NS-C-900 (81.1 mV dec−1) < Co-MOF-1000 (116.3 mV dec−1) < Co-MOF-800 (182.2 mV dec−1), which is consistent with the results from Fig. 5a. The Co9S8@NS-C-900 composite has the smallest Tafel slope among the three Co-MOF-derived catalysts and is close to that of Pt/C, showing efficient HER kinetics. The Tafel value of Co9S8@NS-C-900 also confirms that the HER mechanism abides by a Volmer–Heyrovský pathway, which means that the HER rate-determining progress depends on H2 desorption by Hads reacting with H3O+. The HER inherent activities of these catalysts were evaluated by the exchange current densities (J0) extracted from the Tafel slopes by applying the extrapolation method. The J0 value of 0.40 mA cm−2 for Co9S8@NS-C-900 outperforms the values of 0.30 mA cm−2 for Co-MOF-1000, and 0.26 mA cm−2 for Co-MOF-800, and only a little lower than that of Pt/C with the value of 0.54 mA cm−2, but the value is higher than that for most of the reported noble-metal-free HER catalysts.5–12,15,17 Therefore, the Co9S8@NS-C-900 catalyst has a small overpotential, a small Tafel slope and a large J0 value, which was integrated to evaluate its efficient electrocatalytic activity towards HER. The comparison of the HER activity for some cobalt-based materials reported in literature is listed in Table S2 (ESI†). From the table, the HER catalytic performance of Co9S8@NS-C-900 is better than that of most of similar Co9S8, and its composite catalysts under alkaline conditions. It can also match most of the other cobalt-based HER catalysts, but is lower than that for some high-performance materials.
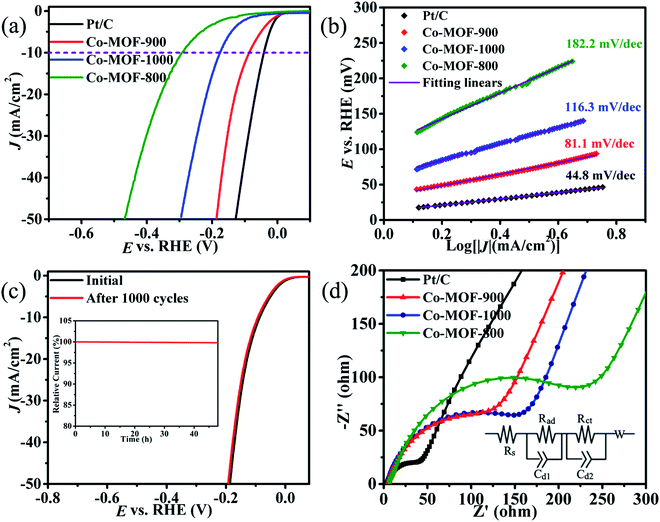 |
| Fig. 5 (a) LSV curves of three Co-MOF-derived materials and Pt/C (1 M KOH). (b) Tafel slopes were obtained from the LSV curves of three Co-MOF-derived catalysts and Pt/C. (c) LSV plots of the Co9S8@NS-C-900 catalyst before and after 1000 cycles (inset: the i–t (current–time) chronoamperometry plot). (d) EIS plots of three Co-MOF derived catalysts and 20% Pt/C (inset: simulated equivalent circuit). | |
Long-term durability is a key factor to evaluate the catalyst performance. Therefore, the cyclability of the Co9S8@NS-C-900 catalyst was tested. In Fig. 5c, the Co9S8@NS-C-900 catalyst displays excellent cycling stability, and there is almost no loss in the HER activity after 1000 potential cycles. Furthermore, the long-term durability of the Co9S8@NS-C-900 catalyst was also evaluated by chronoamperometric measurements. As displayed in the i–t (current–time) plot (Fig. 5c, inset), the Co9S8@NS-C-900 catalyst retains 99.5% of the initial current after 48 h, also indicating that it exhibits excellent cycling stability in the KOH solution. Furthermore, the EIS of three Co-MOF-derived catalysts and Pt/C were also tested to estimate their catalytic kinetics. From Fig. 5d, Nyquist curves are presented for these electrocatalysts. We know that Nyquist radius could respond to Rct (charge transfer resistance) and can effectively estimate the electrocatalytic activities. From Fig. 5d, 20% Pt/C has the smallest radius among all catalysts, which is consistent with its best electrocatalytic activity as a commonly accepted benchmark of HER. In three Co-MOF-derived catalysts, the Co9S8@NS-C-900 catalyst has the smallest radius than that of others and a little larger than that of Pt/C, which displayed slightly worse HER performance than Pt/C and the best HER electrocatalytic performance among three Co-MOF-derived species. The simulated equivalent circuit (Fig. 5d inset) was also built by fitting the ESI data, and it is composed of three resistances: the solution resistance (Rs), hydrogen adsorption resistance (Rad), and Rct. Due to the same equipment and outer solutions, Rs and Rad are supposed to be quite similar. Rct of Co-MOF-1000, Co-MOF-900, and Co-MOF-800 are 361.2, 145.8 and 663.2 Ω, respectively. The smallest Rct of the Co-MOF-900 electrode among them suggests its fastest reaction kinetics during the HER process. All told, the Co9S8@NS-C-900 composite is an efficient HER catalyst possessing excellent cycling stabilities and superior electrocatalytic activities.
4. Conclusion
In conclusion, a porous Co9S8@NS-C-900 composite was fabricated by the pyrolysis of crystalline Co-MOF involving thiourea. The N,S-dual-doped carbon shell can protect Co9S8 nanoparticles from corrosion and aggregation by encapsulating them, and further prompt the stability and conductivity of the whole electrocatalyst. Therefore, benefiting from the synergistic interaction of Co9S8 nanoparticles and N,S-dual-doped carbon substrate, the Co9S8@NS-C-900 composite exhibits efficient electrocatalytic activities and long-term durability towards HER in alkaline electrolytes. In particular, the electrocatalyst requires a small overpotential of −86.4 mV at a 10.0 mA cm−2 current density, a small Tafel slope of 81.1 mV dec−1, and a large exchange current density (J0) of 0.40 mA cm−2, representing a promising noble-free-metal electrocatalyst that approaches the performance of the state-of-the-art Pt/C electrocatalyst.
Author contributions
Yun-Wu Li: conceptualization, investigation, funding acquisition, writing-original draft. Qian Wu: data curation, project administration. Rui-Cong Ma: project administration, validation. Xiao-Qi Sun: investigation, data curation. Dan-Dan Li: formal analysis, resources. Hong-Mei Du: methodology, writing-review & editing. Hui-Yan Ma: supervision, conceptualization, visualization, writing-review & editing. Da-Cheng Li: writing-review & editing. Su-Na Wang: supervision, validation, funding acquisition, writing-review & editing. Jian-Min Dou: conceptualization, writing-review & editing. All the authors gave their final approval for publication.
Conflicts of interest
The authors declare that they have no known competing financial interests or personal relationships that could have appeared to influence the work reported in this paper.
Acknowledgements
This work was financially supported by the National Natural Science Foundation of China (21771095, 21571092 and 21601079), the Natural Science Foundation of Shandong Province (ZR2017JL013), and the Youth Innovation Team of Shandong Colleges and Universities (2019KJC027).
References
-
(a) Z. W. Seh, J. Kibsgaard, C. F. Dickens, I. Chorkendorff, J. K. Norskov and T. F. Jaramillo, Combining theory and experiment in electrocatalysis: Insights into materials design, Science, 2017, 355, eaad4998 CrossRef
;
(b) Y. Zheng, Y. Jiao, A. Vasileff and S. Z. Qiao, The Hydrogen Evolution Reaction in Alkaline Solution: From Theory, Single Crystal Models, to Practical Electrocatalysts, Angew. Chem., Int. Ed., 2018, 57, 7568–7579 CrossRef CAS
;
(c) W. J. Jiang, T. Tang, Y. Zhang and J. S. Hu, Synergistic Modulation of Non-Precious-Metal Electrocatalysts for Advanced Water Splitting, Acc. Chem. Res., 2020, 53, 1111–1123 CrossRef CAS
;
(d) C. L. Hu, L. Zhang and J. L. Gong, Recent progress made in the mechanism comprehension and design of electrocatalysts for alkaline water splitting, Energy Environ. Sci., 2019, 12, 2620–2645 RSC
;
(e) C. Wei, R. R. Rao, J. Y. Peng, B. T. Huang, I. E. L. Stephens, M. Risch, Z. C. J. Xu and S. H. Yang, Recommended Practices and Benchmark Activity for Hydrogen and Oxygen Electrocatalysis in Water Splitting and Fuel Cells, Adv. Mater., 2019, 31, 1806296 CrossRef
;
(f) J. Zhang, Q. Y. Zhang and X. L. Feng, Support and Interface Effects in Water-Splitting Electrocatalysts, Adv. Mater., 2019, 31, 1808167 CrossRef
. -
(a) J. Zhu, L. S. Hu, P. X. Zhao, L. Y. Suk Lee and K. Y. Wong, Recent Advances in Electrocatalytic Hydrogen Evolution Using Nanoparticles, Chem. Rev., 2020, 120, 851–918 CrossRef CAS
;
(b) C. G. Morales-Guio, L. A. Stern and X. L. Hu, Nanostructured hydrotreating catalysts for electrochemical hydrogen evolution, Chem. Soc. Rev., 2014, 43, 6555–6569 RSC
;
(c) J. A. Trindell, Z. Y. Duan, G. Henkelman and R. M. Crooks, Well-Defined Nanoparticle Electrocatalysts for the Refinement of Theory, Chem. Rev., 2020, 120, 814–850 CrossRef CAS
;
(d) T. Y. Wang, H. Xie, M. J. Chen, A. D'Aloia, J. Choc, G. Wu and Q. Li, Precious metal-free approach to hydrogen electrocatalysis for energy conversion: From mechanism understanding to catalyst design, Nano Energy, 2017, 42, 69–89 CrossRef CAS
;
(e) X. C. Du, J. W. Huang, J. J. Zhang, Y. C. Yan, C. Y. Wu, Y. Hu, C. Y. Yan, T. Y. Lei, W. Chen, C. Fan and J. Xiong, Modulating Electronic Structures of Inorganic Nanomaterials for Efficient Electrocatalytic Water Splitting, Angew. Chem., Int. Ed., 2019, 58, 4484–4502 CrossRef CAS
;
(f) B. Ginovska-Pangovska, A. Dutta, M. L. Reback, J. C. Linehan and W. J. Shaw, Beyond the active site: the impact of the outer coordination sphere on electrocatalysts for hydrogen production and oxidation, Acc. Chem. Res., 2014, 47, 2621–2630 CrossRef CAS
. -
(a) Z. X. Fan and H. Zhang, Template Synthesis of Noble Metal Nanocrystals with Unusual Crystal Structures and Their Catalytic Applications, Acc. Chem. Res., 2016, 49, 2841–2850 CrossRef CAS
;
(b) L. Zhang, K. Doyle-Davis and X. L. Sun, Pt-Based Electrocatalysts with High Atom Utilization Efficiency: From Nanostructures to Single Atoms, Energy Environ. Sci., 2019, 12, 492–517 RSC
;
(c) Y. J. Li, Y. J. Sun, Y. N. Qin, W. Y. Zhang, L. Wang, M. C. Luo, H. Yang and S. J. Guo, Recent Advances on Water Splitting Electrocatalysis Mediated by Noble-Metal-Based Nanostructured Materials, Adv. Energy Mater., 2020, 10, 1903120 CrossRef CAS
;
(d) Y. X. Du, H. T. Sheng, D. Astruc and M. Z. Zhu, Atomically Precise Noble Metal Nanoclusters as Efficient Catalysts: A Bridge between Structure and Properties, Chem. Rev., 2020, 120, 526–622 CrossRef CAS
. -
(a) J. Kim, H. Kim, W. J. Lee, B. Ruqia, H. Baik, H. S. Oh, S. M. Paek, H. K. Lim, C. H. Choi and S. I. Choi, Theoretical and Experimental Understanding of Hydrogen Evolution Reaction Kinetics in Alkaline Electrolytes with Pt-Based Core-Shell Nanocrystals, J. Am. Chem. Soc., 2019, 141, 18256–18263 CrossRef CAS
;
(b) Q. Yang, G. W. Li, K. Manna, F. R. Fan, C. Felser and Y. Sun, Topological Engineering of Pt-Group-Metal-Based Chiral Crystals toward High-Efficiency Hydrogen Evolution Catalysts, Adv. Mater., 2020, 32, 1908518 CrossRef CAS
;
(c) J. Park, S. Lee, H. E. Kim, A. Cho, S. Kim, Y. Ye, J. W. Han, H. Lee, J. H. Jang and J. Lee, Investigation of the Support Effect in Atomically Dispersed Pt on WO3−x for Utilization of Pt in the Hydrogen Evolution Reaction, Angew. Chem., Int. Ed., 2019, 58, 16038–16042 CrossRef CAS
;
(d) A. Alinezhad, L. Gloag, T. M. Benedetti, S. Cheong, R. F. Webster, M. Roelsgaard, B. B. Iversen, W. Schuhmann, J. J. Gooding and R. D. Tilley, Direct Growth of Highly Strained Pt Islands on Branched Ni Nanoparticles for Improved Hydrogen Evolution Reaction Activity, J. Am. Chem. Soc., 2019, 141, 16202–16207 CrossRef CAS
;
(e) J. P. Ji, Y. P. Zhang, L. B. Tang, C. Y. Liu, X. H. Gao, M. H. Sun, J. H. Zheng, M. Ling, C. D. Liang and Z. Lin, Platinum single-atom and cluster anchored on functionalized MWCNTs with ultrahigh mass efficiency for electrocatalytic hydrogen evolution, Nano Energy, 2019, 63, 103849 CrossRef CAS
. -
(a) D. Zhao, K. Sun, W. C. Cheong, L. R. Zheng, C. Zhang, S. J. Liu, X. Cao, K. L. Wu, Y. Pan, Z. W. Zhuang, B. T. Hu, D. S. Wang, Q. Peng, C. Chen and Y. D. Li, Synergistically Interactive Pyridinic–N–MoP Sites: Identified Active Centers for Enhanced Hydrogen Evolution in Alkaline Solution, Angew. Chem., Int. Ed., 2020, 59, 8982–8990 CrossRef CAS
;
(b) C. Huang, X. W. Miao, C. R. Pi, B. Gao, X. M. Zhang, P. Qin, K. F. Huo, X. Peng and P. K. Chu, Mo2C/VC heterojunction embedded in graphitic carbon network: An advanced electrocatalyst for hydrogen evolution, Nano Energy, 2019, 60, 520–526 CrossRef CAS
;
(c) F. Li, G. F. Han, H. J. Noh, Y. L. Lu, J. Xu, Y. F. Bu, Z. P. Fu and J. B. Baek, Construction of Porous Mo3P/Mo Nanobelts as Catalysts for Efficient Water Splitting, Angew. Chem., Int. Ed., 2018, 57, 14139–14143 CrossRef CAS
;
(d) Z. X. Sun, M. F. Yang, Y. W. Wang and Y. H. Hu, Novel Binder-Free Three-Dimensional MoS2-Based Electrode for Efficient and Stable Electrocatalytic Hydrogen Evolution, ACS Appl. Energy Mater., 2019, 2, 1102–1110 CrossRef CAS
;
(e) J. S. Li, Y. Wang, C. H. Liu, S. L. Li, Y. G. Wang, L. Z. Dong, Z. H. Dai, Y. F. Li and Y. Q. Lan, Coupled molybdenum carbide and reduced graphene oxide electrocatalysts for efficient hydrogen evolution, Nat. Commun., 2016, 7, 11204 CrossRef CAS
;
(f) D. Z. Wang, D. Z. Zhang, C. Y. Tang, P. Zhou, Z. Z. Wu and B. Z. Fang, Hydrogen evolution catalyzed by cobalt-promoted molybdenum phosphide nanoparticles, Catal. Sci. Technol., 2016, 6, 1952–1956 RSC
;
(g) Z. Z. Wu, C. Y. Tang, P. Zhou, Z. H. Liu, Y. S. Xu, D. Z. Wang and B. Z. Fang, Enhanced hydrogen evolution catalysis from osmotically swollen ammoniated MoS2, J. Mater. Chem. A, 2015, 3, 13050–13056 RSC
. -
(a) M. A. Lukowski, A. S. Daniel, C. R. English, F. Meng, A. Forticaux, R. J. Hamers and S. Jin, Highly active hydrogen evolution catalysis from metallic WS2 nanosheets, Energy Environ. Sci., 2014, 7, 2608–2613 RSC
;
(b) L. Cheng, W. J. Huang, Q. F. Gong, C. H. Liu, Z. Liu, Y. G. Li and H. J. Dai, Ultrathin WS2 Nanoflakes as a High-Performance Electrocatalyst for the Hydrogen Evolution Reaction, Angew. Chem., Int. Ed., 2014, 53, 7860–7863 CrossRef CAS
;
(c) H. J. Yan, C. G. Tian, L. Wang, A. P. Wu, M. C. Meng, L. Zhao and H. G. Fu, Phosphorus-Modified Tungsten Nitride/Reduced Graphene Oxide as a High-Performance, Non-Noble-Metal Electrocatalyst for the Hydrogen Evolution Reaction, Angew. Chem., Int. Ed., 2015, 54, 6325–6329 CrossRef CAS
;
(d) Y. Y. Ma, Z. L. Lang, L. K. Yan, Y. H. Wang, H. Q. Tan, K. Feng, Y. J. Xia, J. Zhong, Y. Liu, Z. H. Kang and Y. G. Li, High efficient hydrogen evolution triggered by a multi-interfacial Ni/WC hybrid electrocatalyst, Energy Environ. Sci., 2018, 11, 2114–2123 RSC
;
(e) Z. G. Chen, W. B. Gong, S. Cong, Z. Wang, G. Song, T. Pan, X. Q. Tang, J. Chen, W. B. Lu and Z. G. Zhao, Eutectoid-structured WC/W2C heterostructures: A new platform for long-term alkaline hydrogen evolution reaction at low overpotentials, Nano Energy, 2020, 68, 104335 CrossRef CAS
. -
(a) S. Anantharaj and V. Aravindan, Developments and Perspectives in 3d Transition-Metal-Based Electrocatalysts for Neutral and Near-Neutral Water Electrolysis, Adv. Energy Mater., 2019, 9, 1902666 Search PubMed
;
(b) V. S. Thoi, Y. J. Sun, J. R. Long and C. J. Chang, Complexes of earth-abundant metals for catalytic electrochemical hydrogen generation under aqueous conditions, Chem. Soc. Rev., 2013, 42, 2388–2400 RSC
;
(c) Y. M. Shi and B. Zhang, Recent advances in transition metal phosphide nanomaterials: synthesis and applications in hydrogen evolution reaction, Chem. Soc. Rev., 2016, 45, 1529–1541 RSC
;
(d) Y. N. Guo, T. Park, J. W. Yi, J. Henzie, J. Kim, Z. L. Wang, B. Jiang, Y. Bando, Y. Sugahara, J. Tang and Y. Yamauchi, Nanoarchitectonics for Transition-Metal-Sulfide-Based Electrocatalysts for Water Splitting, Adv. Mater., 2019, 31, 1807134 CrossRef
;
(e) J. H. Wang, W. Cui, Q. Liu, Z. C. Xing, A. M. Asiri and X. P. Sun, Recent Progress in Cobalt-Based Heterogeneous Catalysts for Electrochemical Water Splitting, Adv. Mater., 2016, 28, 215–230 CrossRef CAS
. -
(a) Y. R. Zheng, P. Wu, M. R. Gao, X. L. Zhang, F. Y. Gao, H. X. Ju, R. Wu, Q. Gao, R. You, W. X. Huang, S. J. Liu, S. W. Hu, J. F. Zhu, Z. Y. Li and S. H. Yu, Doping induced structural phase transition in cobalt diselenide enables enhanced hydrogen evolution catalysis, Nat. Commun., 2018, 9, 2533 CrossRef
;
(b) X. Zou, X. Huang, A. Goswami, R. Silva, B. R. Sathe, E. Mikmekova and T. Asefa, Cobalt-Embedded Nitrogen-Rich Carbon Nanotubes Efficiently Catalyze Hydrogen Evolution Reaction at All pH Values, Angew. Chem., Int. Ed., 2014, 53, 4372–4376 CrossRef CAS
;
(c) J. X. Feng, S. Y. Tong, Y. X. Tong and G. R. Li, Pt-like Hydrogen Evolution Electrocatalysis on PANI/CoP Hybrid Nanowires by Weakening the Shackles of Hydrogen Ions on the Surfaces of Catalysts, J. Am. Chem. Soc., 2018, 140, 5118–5126 CrossRef CAS
;
(d) C. Tang, R. Zhang, W. B. Lu, L. B. He, X. E. Jiang, A. M. Asiri and X. P. Sun, Fe-Doped CoP Nanoarray: A Monolithic Multifunctional Catalyst for Highly Efficient Hydrogen Generation, Adv. Mater., 2017, 29, 1602441 CrossRef
;
(e) T. Liu, P. Li, N. Yao, G. Z. Cheng, S. L. Chen, W. Luo and Y. D. Yin, CoP-Doped MOF-Based Electrocatalys for pH-Universal Hydrogen Evolution Reaction, Angew. Chem., Int. Ed., 2019, 58, 4679–4684 CrossRef CAS
. -
(a) K. Srinivas, Y. J. Lu, Y. F. Chen, W. L. Zhang and D. X. Yang, FeNi3-Fe3O4 Heterogeneous Nanoparticles Anchored on 2D MOF Nanosheets/1D CNT Matrix as Highly Efficient Bifunctional Electrocatalysts for Water Splitting, ACS Sustainable Chem. Eng., 2020, 8, 3820–3831 CrossRef CAS
;
(b) D. Y. Chung, S. W. Jun, G. Yoon, H. Kim, J. M. Yoo, K. S. Lee, T. Kim, H. Shin, A. K. Sinha, S. G. Kwon, K. Kang, T. Hyeon and Y. E. Sung, Large-Scale Synthesis of Carbon-Shell-Coated FeP Nanoparticles for Robust Hydrogen Evolution Reaction Electrocatalyst, J. Am. Chem. Soc., 2017, 139, 6669–6674 CrossRef CAS
;
(c) X. H. Fan, F. T. Kong, A. G. Kong, A. L. Chen, Z. Q. Zhou and Y. K. Shan, Covalent Porphyrin Framework-Derived Fe2P@Fe4N-Coupled Nanoparticles Embedded in N-Doped Carbons as Efficient Trifunctional Electrocatalysts, ACS Appl. Mater. Interfaces, 2017, 9, 32840–32850 CrossRef CAS
;
(d) J. Jin, J. Yin, H. B. Liu, M. Lu, J. Y. Li, M. Tian and P. X. Xi, Transition Metal (Fe, Co and Ni)-Carbide-Nitride (M-C-N) Nanocatalysts: Structure and Electrocatalytic Applications, ChemCatChem, 2019, 11, 2780–2792 CrossRef CAS
;
(e) J. Y. Yu, G. X. Li, H. Liu, L. L. Zeng, L. L. Zhao, J. Jia, M. Y. Zhang, W. J. Zhou, H. Liu and Y. Y. Hu, Electrochemical Flocculation Integrated Hydrogen Evolution Reaction of Fe@N-Doped Carbon Nanotubes on Iron Foam for Ultralow Voltage Electrolysis in Neutral Media, Adv. Sci., 2019, 6, 1901458 CrossRef CAS
. -
(a) R. Subbaraman, D. Tripkovic, D. Strmcnik, K. C. Chang, M. Uchimura, A. P. Paulikas, V. Stamenkovic and N. M. Markovic, Enhancing Hydrogen Evolution Activity in Water Splitting by Tailoring Li+-Ni(OH)2-Pt Interfaces, Science, 2011, 334, 1256–1260 CrossRef CAS
;
(b) D. D. Zhang, J. Y. Shi, Y. Qi, X. M. Wang, H. Wang, M. R. Li, S. Z. Liu and C. Li, Quasi-Amorphous Metallic Nickel Nanopowder as an Efficient and Durable Electrocatalyst for Alkaline Hydrogen Evolution, Adv. Sci., 2018, 5, 1801216 CrossRef
;
(c) C. J. Lei, Y. Wang, Y. Hou, P. Liu, J. Yang, T. Zhang, X. D. Zhuang, M. W. Chen, B. Yang, L. C. Lei, C. Yuan, M. Qiu and X. L. Feng, Efficient Alkaline Hydrogen Evolution on Atomically Dispersed Ni–Nx Species Anchored Porous Carbon with Embedded Ni Nanoparticles by Accelerating Water Dissociation Kinetics, Energy Environ. Sci., 2019, 12, 149–156 RSC
;
(d) Z. Fang, L. Peng, Y. Qian, X. Zhang, Y. Xie, J. J. Cha and G. Yu, Dual Tuning of Ni-Co-A (A = P, Se, O) Nanosheets by Anion Substitution and Holey Engineering for Efficient Hydrogen Evolution, J. Am. Chem. Soc., 2018, 140, 5241–5247 CrossRef CAS
;
(e) Y. B. Li, X. Tan, S. Chen, X. Bo, H. J. Ren, S. C. Smith and C. Zhao, Processable Surface Modification of Nickel-Heteroatom (N, S) Bridge Sites for Promoted Alkaline Hydrogen Evolution, Angew. Chem., Int. Ed., 2019, 58, 461–466 CrossRef CAS
. -
(a) R. Paul, L. Zhu, H. Chen, J. Qu and L. M. Dai, Recent Advances in Carbon-Based Metal-Free Electrocatalysts, Adv. Mater., 2019, 31, 1806403 CrossRef
;
(b) B. R. Sathe, X. X. Zou and T. Asefa, Metal-free B-doped graphene with efficient electrocatalytic activity for hydrogen evolution reaction, Catal. Sci. Technol., 2014, 4, 2023–2030 RSC
;
(c) K. G. Qu, Y. Zheng, X. X. Zhang, K. Davey, S. Dai and S. Z. Qiao, Promotion of Electrocatalytic Hydrogen Evolution Reaction on Nitrogen-Doped Carbon Nanosheets with Secondary Heteroatoms, ACS Nano, 2017, 11, 7293–7300 CrossRef CAS
;
(d) L. Z. Zhang, Y. Jia, X. C. Yan and X. D. Yao, Activity Origins in Nanocarbons for the Electrocatalytic Hydrogen Evolution Reaction, Small, 2018, 14, 1800235 CrossRef
;
(e) F. Xiao, Z. M. Chen, H. Wu, Y. Wang, E. P. Cao, X. D. Lu, Y. Q. Wu and Z. Y. Ren, Phytic Acid-Guided Ultra-Thin N,P Co-Doped Carbon Coated Carbon Nanotubes for Efficient All-pH Electrocatalytic Hydrogen Evolution, Nanoscale, 2019, 11, 23027–23034 RSC
. -
(a) H. L. Fei, J. C. Dong, D. L. Chen, T. D. Hu, X. D. Duan, I. Shakir, Y. Huang and X. F. Duan, Single atom electrocatalysts supported on graphene or graphene-like carbons, Chem. Soc. Rev., 2019, 48, 5207–5241 RSC
;
(b) Z. S. Shi, W. Q. Yang, Y. T. Gu, T. Liao and Z. Q. Sun, Metal-Nitrogen-Doped Carbon Materials as Highly Efficient Catalysts: Progress and Rational Design, Adv. Sci., 2020, 7, 2001069 CrossRef CAS
;
(c) X. J. Zhu, J. L. Dai, L. G. Li, D. K. Zhao, Z. X. Wu, Z. H. Tang, L. J. Ma and S. W. Chen, Hierarchical carbon microflowers supported defect-rich Co3S4 nanoparticles: An efficient electrocatalyst for water splitting, Carbon, 2020, 160, 133–144 CrossRef CAS
;
(d) X. P. Gao, Y. A. Zhou, S. Q. Liu, Z. W. Cheng, Y. J. Tan and Z. M. Shen, Single Cobalt Atom Anchored on N-Doped Graphyne for Boosting the Overall Water Splitting, Appl. Surf. Sci., 2020, 502, 144155 CrossRef CAS
;
(e) S. V. Mohite, R. M. Xing, B. Y. Li, S. S. Latthe, Y. Zhao, X. Y. Li, L. Q. Mao and S. H. Liu, Spatial Compartmentalization of Cobalt Phosphide in P-Doped Dual Carbon Shells for Efficient Alkaline Overall Water Splitting, Inorg. Chem., 2020, 59, 1996–2004 CrossRef CAS
;
(f) S. Yu, S. L. Song, R. Li and B. Z. Fang, The lightest solid meets the lightest gas: an overview of carbon aerogels and their composites for hydrogen related applications, Nanoscale, 2020, 12, 19536–19556 RSC
. -
(a) G. F. Liao, Y. Gong, L. Zhang, H. Y. Gao, G. J. Yang and B. Z. Fang, Semiconductor polymeric graphitic carbon nitride photocatalysts: the “holy grail” for the photocatalytic hydrogen evolution reaction under visible light, Energy Environ. Sci., 2019, 12, 2080–2147 RSC
;
(b) G. F. Liao, J. S. Fang, Q. Li, S. H. Li, Z. S. Xu and B. Z. Fang, Ag-Based nanocomposites: synthesis and applications in catalysis, Nanoscale, 2019, 11, 7062–7096 RSC
;
(c) G. Q. Suo, J. Q. Zhang, D. Li, Q. Y. Yu, W. A. Wang, M. He, L. Feng, X. J. Hou, Y. L. Yang, X. H. Ye and L. Zhang, N-doped carbon/ultrathin 2D metallic cobalt selenide core/sheath flexible framework bridged by chemical bonds for high-performance potassium storage, Chem. Eng. J., 2020, 388, 124396 CrossRef CAS
;
(d) D. Li, J. Q. Zhang, S. M. Ahmed, G. Q. Suo, W. A. Wang, L. Feng, X. J. Hou, Y. L. Yang, X. H. Ye and L. Zhang, Amorphous carbon coated SnO2 nanohseets on hard carbon hollow spheres to boost potassium storage with high surface capacitive contributions, J. Colloid Interface Sci., 2020, 574, 174–181 CrossRef CAS
;
(e) G. Q. Suo, J. Q. Zhang, D. Li, Q. Y. Yu, M. He, L. Feng, X. J. Hou, Y. L. Yang, X. H. Ye, L. Zhang and W. A. Wang, J. Colloid Interface Sci., 2020, 566, 427–433 CrossRef CAS
;
(f) X. F. Wang, H. X. Liu, Q. Wang, J. H. Huo, W. Y. Ge, X. B. Duan and S. Q. Guo, Appl. Surf. Sci., 2021, 540, 148351 CrossRef CAS
. -
(a) X. M. Guo, W. Zhang, D. Zhang, S. L. Qian, X. Z. Tong, D. C. Zhou, J. H. Zhang and A. H. Yuan, Co9S8/CoS/C submicron-spheres derived from bacteria for electrocatalytic oxygen reduction reaction, ChemElectroChem, 2019, 6, 4571–4575 CrossRef CAS
;
(b) X. F. Liu, C. C. Hao, L. H. He, C. Yang, Y. B. Chen, C. B. Jiang and R. H. Yu, Yolk-shell structured Co-C/Void/Co9S8 composites witha tunable cavity for ultrabroad band and efficient low-frequency microwave absorption, Nano Res., 2018, 11, 4169–4182 CrossRef CAS
;
(c) P. Y. Zeng, J. W. Li, M. Ye, K. F. Zhuo and Z. Fang, In Situ Formation of Co9S8/N-C Hollow Nanospheres by Pyrolysis and Sulfurization of ZIF-67 for High-Performance Lithium-Ion Batteries, Chem.–Eur. J., 2017, 23, 9517–9524 CrossRef CAS
;
(d) Y. Y. Zhao, Q. Fu, D. S. Wang, Q. Pang, Y. Gao, A. Missiul, R. Nemausat, A. Sarapulova, H. Ehrenberg, Y. J. Wei and G. Chen, Co9S8@carbon yolk-shell nanocages as a high performance direct conversion anode material for sodium ion batteries, Energy Storage Materials, 2019, 18, 51–58 CrossRef
;
(e) H. X. Zhong, K. Li, Q. Zhang, J. Wang, F. L. Meng, Z. J. Wu, J. M. Yan and X. B. Zhang, In situ anchoring of Co9S8 nanoparticles on N and S co-doped porous carbon tube as bifunctional oxygen electrocatalysts, NPG Asia Mater., 2016, 8, e308 CrossRef CAS
. -
(a) J. Du, R. Wang, Y. R. Lv, Y. L. Wei and S. Q. Zang, One-step MOF-derived Co/Co9S8 nanoparticles embedded in nitrogen, sulfur and oxygen ternary-doped porous carbon: an efficient electrocatalyst for overall water splitting, Chem. Commun., 2019, 55, 3203–3206 RSC
;
(b) N. Huang, S. F. Yan, M. Y. Zhang, Y. Y. Ding, L. Yang, P. P. Sun and X. H. Sun, A MoS2-Co9S8-NC heterostructure as an efficient bifunctional electrocatalyst towards hydrogen and oxygen evolution reaction, Electrochim. Acta, 2019, 327, 134942 CrossRef CAS
;
(c) H. Yu, X. Y. Sun, D. H. Tang, Y. Huang, W. T. Zhang, S. J. Miao, Z. A. Qiao, J. J. Wang and Z. Zhao, Molten salt strategy to synthesize alkali metal doped Co9S8 nanoparticles embedded, N, S co-doped mesoporous carbon as hydrogen evolution electrocatalyst, Int. J. Hydrogen Energy, 2020, 45, 6006–6014 CrossRef CAS
;
(d) L. G. Wang, X. X. Duan, X. J. Liu, J. Gu, R. Si, Y. Qiu, Y. M. Qiu, D. E. Shi, F. H. Chen, X. M. Sun, J. H. Lin and J. L. Sun, Atomically Dispersed Mo Supported on Metallic Co9S8 Nanoflakes as an Advanced Noble-Metal-Free Bifunctional Water Splitting Catalyst Working in Universal pH Conditions, Adv. Energy Mater., 2019, 9, 1903137 Search PubMed
;
(e) J. G. Yan, L. G. Chen and X. Liang, Co9S8 Nanowires@NiCo LDH Nanosheets Arrays on Nickel Foams towards Efficient Overall Water Splitting, Sci. Bull., 2019, 64, 158–165 CrossRef CAS
. -
(a) B. J. Zhu, R. Q. Zou and Q. Xu, Metal−Organic Framework Based Catalysts for Hydrogen Evolution, Adv. Energy Mater., 2018, 8, 1801193 CrossRef
;
(b) P. Q. Liao, J. Q. Shen and J. P. Zhang, Metal−organic frameworks for electrocatalysis, Coord. Chem. Rev., 2018, 373, 22–48 CrossRef CAS
;
(c) L. J. Kong, M. Zhong, W. Shuang, Y. H. Xu and X. H. Bu, Electrochemically active sites inside crystalline porous materials for energy storage and conversion, Chem. Soc. Rev., 2020, 49, 2378–2407 RSC
;
(d) X. D. Wen and J. Q. Guan, Recent progress on MOF-derived electrocatalystsfor hydrogen evolution reaction, Applied Materials Today, 2019, 16, 146–168 CrossRef
;
(e) H. Kobayashi, Y. Mitsuka and H. Kitagawa, Metal Nanoparticles Covered with a Metal−Organic Framework: From One-Pot Synthetic Methods to Synergistic Energy Storage and Conversion Functions, Inorg. Chem., 2016, 55, 7301–7310 CrossRef CAS
;
(f) D. Liu, X. M. Zhang, Y. J. Wang, S. Y. Song, L. F. Cui, H. B. Fan, X. C. Qiao and B. Z. Fang, A new perspective of lanthanide metal−organic frameworks: tailoring Dy-BTC nanospheres for rechargeable Li−O2 batteries, Nanoscale, 2020, 12, 9524–9532 RSC
;
(g) Y. W. Li, W. J. Zhang, J. Li, H. Y. Ma, H. M. Du, D. C. Li, S. N. Wang, J. S. Zhao, J. M. Dou and L. Q. Xu, Fe-MOF-Derived Efficient ORR/OER Bifunctional Electrocatalyst for Rechargeable Zinc−Air Batteries, ACS Appl. Mater. Interfaces, 2020, 12, 44710–44719 CrossRef CAS
. -
(a) L. T. Yan, L. Cao, P. C. Dai, X. Gu, D. D. Liu, L. J. Li, Y. Wang and X. B. Zhao, Metal−Organic Frameworks Derived Nanotube of Nickel–Cobalt Bimetal Phosphides as Highly Efficient Electrocatalysts for Overall Water Splitting, Adv. Funct. Mater., 2017, 27, 1703455 CrossRef
;
(b) B. C. Weng, C. R. Grice, W. W. Meng, L. Guan, F. H. Xu, Y. Yu, C. L. Wang, D. W. Zhao and Y. F. Yan, Metal−Organic Framework-Derived CoWP@C Composite Nanowire Electrocatalyst for Efficient Water Splitting, ACS Energy Lett., 2018, 3, 1434–1442 CrossRef CAS
;
(c) W. Q. Liu, Y. M. Zhou, J. H. Bao, J. Q. Wang, Y. W. Zhang, X. L. Sheng, Y. Xue, C. Guo and X. X. Chen, Co-CoO-ZnFe2O4 encapsulated in carbon nanowires derived from MOFs as electrocatalysts for hydrogen evolution, J. Colloid Interface Sci., 2020, 561, 620–628 CrossRef CAS
;
(d) Y. J. Lei, L. Wei, S. L. Zhai, Y. Q. Wang, H. E. Karahan, X. C. Chen, Z. Zhou, C. J. Wang, X. Sui and Y. Chen, Metal-free bifunctional carbon electrocatalysts derived from zeolitic imidazolate frameworks for efficient water splitting, Mater. Chem. Front., 2018, 2, 102–111 RSC
;
(e) Y. L. Li, B. M. Jia, B. Y. Chen, Q. L. Liu, M. K. Cai, Z. Q. Xue, Y. A. Fan, H. P. Wang, C. Y. Su and G. Q. Li, MOF-derived Mn doped porous CoP nanosheets as efficient and stable bifunctional electrocatalysts for water splitting, Dalton Trans., 2018, 47, 14679–14685 RSC
. - H. Y. Ma, Y. Z. Zhang, H. Yan, W. J. Zhang, Y. W. Li, S. N. Wang, D. C. Li, J. M. Dou and J. R. Li, Two Microporous CoII-MOFs with Dual Active Sites for Highly Selective Adsorption of CO2/CH4 and CO2/N2, Dalton Trans., 2019, 48, 13541–13545 RSC
. -
(a) A. C. Ferrari and D. M. Basko, Raman spectroscopy as a versatile tool for studying the properties of grapheme, Nat. Nanotechnol., 2013, 8, 235–246 CrossRef CAS
;
(b) N. Sikdar, B. Konkena, J. Masa, W. Schuhmann and T. K. Maji, Co3O4@Co/NCNT Nanostructure Derived from a Dicyanamide-Based Metal–Organic Framework as an Efficient Bi-functional Electrocatalyst for Oxygen Reduction and Evolution Reactions, Chem.–Eur. J., 2017, 23, 18049–18056 CrossRef CAS
;
(c) D. N. Ding, K. Shen, X. D. Chen, H. R. Chen, J. Y. Chen, T. Fan, R. F. Wu and Y. W. Li, Multi-Level Architecture Optimization of MOF-Templated Co-Based Nanoparticles Embedded in Hollow N-Doped Carbon Polyhedra for Efficient OER and ORR, ACS Catal., 2018, 8, 7879–7888 CrossRef CAS
;
(d) S. M Alshehri, J. Ahmed, A. Khan, M. Naushad and T. Ahamad, Bifunctional Electrocatalysts (Co9S8@NSC) Derived from a Polymer-metal Complex for the Oxygen Reduction and Oxygen Evolution Reactions, ChemElectroChem, 2018, 5, 355–361 CrossRef
. -
(a) Y. W. Li, J. Xu, D. C. Li, J. M. Dou, H. Yan, T. L. Hu and X. H. Bu, Two microporous MOFs constructed from different metal cluster SBUs for selective gas adsorption, Chem. Commun., 2015, 51, 14211–14214 RSC
;
(b) Y. W. Li, H. Yan, T. L. Hu, H. Y. Ma, D. C. Li, S. N. Wang, Q. X. Yao, J. M. Dou, J. Xu and X. H. Bu, Two microporous Fe-based MOFs with multiple active sites for selective gas adsorption, Chem. Commun., 2017, 53, 2394–2397 RSC
;
(c) D. S. Zhang, Y. Z. Zhang, X. L. Zhang, F. Wang, J. Zhang, H. Hu, J. Gao, H. Yan, H. L. Liu, H. Y. Ma, L. L. Geng and Y. W. Li, Nanocage-Based Porous Metal–Organic Frameworks Constructed from Icosahedrons and Tetrahedrons for Selective Gas Adsorption, ACS Appl. Mater. Interfaces, 2019, 11, 20104–20109 CrossRef CAS
. -
(a) N. Yao, P. Li, Z. R. Zhou, Y. M. Zhao, G. Z. Cheng, S. L. Chen and W. Luo, Synergistically Tuning Water and Hydrogen Binding Abilities Over Co4N by Cr Doping for Exceptional Alkaline Hydrogen Evolution Electrocatalysis, Adv. Energy Mater., 2019, 9, 1902449 CrossRef CAS
;
(b) Z. Y. Chen, Y. Song, J. Y. Cai, X. S. Zheng, D. D. Han, Y. S. Wu, Y. P. Zang, S. W. Niu, Y. Liu, J. F. Zhu, X. J. Liu and G. M. Wang, Tailoring the d-Band Centers Enables Co4N Nanosheets To Be Highly Active for Hydrogen Evolution Catalysis, Angew. Chem., Int. Ed., 2018, 57, 5076–5080 CrossRef CAS
;
(c) Y. P. Wu, W. Zhou, J. Zhao, W. W. Dong, Y. Q. Lan, D. S. Li, C. H. Sun and X. H. Bu, Surfactant-Assisted Phase-Selective Synthesis of New Cobalt MOFs and Their Efficient Electrocatalytic Hydrogen Evolution Reaction, Angew. Chem., Int. Ed., 2017, 56, 13001–13005 CrossRef CAS
;
(d) H. Li, P. Wen, D. S. Itanze, M. W. Kim, S. Adhikari, C. Lu, L. Jiang, Y. J. Qiu and S. M. Geyer, Phosphorus-Rich Colloidal Cobalt Diphosphide (CoP2) Nanocrystals for Electrochemical and Photoelectrochemical Hydrogen Evolution, Adv. Mater., 2019, 31, 1900813 CrossRef
;
(e) H. P. Feng, L. Tang, G. M. Zeng, J. F. Yu, Y. C. Deng, Y. Y. Zhou, J. J. Wang, C. Y. Feng, T. Luo and B. B. Shao, Electron density modulation of Fe1-xCoxP nanosheet arrays by iron incorporation for highly efficient water splitting, Nano Energy, 2020, 67, 104174 CrossRef CAS
. -
(a) E. P. Cao, Z. M. Chen, H. Wu, P. Yu, Y. Wang, F. Xiao, S. Chen, S. C. Du, Y. Xie, Y. Q. Wu and Z. Y. Ren, Boron-Induced Electronic-Structure Reformation of CoP Nanoparticles Drives Enhanced pH-Universal Hydrogen Evolution, Angew. Chem., Int. Ed., 2020, 59, 4154–4160 CrossRef CAS
;
(b) Y. Tong, X. W. Yu, H. Y. Wang, B. W. Yao, C. Li and G. Q. Shi, Trace Level Co-N Doped Graphite Foams as High-Performance Self-Standing Electrocatalytic Electrodes for Hydrogen and Oxygen Evolution, ACS Catal., 2018, 8, 4637–4644 CrossRef CAS
;
(c) X. Q. Wang, J. R. He, B. Yu, B. C. Sun, D. X. Yang, X. J. Zhang, Q. H. Zhang, W. L. Zhang, L. Gu and Y. F. Chen, CoSe2 nanoparticles embedded MOF-derived Co-N-C nanoflake arrays as efficient and stable electrocatalyst for hydrogen evolution reaction, Appl. Catal., B, 2019, 258, 117996 CrossRef CAS
;
(d) Y. J. Zhang, W. F. Li, L. H. Lu, W. G. Song, C. R. Wang, L. S. Zhou, J. H. Liu, Y. Chen, H. Y. Jin and Y. G. Zhang, Tuning active sites on cobalt/nitrogen doped graphene for electrocatalytic hydrogen and oxygen evolution, Electrochim. Acta, 2018, 265, 497–506 CrossRef CAS
;
(e) S. M. Wang, L. Zhang, Y. Qin, D. Ding, Y. F. Bu, F. Q. Chu, Y. Kong and M. L. Liu, Co,N-codoped graphene as efficient electrocatalyst for hydrogen evolution reaction: Insight into the active centre, J. Power Sources, 2017, 363, 260–268 CrossRef CAS
;
(f) X. Z. Ma, K. Y. Li, X. Zhang, B. Wei, H. Yang, L. N. Liu, M. Y. Zhang, X. T. Zhang and Y. J. Chen, The surface engineering of cobalt carbide spheres through N, B co-doping achieved by room-temperature in situ anchoring effects for active and durable multifunctional electrocatalysts, J. Mater. Chem. A, 2019, 7, 14904–14915 RSC
. -
(a) J. R. He, Y. F. Chen and A. Manthiram, Vertical Co9S8 hollow nanowall arrays grown on Celgard separator as a multifunctional polysulfide barrier for high-performance Li-S batteries, Energy Environ. Sci., 2018, 11, 2560–2568 RSC
;
(b) W. Xiong, K. Hu, Z. Li, Y. X. Jiang, Z. G. Li, Z. Li and X. W. Wang, A wearable system based on core-shell structured peptide-Co9S8 supercapacitor and triboelectric nanogenerator, Nano Energy, 2019, 66, 104149 CrossRef CAS
.
Footnote |
† Electronic supplementary information (ESI) available: Materials and characterization, experimental details, structure and structure parameters of Co-MOF (LCU-105), and IR spectra of Co-MOF-900. See DOI: 10.1039/d0ra10864b |
|
This journal is © The Royal Society of Chemistry 2021 |