DOI:
10.1039/D0RA09553B
(Paper)
RSC Adv., 2021,
11, 5399-5410
Dissipation and sorption–desorption of benzisothiazolinone in agricultural soils and identification of its metabolites†
Received
10th November 2020
, Accepted 22nd January 2021
First published on 1st February 2021
Abstract
Benzisothiazolinone has been widely used to control bacterial and fungal diseases in various agricultural crops by destroying the nuclear structure and interfering with the metabolism of microbial cells. In this study, the dissipation, transformation and sorption–desorption of benzisothiazolinone (BIT) in five soils were investigated to evaluate its environmental fate. Results showed that the degradation of BIT in all the tested soils fitted the first order kinetics and increased with soil organic matter (OM) content. Degradation differences between unsterilized natural and sterilized soils (t1/2 = 0.09–26.66 and 6.80–86.64 d) suggested that BIT degradation is primarily driven by biological processes and assisted by abiotic degradation. Additionally, BIT dissipated fastest in flooded soils (t1/2 = 0.20–4.53 d), indicating that anaerobic microorganisms are more likely to degrade BIT compared to aerobic microbes. Also, during the soil degradation process, two metabolites were monitored and identified for the first time. BIT sorption was a spontaneous physical process with no desorption hysteresis effect, which fit the Freundlich model. BIT causes relatively strong sorption (log
KOC = 3.76–4.19) and low persistence in soils, thus exhibiting a low potential risk for groundwater contamination.
1. Introduction
The extensive utilization of pesticides has made great contributions to the development of agriculture for the growing world population.1 However, the excessive usage of pesticides has incited great concern over the environmental issues and environmental fate of pesticides. Soil leaching and runoff following application of pesticides to crops are the primary routes of water resource contamination by pesticides.2 Sorption affects leaching and runoff, and it also governs pesticide bioavailability, because it controls the amount of pesticide that is available for leaching, runoff, plant uptake, and microbial degradation.3,4 Increased pesticide sorption often reduces the degree of degradation, thereby decreasing pesticide bioavailability.3 In addition, compounds with poor sorption capacity have a high risk of leaching into waterbodies.5 For example, oxyfluorfen has a low and acceptable leaching risk, due to its high sorption on all three Hawaiian soils.6 Thus, the mobility and persistence of pesticides in soil may affect its pollution potential to water, and consequently influence human health through the food chain. To protect the environment from contamination, knowledge of the environmental behavior and fate of pesticides is required.
To a large extent, the partitioning and transformation of pesticides in soil are affected by soil properties, pesticide characteristics, and environmental conditions.7 Soils with higher contents of organic matter (OM) cause larger sorption and faster degradation.8–11 Pesticide degradation in the soil involves chemical degradation and biological degradation. Biodegradation performed by the microbial community present in the soil plays an essential role in dissipating pesticides in the soil.12,13 Chemical redox technology, photocatalytic degradation and microbial remediation have been used to degrade pollutants in soils. However, microbial degradation is considered a major technique and the main pathway for eliminating almost all kinds of environmental pollutants.14
Benzisothiazolinone (1,2-benzisothiazolin-3-one; BIT), an organic heterocyclic fungicide, has been widely used to control bacterial and fungal diseases with high efficiency and broad bactericidal spectrum.15,16 BIT (C7H5NOS) is white to off-white fine, crystalline powder, molecular weight: 151.19 g mol−1, boiling point: 327.6 °C, melting point: 156.6 °C, solubility in water: 1.1 g L−1 (20 °C), pKa: 7.3 (25 °C), vapor pressure: 2.78 × 10−6 mm Hg (25 °C), log
Kow: 0.76 (30 °C, pH 7). It has been registered in China for use in various agricultural crops, such as wheat, rice, cucumber, tobacco, citrus, and apple. Additionally, BIT is also used as an important industrial bactericidal and mildew-proof preservative, which has remarkable effect of inhibiting the growth of microorganisms (fungus, bacteria, and algae).16 It can destroy the nuclear structure and interfere with the metabolism of microbial cells, leading to physiological disorders, collapse, and eventually death.17 However, BIT could cause certain risks for human and animals. Human dermal exposure to BIT at sufficient dose and duration can produce skin sensitization and allergic contact dermatitis.18 Decreased body weight, vomiting, damage to the lining of the stomach, increased absolute liver weight, and changes in blood chemistry were observed in laboratory animals (rats and dogs) exposed to a moderate oral doses of BIT over a long period of time.19 Additionally, it is very toxic to aquatic organisms (Oncorhynchus mykiss, 96 h LC50 = 0.75 mg L; Lepomis macrochirus, 96 h LC50 = 0.54 mg L; Daphnia magna, 48 h EC50 = 0.097 mg L−1).20 Studies related to BIT mainly focused on synthetic method, control efficiency on target organisms, and instrumental analysis method of residue detection in crops (cucumber, wheat, and tobacco) and soil.15–17,21,22 Previous investigation of our group found BIT residues in citrus, and the results showed BIT poses certain dietary exposure risk to human.23 United States Environmental Protection Agency (EPA) have reported the application amount of BIT to crops is 0.02 lbs per acre.19 BIT may transfer to soil environment after its large application to crops, and cause adverse environmental effects. To our knowledge, little published information regarding the environmental behavior and fate of BIT in the soil is available. Based on its extensive use, high solubility in water, and potential risks to aquatic life and human health, the dissipation and partitioning of BIT should be investigated to ensure adequate degradation and low mobility prior to its release into the waterbodies. Present study investigated the dissipation, sorption–desorption of BIT in five agricultural soils, and identified the transformation products of BIT in soil for the first time.
This study aims to (1) investigate the dissipation of BIT in five soils as well as determine the influence of soil properties, flooding, and autoclaving on degradation rates, (2) identify the transformation products of BIT in soils and (3) study sorption kinetics and thermodynamics, and sorption–desorption isotherms of BIT in soils. Present study will provide a scientific basis for assessing the mobility and persistence of BIT in the soil, as well as evaluating its potential environmental risks.
2. Materials and methods
2.1. Chemicals
BIT standards with purity of 99.37% were purchased from Xi'an Hytech Agrochemicals Co., Ltd. (Xian, China). LC-grade methanol and formic acid were obtained from Thermo Fisher Scientific (Waltham, MA, USA). Distilled water was supplied by the Watson Group (Hong Kong, China). Other solvents used (sodium chloride (NaCl), acetonitrile, calcium chloride (CaCl2)) were of analytical grade. A stock standard solution of BIT at a concentration of 200 mg L−1 was prepared by dissolving BIT in methanol.
2.2. Soil samples collection
Five typical soil samples with no history of BIT application were collected from Jilin (soil #1), Anhui (soil #3), Guizhou (soil #4), and Shandong (soil #5) provinces, as well as Beijing city (soil #2), representing different regions with BIT usage in China. At each location, surface soil (0–20 cm) was randomly sampled, air dried, sieved through a 2 mm sieve, and then stored at 4 °C in the dark until use. Physicochemical properties of these soils, including soil organic matter (OM) content, pH, cation-exchange capacity (CEC) and texture, were determined according to a series of standard methods,24–27 and presented in Table 1. Soil #1 is sandy clay soil, pH 5.82, OM (%) 7.50, CEC (%) 24.3; soil #2 is sandy soil, pH 7.91, OM (%) 6.10, CEC (%) 17.5; soil #3 is loamy sand soil, pH 8.71, OM (%) 6.57, CEC (%) 18.2; soil #4 is clay soil, pH 8.15, OM (%) 3.10, CEC (%) 18.6; soil #5 is sandy loam soil, pH 8.96, OM (%) 2.35, CEC (%) 16.6.
Table 1 Physicochemical properties of five experimental soils
No. |
Texture (%) |
Textural class |
pH |
CEC (%) |
OC (%) |
OM (%) |
Soil sites |
Clay |
Silt |
Sand |
#1 |
25.3 |
22.4 |
52.3 |
Sandy clay |
5.82 |
24.3 |
4.35 |
7.50 |
Baishan, Jilin (41° 56′ N, 126° 26′ E) |
#2 |
9.2 |
31.2 |
59.6 |
Sandy |
7.91 |
17.5 |
3.54 |
6.10 |
Beijing (39° 92′ N, 116° 46′ E) |
#3 |
8.2 |
15.1 |
76.7 |
Loamy sand |
8.71 |
18.2 |
3.81 |
6.57 |
Hefei, Anhui (31° 86′ N, 117° 27′ E) |
#4 |
45 |
28.3 |
26.7 |
Clay |
8.15 |
18.6 |
1.80 |
3.10 |
Guiyang, Guizhou (26° 57′ N, 106° 71′ E) |
#5 |
7.8 |
30 |
62.2 |
Sandy loam |
8.96 |
16.6 |
1.36 |
2.35 |
Qingdao, Shandong (36° 07′ N, 120° 33′ E) |
2.3. Instrumental analysis conditions
2.3.1. Determination of the BIT by LC-MS/MS. BIT analysis was conducted on a Shimadzu 20AD-XR LC system (SHIMADZU Corporation, Tokyo, Japan) coupled with an AB Sciex 4000Q TRAP mass spectrometer (Applied BioSystems, Foster City, CA, USA). Chromatographic separation was conducted on an Eclipse XDB-C18 column (4.6 mm × 150 mm, 5 μm particle size, Agilent, Santa Clara, CA, USA) with column temperature of 40 °C. The mobile phase comprised 0.1% (v/v) formic acid in ultrapure water as eluent A and LC-grade methanol as eluent B with a flow rate of 1 mL min−1. The gradient elution mode was set as: 0–0.5 min, 10% B; 3–4 min, 90% B; 4.1–6 min, 10% B. Throughout the analysis, the injection volume was 5 μL. Analyses were performed by positive electrospray ionization (ESI+) with the multiple reaction monitoring mode (MRM). Representative chromatograms of BIT are available in Fig. S1.†Limits of detection (LOD) and quantitation (LOQ) for the developed method were determined using matrix-matched standard solution. The LOD was calculated as the concentration resulting in a signal-to-noise ratio (S/N) of 3, whereas the LOQ was determined as the concentration resulting in a signal-to-noise ratio (S/N) of 10. The LODs and LOQs for BIT in five blank soil samples were detected to be 0.06 mg kg−1 and 0.2 mg kg−1 respectively (Table S1†). Mean recoveries of BIT in soils were satisfactory with a recovery of 74.40–103.67% and RSD of 0.92–8.28% (Table S2†).
2.3.2. Identification of metabolites by UHPLC-HRMS. The identification of metabolite was conducted on a Dionex UltiMate 3000 RSLC UHPLC system (Dionex, Sunnyvale, CA, USA) connected to a Q-Exactive Orbitrap mass spectrometer (Thermo Fischer Scientific, MA, USA). A Kinetex Polar C18 column (2.1 mm × 100 mm, 2.6 μm, 100 Å, Phenomenex) was used at 30 °C with 5 μL of the injection volume. Eluent A was ultrapure water acidified with 0.1% formic acid, and eluent B was methanol containing 0.1% formic acid. The gradient elution mode with a flow rate of 0.25 mL min−1 was set as: 0–1 min, 100% A; 11.5–12.5 min, 100% B; 12.6–15 min, 100% A. The mass spectrometer was operated with an electrospray ionization source (ESI) in positive full-scan mode at a resolution of 70
000 (at 50–300 m/z). Spectra data were analyzed using Xcalibur 4.0 (Thermo Fisher Scientific, Carlsbad, CA) and Compound Discoverer 2.0 (Thermo Fisher Scientific, Carlsbad, CA) software.
2.4. Degradation experiments
The degradation of the BIT in five soils were studied under sterilized, unsterilized, and flooded conditions. To investigate the role of soil microorganism in the degradation of BIT, a subsample of each soil was sterilized at 120 °C for 30 min in triplicate before incubation. For all treatments, a portion of 5.0 g soil was weighed into 50 mL polypropylene centrifuge tube and maintained at 60% water holding capacity (WHC) adjusted using distilled water. Then, soil samples were pre-incubated for 5 d in an incubator at 25 °C before spiking with BIT to achieve an initial nominal concentration of 10 mg kg−1. For flooded treatments, additional 5 mL of distilled water was added to raise the level of water to approximately 1 cm above the soil surface. Soil samples were incubated in the dark in an incubator at 25 °C for 0, 0.5, 1, 2, 5, 10, 20, 60, and 120 d under sterilized and unsterilized conditions, and for 0, 0.5, 1, 2, 5, 10, 20, and 60 d under flooded condition (0, 2, 4, 6, 8, 10, 12, and 24 h for soil #1 under unsterilized and flooded conditions).
At each period, triplicate soil samples from each treatment were collected and extracted using 5 mL of distilled water and 20 mL acetonitrile, and vigorously vortexed for 5 min at 2500 rpm. After the addition of 5 g NaCl, sample tubes were immediately vortexed for 2 min, and then centrifuged at 6000 rpm for 5 min. Then, 1 mL of the supernatant solution was transferred into a 2 mL plastic centrifuge tube containing 100 mg C18, and vortexed for 1 min. Finally, the supernatant was filtered through a 0.22 μm filter and analyzed by liquid chromatography-tandem mass spectrometry (LC-MS/MS).
2.5. Sorption–desorption experiments
Sorption–desorption studies of BIT were conducted in five sterilized soils by a batch equilibrium method using an Erlenmeyer flask (250 mL) in a thermostatic water bath shaker (25 ± 2 °C, darkness). Solutions used in this experiment were prepared by diluting BIT stock solution with 0.01 mol L−1 CaCl2 aqueous solution to the required concentration. For sorption kinetics experiments, 50 mL of 5 mg L−1 BIT was added to the 250 mL Erlenmeyer flask containing 5.0 g air dried soil, resulting in a soil/solution ratio of 1
:
10 (w/v). Then, the sample flasks were closed with caps, shaken manually for 1 min, and then agitated on a shaker for specific periods (0.5, 1, 2, 4, 8, 12, and 24 h). Sorption isotherm experiments in soils were evaluated at five different concentrations of BIT (0.1, 0.5, 1, 5, and 10 mg L−1) and shaken for 24 h. Blanks were prepared by adding 50.0 mL of 0.01 mol L−1 CaCl2 solution without BIT to 5.0 g soil and treated as described above. Simultaneously, 50 mL of 0.01 mol L−1 CaCl2 solutions spiked with BIT were included for each treatment as a control. After specific shaking periods, soil suspensions were transferred and centrifuged at 6000 rpm for 5 min. Then, an aliquot of 1.0 mL supernatant from each sample was removed and filtered through a 0.22 μm filter. Subsequently, the filtrate was directly analyzed by LC-MS/MS. The determination of BIT in soils was the same as that for the degradation experiments.
Desorption isotherm was determined immediately after sorption by adding 50 mL of 0.01 mol L−1 CaCl2 without BIT to soils used for sorption isotherms. After 24 h of agitation at 25 ± 2 °C, the solution was transferred, centrifuged, filtered, and analyzed as described for sorption determination. All experiments were performed in three replications.
2.6. Calculation and statistical analyses
The degradation rate constants k of BIT in soils were determined from eqn (1), and the half life (t1/2, d) was calculated from eqn (2). |
t1/2 = ln 2/k = 0.693/k
| (2) |
where C0 (mg kg−1) is the initial concentration of BIT in soils, C (mg kg−1) is the concentration of BIT at time t (d), k is the degradation rate constants of BIT.
Sorption kinetic data were analyzed using pseudo-first-order, pseudo-second-order and intraparticle diffusion models. They are expressed as eqn (3)–(5), respectively.
|
log(Cs − Ct) = log Cs − k1t/2.303
| (3) |
|
t/Ct = 1/(k2Cs2) + t/Cs
| (4) |
where
Cs and
Ct are the concentration (mg kg
−1) of
BIT absorbed to the soils at the equilibrium state and at time
t (min), respectively; while
k1 and
k2 are the pseudo-first-order rate constant (min
−1) and the pseudo-second-order rate constant (kg (mg min)
−1), respectively;
kpi is the intraparticle diffusion rate constant (mg (kg min
0.5)
−1),
Ci is the intercept which is proportional to the boundary layer thickness.
Data obtained from sorption isotherm experiments were fitted to the linear isotherm, the Freundlich sorption isotherm, and the Langmuir isotherm. They are shown in eqn (6)–(8), respectively.
|
Log Cs = lg Kf + (1/n)log Ce
| (7) |
|
Ce/Cs = 1/(KLQmax) + Ce/Qmax
| (8) |
where
Cs (mg kg
−1) is defined in
eqn (3),
Ce (mg L
−1) is the equilibrium concentration of
BIT in the aqueous phase. The sorption coefficient,
Kd (L kg
−1), is the slope of the linear isotherm model.
Kf is the Freundlich sorption coefficient (L kg
−1), 1/
n is the Freundlich exponent.
KL is the Langmuir constant (L mg
−1), and
Qmax is the maximum sorption capacity (mg kg
−1).
Kd values were normalized to soil organic carbon content (OC%) to obtain the soil organic carbon–water partitioning coefficient (KOC) using eqn (9). The OC was calculated from the organic matter (OM) content obtained for each soil, and 1.724 is the ratio of OM to OC content.28
Data obtained from desorption isotherm experiments were fitted to the Freundlich desorption isotherm model (eqn (10)).
|
log Cs = lg Kfd + (1/nd)log Ce
| (10) |
where
Cs is the concentration (mg kg
−1) of
BIT absorbed to the soils at the equilibrium state,
Ce (mg L
−1) is the equilibrium concentration of
BIT in the aqueous phase.
Kfd is the Freundlich desorption coefficient (L kg
−1), 1/
nd is the Freundlich exponent from desorption isotherms.
The hysteresis coefficient (H) was determined for the sorption–desorption isotherms according to eqn (11).
where 1/
n and 1/
nd are the Freundlich constants obtained for the sorption and desorption isotherms, respectively.
The thermodynamic parameter, standard free energy change (ΔG°, kJ mol−1), of BIT sorption process at 25 °C was calculated according to the following eqn (12) and (13).
|
ΔG° =−RT ln KOM
| (12) |
where
R is the molar gas constant (8.314 × 10
−3 kJ (K mol)
−1) and
T is the sorption temperature (K). In this study,
T is considered as 298.15 K, while
KOM is the soil organic matter–water partition coefficient.
In addition, data processing such as determining the average value, standard deviation (SD), and relative standard deviation (RSD) were performed using Excel 2013 software (Microsoft Corp., Redmond, WA, USA). Regression analysis was conducted using SPSS 16.0 (SPSS Inc., Armonk, NY, USA). Graphs were plotted by OriginPro 2017 (OriginLab Corp., Northampton, MA, USA).
3. Results and discussion
3.1. Degradation of BIT in soils
BIT degradation in five soils under unsterilized, sterilized, and flooded conditions well followed the first-order kinetics model with R2 between 0.8743–0.9991 (Fig. 1). Degradation rate constants and half-lives for BIT are presented in Table 2. BIT loss in hydrolysis controls was limited to 10% after 120 d, indicating that abiotic hydrolysis was slow. The degradation of BIT was influenced by OM content, soil microorganisms and flooding condition.
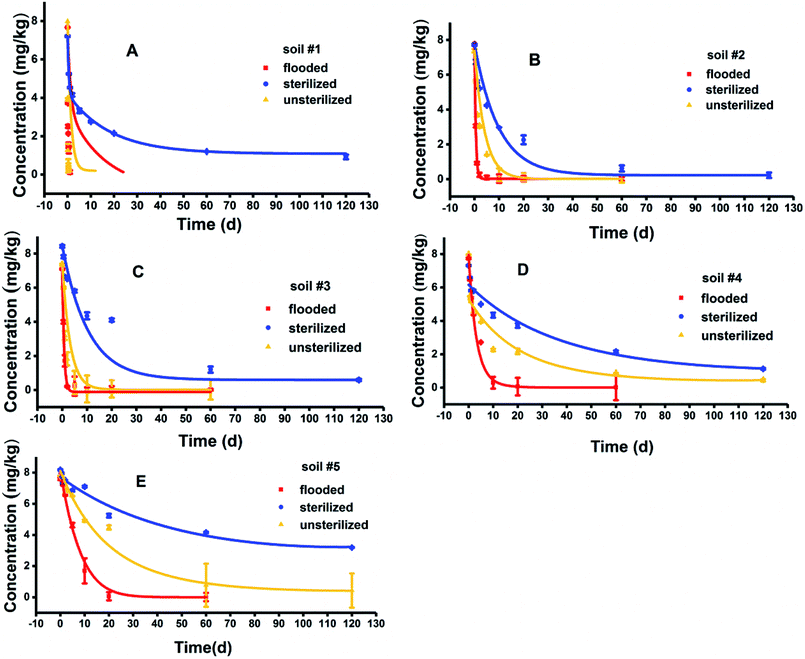 |
| Fig. 1 The dissipation curves of BIT in five soils under three different conditions: (A) in soil #1, (B) in soil #2, (C) in soil #3, (D) in soil #4, (E) in soil #5. The error bars represent the standard deviation. | |
Table 2 BIT dissipation curves and the half-lives in five different soils under three incubation conditions
Treatments |
Soils |
Dissipation curve |
k (d−1) |
R2 |
t1/2 (d) |
Unsterilized |
#1 |
C = 5.9226e−7.443t |
7.4430 |
0.9322 |
0.09 |
#2 |
C = 7.2480e−0.3667t |
0.3667 |
0.9012 |
1.89 |
#3 |
C = 6.7144e−0.564t |
0.5640 |
0.9797 |
1.23 |
#4 |
C = 7.9579e−0.2014t |
0.2014 |
0.8743 |
3.44 |
#5 |
C = 7.0526e−0.026t |
0.0260 |
0.9387 |
26.66 |
Sterilized |
#1 |
C = 7.2116e−0.0862t |
0.0862 |
0.9375 |
8.04 |
#2 |
C = 7.5047e−0.102t |
0.1020 |
0.9446 |
6.80 |
#3 |
C = 7.8421e−0.022t |
0.0220 |
0.9501 |
31.51 |
#4 |
C = 7.3168e−0.015t |
0.0150 |
0.9505 |
46.21 |
#5 |
C = 7.3857e−0.008t |
0.0080 |
0.9128 |
86.64 |
Flooded |
#1 |
C = 5.0795e−3.4460t |
3.4460 |
0.9192 |
0.20 |
#2 |
C = 7.7783e−1.9686t |
1.9686 |
0.9991 |
0.35 |
#3 |
C = 7.1178e−1.5676t |
1.5676 |
0.9773 |
0.44 |
#4 |
C = 8.1966e−0.313t |
0.3130 |
0.9622 |
2.21 |
#5 |
C = 8.4968e−0.153t |
0.1530 |
0.9732 |
4.53 |
3.1.1. Influence of soil organic matter content on the degradation of BIT. The degradation of BIT in soils was rapid under unsterilized conditions (Fig. 1), approximately 100% of the applied amount were degraded in 24 h for soil #1; however, approximately 96.62%, 99.16%, 71.77%, and 37.19% of the applied amount were degraded at 10 d for soils #2, #3, #4, and #5, respectively. At the end of incubation (120 d), the dissipation rate was >94.50% for soils #2, #3, #4, and #5. Some amide-containing heterocyclic compounds have presented antimicrobial activity and adverse effects on microbial community in soil.29,30 Nutrient depletion from soil microcosms was observed with incubation time increasing.31 The cease of chlorantraniliprole (CAP) was likely caused by depletion of available micronutrients (such as nitrogen and phosphorus), resulting in the death or dormancy of the microbial population degrading CAP.13 Therefore, the slow degradation rate of BIT (amide-containing heterocyclic compounds) in the later stage may be attributed to a decreased microbial activity due to the antimicrobial action of BIT, and depletion of available micronutrient such as nitrogen and phosphorus. The degradation of BIT in five unsterilized soils showed various half-lives during incubation, with the estimated t1/2 value of 0.09 d in soil #1, 1.89 d in soil #2, 1.23 d in soil #3, 3.44 d in soil #4, and 26.66 d in soil #5 (Table 2). The degradation rate showed a decreasing sequence soil #1 > soil #3 > soil #2 > soil #4 > soil #5, which is consistent with the change of OM content in soils. In conclusion, higher soil OM content is beneficial to BIT degradation, and this agrees with the report about the degradation of pyriproxyfen.32 Alternatively, since OM is a source of energy for soil microorganisms, higher OM content may promote the growth of BIT-degrading microbes. Thus, this result also confirmed that soil microorganisms are an important factor affecting BIT degradation in the soil.
3.1.2. Influence of soil microorganisms on the degradation of BIT. BIT degradation differences in sterilized and nonsterilized soils could be considered as the effect of soil microorganisms (Fig. 1 and Table 2). The dissipation rate of BIT under sterilized conditions (t1/2, 6.80–86.64 d) was lower than that under unsterilized conditions for each soil (t1/2, 0.09–26.66 d), implying that soil microorganisms played a significant role in the degradation of BIT. Similar observations for other pesticides have also been reported.6,13,33 Additionally, degradation under sterilized conditions may be attributed to the recovery of soil microbes and enzymes after autoclaving,34,35 as well as abiotic degradation. Overall, biological degradation is a major mechanism for BIT dissipation in the soil, which is mediated by soil microbes capable of degrading BIT and assisted by abiotic processes.
3.1.3. Influence of flooding on the degradation of BIT. In this study, 5 mL of distilled water was added to raise the level of water to approximately 1 cm above the soil surface to simulate the field flooded conditions. According to previous investigations,36,37 anaerobic conditions occur rapidly when the soil is flooded, resulting in great changes in dominant microbial communities. The dissipation of BIT under flooded (anaerobic) conditions was faster with t1/2 of 0.20–4.53 d, compared to unsterilized (aerobic) conditions (t1/2, 0.09–26.66 d) for each tested soils except soil #1 (Fig. 1 and Table 2). This result showed that flooded (anaerobic) conditions accelerated the microbial degradation of BIT in the soil, and this is similar to the report on tricyclazole.7 Additionally, this finding further suggested that anaerobic microbes dominate BIT degradation under flooded conditions.
3.2. Degradation products of BIT in soil
3.2.1. Identification of transformation products. Major degradation products of BIT in unsterilized soil #1 during incubation for 24 h were analyzed and identified using UHPLC-HRMS. Post-acquisition data were mined using the software Compound Discoverer 2.0 to screen possible metabolites and hypothesize the molecular formula of metabolites. In this study, two transformation products were observed and analyzed in addition to the BIT parent compound, which are labeled here as TP 1 and TP 2. Their structures were hypothesized and identified based on the molecular ion [M + H]+, retention time, fragmentation pattern from the MS2 spectra, and comparison with mass spectral data of the corresponding metabolite standard under the same UHPLC-HRMS conditions. Molecular weights of metabolites were obtained using full scan analysis in a positive mode with high-mass accuracy (error ratio of <3.0 ppm). Structure and fragmentation information are shown in Table 3.
Table 3 The structure and fragmentation information for parent compound and degradation products
Product ID |
Product name |
Mw |
Chemical structure |
ESI(+) MS m/z |
ESI(+) M2 m/z |
Parent |
BIT |
151.01 |
 |
152.01707 |
134.00636, |
105.03390 |
109.01106 |
124.02221 |
TP 1 |
2-Hydroxybenzamide |
137.05 |
 |
138.05550 |
121.02883, |
120.04482, |
93.03384, |
65.03889 |
TP 2 |
1,2-Benzisothiazole |
135.01 |
 |
136.02207 |
109.01117 |
103.04203 |
65.03888 |
BIT had a retention time of 7.42 min, and its mass spectrum showed that ion fragments of BIT were at m/z 152.01707 [M + H]+, including daughter ions of 134.00636 ([M + H]+ – H2O), 124.02221 ([M + H]+ – CO), 109.01106 ([M + H]+ – CONH), and 105.03387 ([M + H]+ – SNH) (Fig. 2A).
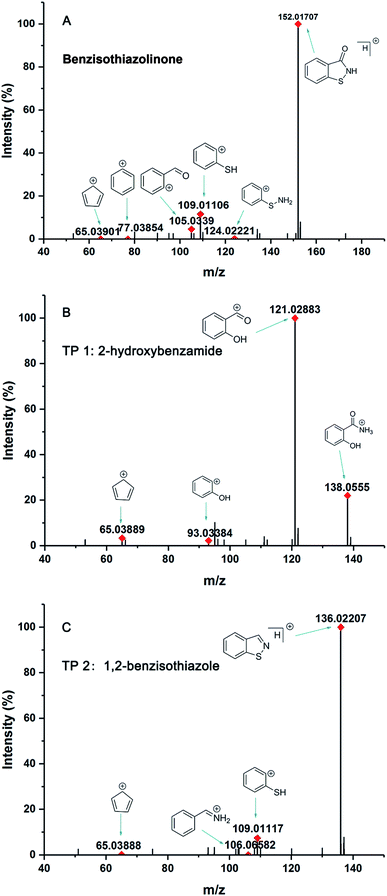 |
| Fig. 2 The mass spectrum of BIT and metabolites in the identification process: (A) BIT, (B) TP 1, (C) TP 2. | |
TP 1 with a UHPLC relative retention time of 6.76 min, matched formula C7H7NO2. According to MS2 spectra of the metabolites, TP 1 with a molecular ion of 138.05550 was identified as 2-hydroxybenzamide, as indicated by the characteristic ion at m/z 121.02883 (Fig. 2B). The generation of fragment ion at m/z 121.02883 could have resulted from the loss of an amino group ([M + H]+ – NH3), and m/z 93.03384 was indicative of phenol. Additionally, a fragment ion at m/z 120.04482 with the loss of 18 mass units was likely generated from the loss of a water molecule.
TP 2 with a UHPLC relative retention time of 9.04 min, matched formula C7H5NS. The mass of major fragments at m/z 110.01436, 109.01117, 106.06582, 95.04954, and 65.03888 was identical to BIT fragments (Fig. 2C). Based on similar fragmentation patterns, which were compared with the structure and mass spectrum of the parent BIT, the proposed structure for TP 2 with the protonated molecular ion at m/z 136.02207 [M + H]+ was identified as 1,2-benzisothiazole. Mass fragment ions at m/z 106.06582 and 109.01117 indicated sulfur atom and C
N bond losses, respectively.
Additionally, to further verify the structure of TP 2, 1,2-benzisothiazole was synthesized, and identified by 1H and 13C NMR (Fig. S2 and S3†). 1,2-Benzisothiazole and 2-hydroxybenzamide were analyzed using UHPLC-HRMS under the same conditions as the soil samples. Results showed that TP 1, its characteristic fragment ion peaks (m/z = 121.02883), and retention time were consistent with the MS spectrum of 2-hydroxybenzamide. TP 2 had the same retention time as 1,2-benzisothiazole, which was further confirmed by the presence of ions with m/z = 106.06582, 109.01117, and 136.02207.
3.2.2. Degradation pathway of BIT in soils. The peak areas of two metabolites over the incubation time are presented in Fig. 3, which reflects the trend of metabolites concentration during degradation. TP 2 emerged at the beginning of incubation and was detected during the entire incubation period. TP 1 emerged at an incubation time of 4 h, then reached its maximum concentration at 12 h. Based on the information of the identified intermediates, the possible degradation pathway of BIT in soils under unsterilized condition was proposed. BIT degradation in soil mainly occurred on the thiazole ring. The cleavage of five membered ring via the S–N bond, then followed the conversion of hydrosulphonyl to a hydroxyl to produce TP 1. TP 2, 1,2-benzisothiazole, was likely generated from a reduction of the carbonyl group and then dehydration, thereby resulting in the formation of a double bond between carbon and nitrogen.
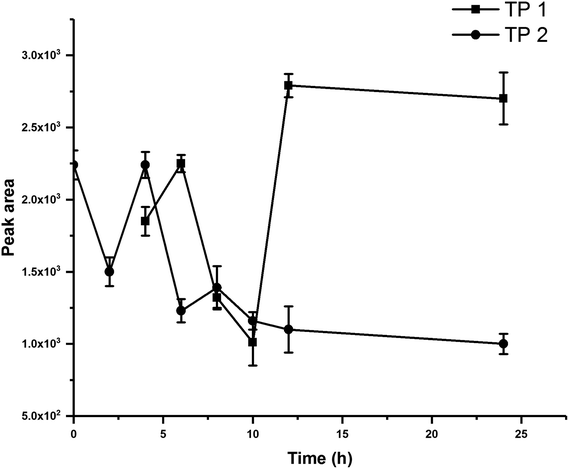 |
| Fig. 3 Peak area–time curves of TP 1 and TP 2 in soil #1. The error bars represent the standard deviation. | |
3.3. Sorption–desorption of BIT in soils
3.3.1. Sorption kinetics. The sorption process of BIT on tested soils involved two steps, an initial rapid sorption stage on the most accessible sites followed by slower and gradual sorption on less accessible sites to equilibration stage (Fig. 4A). Sorption equilibrium times for BIT differed among these five soils. In soil #1, BIT attained sorption equilibrium very quickly within 4 h. However, in soils #2, #3, #4, and #5, BIT reached equilibrium between 12–24 h; increases in the sorption amount after 24 h were insignificant, approximately 82.09% (soil #1), 58.31% (soil #2), 51.66% (soil #3), 43.32% (soil #4), and 39.84% (soil #5) of BIT was absorbed within 24 h at an initial concentration of 5 mg L−1. The different sorption equilibrium times and sorption amount at equilibrium for each soil may be due to variations in physicochemical properties of soils. Soil sorption of BIT was non-linear with respect to time (Fig. 4A), perhaps because of the heterogeneity of retention reactions and variable affinities of the organic matter sites for sorption of the pesticides.38 Initial surface sorption is fast sorption to easily accessible soil particle sites, while diffusion to internal sites is slower sorption to less accessible sites.39 The fast sites are filled first, and may be limited in number, while slower sorption most likely occurs simultaneously with fast sorption, and continues until equilibrium is reached. A period of 24 h was sufficient to achieve sorption equilibrium for all tested soils; therefore, it was taken as the equilibrium time of BIT and used in subsequent sorption–desorption isotherm experiments.
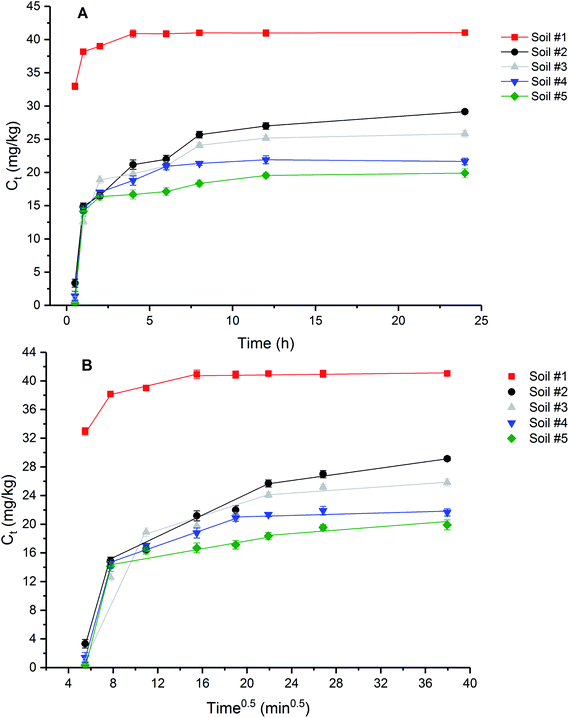 |
| Fig. 4 Sorption kinetics of BIT to five different soils: sorption of BIT to each soil as a function of contact time at concentration of 5 mg L−1 (A). Intraparticle diffusion model of BIT on five agricultural soils (B). The error bars represent the standard deviation. | |
Sorption kinetics provides useful information about the sorption mechanism of BIT. In this study, sorption kinetics data were fitted to pseudo-first-order, pseudo-second-order and intraparticle diffusion models (Tables S3 and S4†). In the case of sorption preceded by diffusion through a boundary, adsorption kinetics followed the pseudo-first-order model.40 The pseudo-second-order model is based on the hypothesis that the sorption capacity is directly proportional to the number of active sites on the sorbent.41 The sorption kinetics for soils #3, #4, and #5 followed the pseudo-first-order model (R2 = 0.9445, 0.9609, and 0.8724) better than the pseudo-second-order model (R2 = 0.6700, 0.9039, and 0.1871), and the calculated amounts of BIT sorbed (Cs,cal) using the pseudo-first-order kinetic model were closest to the experimental value (Cs,exp). This result indicated that the sorption of BIT to soils #3, #4, and #5 was preceded by diffusion. Sorption kinetics data of soils #1 and #2 fitted the pseudo-second-order kinetic model well, as confirmed by high correlation coefficients (R2 = 1 and 0.9865) and the Cs,cal calculated from the equation close to the Cs,exp obtained from experiment. This result suggested that the sorption capacity of BIT in soils #1 and #2 is controlled by the number of available active sites on the soil.
In terms of intraparticle diffusion plots in the present study, it is obvious that the sorption process of BIT on test soils present multilinearity indicating three stages occur in the sorption processes (Fig. 4B). The parameters in the three linear portions of each plot calculated by the intraparticle diffusion equation were listed in Table S4.† It was found that all the Ci values (intercept) were nonzero. Based on the diffusion theory, intraparticle diffusion is considered to be an only rate-controlling step if the intraparticle diffusion plot is linear and passes through the origin (Ci = 0),42 otherwise more than one mechanisms were involved in the sorption process.43 Therefore, the three-stage linear sorption process and the nonzero Ci values indicate that BIT sorption to the tested soils involved multiple steps. Based on previous studies,44,45 the first step was controlled by film diffusion (or external surface sorption) with a high rate because of sufficiently sorption sites, while the second step was associated with intraparticle diffusion when external surface sites were occupied and the adsorbate molecule transferred through inner soil pores. The third step was the equilibrium stage when the intraparticle diffusion gradually slowed down. The good linear regression indicates that intraparticle diffusion occurred in the stages 1 and 2 of BIT sorption on all five soils, and this also applies to the third stage of BIT sorption on soils #2, #3 and #5. However, the relatively lower R2 values of BIT sorption step 3 onto soil #1 and #4 indicates that no more intraparticle diffusion is expected in the last stage of the sorption process. Higher intercept (Ci) usually indicates the thicker boundary layer and the stronger boundary layer effect.46 In present study, soil #1 showed the largest intercept (Fig. 4B), indicating that the boundary layer effect was greater than that of other soils, which may be related to the higher OM content. In addition, the intraparticle diffusion rate (kp2) was lower than the film diffusion rate (kp1) (Table S4†), indicating that the BIT diffusion towards the soil external surfaces is faster compared to the intraparticle diffusion in step 2, and also indicating the BIT sorption to the agricultural soils was related to both intraparticle diffusion and boundary layer diffusion, but the former was the rate-limiting step.
3.3.2. Sorption isotherms. In this study, linear, Freundlich, and Langmuir models were used to describe the BIT sorption isotherms in soils. Freundlich sorption model was used to describe the multilayer sorption equilibrium on heterogeneous surfaces.47 while the Langmuir model is often employed to describe ideal sorption to a homogeneous sorbent.48 The sorption isotherms are shown in Fig. S4,† and corresponding parameters are summarized in Table 4. Due to the highest sorption in soil #1, the Ce values were less than the LOQ, so isothermal data for soil #1 were not obtained. Results showed that sorption isotherm data of BIT for other four soils (soils #2, #3, #4, and #5) fitted the Freundlich sorption model with higher regression coefficients (R2) of 0.8831–0.9826, compared with the linear models (R2 > 0.7328 for all samples except for soil #2 R2 = 0.3060) and Langmuir sorption model (R2 > 0.8245 for all samples except for soil #5 R2 = 0.1928). Thus, BIT sorption to these four soils was non-ideal sorption to heterogeneous sorbents, which is consistent with the DBP (di-n-butyl phthalate) sorption to different soil fractions.49 The 1/n values of the Freundlich model are used to characterize the linearity of an isotherm, which is convex, linear, and concave when 1/n < 1, 1/n = 1, and 1/n > 1, respectively.50 In this study, the 1/n values of the Freundlich model ranged between 0.31–1.12 (Table 4). For soils #2 and #3, the values of 1/n (0.31, 0.46) were significantlly <1, suggesting nonlinear and convex BIT sorption isotherms, in which sorption decreased with increasing BIT concentrations. However, the values of 1/n (0.85, 1.12) for soils #4 and #5 were close to 1, indicating the linearity of BIT sorption isotherm in soils #4 and #5, which were consistent with the results obtained from the linear sorption model with R2 of 0.9078 and 0.9940, respectively. This result also suggested the affinity of BIT sorption to soils #4 and #5 in the tested concentration range was relatively constant, similar results were found for perfluorooctanoic acid sorption isotherms to all soil fractions.51
Table 4 Sorption–desorption parameters of BIT in different soils
Models |
Parameters |
#1a |
#2 |
#3 |
#4 |
#5 |
For soil #1, due to the highest sorption in soil #1, the Ce values at equilibrium were less than the LOQ, thus isothermal data for soil #1 were not be obtained. |
Linear sorption |
Kd |
— |
2.06 |
2.34 |
2.31 |
2.11 |
R2 |
— |
0.3060 |
0.7328 |
0.9078 |
0.9940 |
log KOC |
— |
3.76 |
3.79 |
4.11 |
4.19 |
Freundlich sorption |
Kf |
— |
8.60 |
6.81 |
3.63 |
1.83 |
R2 |
— |
0.9733 |
0.8831 |
0.9598 |
0.9826 |
1/n |
— |
0.31 |
0.46 |
0.85 |
1.12 |
ΔG° |
— |
−23.68 |
−22.92 |
−23.22 |
−22.21 |
Langmuir sorption |
KL |
— |
3.56 |
0.90 |
0.15 |
— |
R2 |
— |
0.9943 |
0.8531 |
0.8245 |
0.1928 |
Qmax |
— |
15.17 |
18.38 |
31.65 |
— |
Freundlich desorption |
Kfd |
— |
15.93 |
22.77 |
5.24 |
23.98 |
R2 |
— |
0.6760 |
0.8022 |
0.9833 |
0.9106 |
1/nfd |
— |
0.24 |
0.37 |
0.55 |
0.84 |
H |
— |
0.77 |
0.81 |
0.65 |
0.75 |
The Kf values—Freundlich sorption distribution coefficients—illustrate the sorption affinity between BIT and tested soils. The Kf values of BIT sorption to tested soils varied between 1.83–8.60 (Table 4). To illustrate the effects of the physicochemical properties of the soils on BIT sorption, the relationships between physicochemical properties of soils and Kf values of the Freundlich model were analyzed. Multiple linear regression analysis between Kf value and physicochemical properties showed that OM content and pH values are main factors affecting the sorption of BIT on soils (Kf = 1.20 × OM − 2.06 × pH + 17.14, R2 = 0.9400). This result indicated that Kf values had positive correlations with OM content, while had negative correlations with pH, and the effect of pH on BIT sorption is greater than that of OM. Soil OM is formed from plants, animals and microbes residues, which include humus (fulvic acid, humic acid and humin) and non-humus (such as protein, hydrocarbon, organic acid, sugar and fat). Non-ionic organic compounds can be adsorbed to the hydrophobic surface (including beeswax, fat, resin, aliphatic side chain of humic acid and fulvic acid) of soil organic matter through hydrophobic interaction.52 Therefore, higher OM contents may provide more active sites for BIT (non-ionic organic compound) sorption via hydrophobic interactions. Additionally, humus contains a large number of acidic groups, high pH value will increase the degree of ionization of humus and reduce the tendency to form hydrophobic area.53,54 Therefore, higher pH value is not conducive to the sorption of BIT.
Organic carbon content-normalized sorption coefficients (log
KOC) were calculated to evaluate the BIT sorption capacities of different soils. In this study, log
KOC was 3.76–4.19 (Table 4). Based on the Test Guidelines on Environmental Safety Assessment for Chemical Pesticides in China,55 pesticides are classified into five types, highly adsorbed pesticide (log
KOC > 4.30), subhighly adsorbed pesticide (3.70 < log
KOC ≤ 4.30), medium-adsorbed pesticide (3.00 < log
KOC ≤ 3.70), sub-difficult adsorbed pesticide (2.30 < log
KOC ≤ 3.00), and difficult adsorbed pesticide (log
KOC < 2.30). Therefore, BIT was ranked as a sub-highly adsorbed pesticide. This result indicated that BIT presents a relatively high affinity and low mobility in the soil, consequently exhibiting a low potential for groundwater contamination.
3.3.3. Sorption thermodynamics. The standard free energy change, ΔG°, was determined to elucidate the sorption mechanisms of BIT. In this study, the values of ΔG° were negative for investigated soil samples, varying from −22.21 to −23.68 kJ mol−1 (Table 4), which showed that the sorption of BIT to soils was spontaneous and feasible, and the degree of spontaneity increased with increasing temperature. Additionally, the sorption process is considered a physical sorption when the value of ΔG° ranged from −20 to 0 kJ mol−1, whereas it was a chemical sorption when ranged from −400 to −80 kJ mol−1.56,57 In this study, the ΔG° values were close to −20 kJ mol−1, implying that the sorption of BIT to soil was a physical process.
3.3.4. Desorption isotherms. Freundlich isotherms well described the desorption data of BIT in soils #2, #3, #4, and #5, as indicated by the R2 of 0.6760–0.9833 (Table 4 and Fig. S4†). Values of 1/nfd for the desorption process were <1, implying that the relationship between BIT concentration and its desorption on these four tested soil samples was nonlinear. All tested soils had an average desorption rate less than 4.49%, which showed that BIT did not readily desorb. Additionally, the values of desorption distribution coefficient (Kfd) ranged between 5.24–23.98, which were consistently higher than those obtained for the sorption Kf on tested soils, confirming that the combination of BIT to soils was stronger, similar to previous study for non-steroidal anti-inflammatory drugs.58 Higher Kfd values indicated lower desorption of BIT, thus, the desorption capacity of BIT was in the decreasing order soil #4 > soil #2 > soil #3 > soil #5. This result showed that the desorption rate of BIT decreased with an increase in OM contents. The hysteresis coefficient, H, measures the extent of hysteresis in the desorption procedure. Previous studies emphasized that a value of H < 1 means that the rate of desorption is lower than that of sorption.59,60 Moreover, Barriuso et al. reported that no hysteresis effect exists when 0.7 < H < 1.61 In this study, H values for BIT were 0.65–0.81, close to 1, indicating that the desorption rate is lower than the sorption, and showing an insignificant desorption hysteresis effect.
4. Conclusions
The degradation of BIT in all the tested soils fitted the first order kinetics and increased with soil OM content. Degradation differences between unsterilized natural and sterilized soils suggested that BIT degradation is primarily driven by biological processes and assisted by abiotic degradation. The fastest dissipation rate of BIT under flooded conditions implied that anaerobic microorganisms are more likely to degrade BIT compared to aerobic microbes. During the soil degradation process, two metabolites were monitored and identified for the first time, which are 1,2-benzisothiazole and 2-hydroxybenzamide respectively. The sorption of BIT was a spontaneous physical process with no desorption hysteresis effect, and was enhanced by soil OM content. Based on relatively strong sorption to soils and low persistence in soils, it can be inferred that the potential risk of groundwater contamination by BIT may be low. Present study may be helpful to guide BIT reasonable use and decrease its potential risks for human and animals, and provides a feasible method of effectively identifying metabolites of BIT in soils. To fully understand the fate of BIT in the environment, further investigation should be conducted on the specific microbial community degrading BIT.
Conflicts of interest
There are no conflicts to declare.
Acknowledgements
This work was supported by the Science and Technology support program of Guizhou Province [grant number (2019)2347]; and the Program of Introducing Talents to Chinese Universities [grant number 111 Program, D20023].
References
- J. Cooper and H. Dobson, Crop Protect., 2007, 26, 1337–1348 CrossRef CAS.
- E. F. G. C. Dores, C. A. Spadotto, O. L. S. Weber, R. D. Villa, A. B. Vecchiato and A. A. Pinto, J. Agric. Food Chem., 2016, 64, 3942–3948 CrossRef CAS.
- C. Trigo, K. Spokas, K. Hall, L. Cox and W. C. Koskinen, J. Agric. Food Chem., 2016, 64, 3141–3149 CrossRef CAS.
- R. P. Sabale, A. T. P. Shabeer, S. Dasgupta, S. C. Utture, K. Banerjee, D. P. Oulkar, P. G. Adsule and M. B. Deshmukh, Environ. Monit. Assess., 2015, 187, 436–447 CrossRef.
- D. M. Pavlović, A. Glavač, M. Gluhak and M. Runje, Chemosphere, 2018, 193, 635–644 CrossRef.
- K. E. Hall, C. Ray, S. J. Ki, K. A. Spokas and W. C. Koskinen, J. Environ. Manage., 2015, 159, 227–234 CrossRef CAS.
- N. Kumar, I. Mukherjee, B. Sarkar and R. K. Paul, J. Hazard. Mater., 2017, 321, 517–527 CrossRef CAS.
- N. Kumar, I. Mukherjee and E. Varghese, Environ. Monit. Assess., 2015, 187, 60–69 CrossRef.
- X. Ma, X. Liu, S. Ding, S. Su and Z. Gan, Chemosphere, 2019, 224, 519–526 CrossRef CAS.
- J. Ou, H. Li, X. Ou, Z. Yang, M. Chen, K. Liu, Y. Teng and B. Xing, Ecotox. Environ. Safe., 2020, 205, 1–7 CrossRef.
- C. Wei, X. Song, Q. Wang and Z. Hu, Ecotox. Environ. Safe., 2017, 142, 40–50 CrossRef CAS.
- Y. Liu, L. Lonappan, S. K. Brar and S. Yang, Sci. Total Environ., 2018, 645, 60–70 CrossRef CAS.
- Z. C. Redman, S. J. Parikh, M. J. Hengel and R. S. Tjeerdema, J. Agric. Food Chem., 2019, 67, 8130–8137 CrossRef CAS.
- Z. Cai, W. Zhang, S. Li, J. Ma, J. Wang and X. Zhao, J. Agric. Food Chem., 2015, 63, 3823–3829 CrossRef CAS.
- F. Yu, W. Liu and Q. Lei, Agrochemicals, 2009, 48, 130–131 CAS.
- Y. Ni, W. Yan, J. Geng and J. Zhao, Fine Spec. Chem., 2004, 12, 21–22 CAS.
- Z. Zhao, Q. Ge, Y. Niu and F. Zhang, Agrochemicals, 2015, 54, 518–520 CAS.
- R. M. Novick, M. L. Nelson, K. M. Unice, J. J. Keenan and D. J. Paustenbach, Food Chem. Toxicol., 2013, 56, 60–66 CrossRef CAS.
- USEPA/Office of Prevention, Pesticides and Toxic Substances, Reregistration Eligibility Decision Document for 1,2-Benzisothiazolin-3-one (BIT), EPA 739-R-05-007, 2005, http://www.epa.gov/pesticides/reregistration/status.htm Search PubMed.
- USEPA/Office of Pesticide Programs, Pesticide Ecotoxicity Database, 2013, as cited in the ECOTOX database, 2013, https://ecotox.ipmcenters.org/ Search PubMed.
- Y. Su, J. Zhou, R. Meng, N. Li, Y. Li and Q. Luo, Chin. J. Pesticide Sci., 2016, 18, 135–140 CAS.
- J. L. Dang, M. L. Gleason, C. K. Niu, X. Liu, Y. Z. Guo, R. Zhang and G. Y. Sun, Plant Dis., 2017, 101, 568–575 CrossRef CAS.
- X. Liu, B. Song, X. Jiang, Y. Yang, P. Lu and D. Hu, Int. J. Environ. Anal. Chem., 2019, 1–12 Search PubMed.
- State Forestry Administration, LY/T 1225-1999: Determination of forest soil particle-size composition (mechanical composition), 1999 Search PubMed.
- State Forestry Administration, LY/T 1237-1999: Determination of organic matter in forest soil and calculation carbon-nitrogen ratio, 1999 Search PubMed.
- State Forestry Administration, LY/T 1239-1999: Determination of pH value in forest soil, 1999 Search PubMed.
- State Forestry Administration, LY/T 1243-1999: Determination of cation exchange capacity in forest soil., 1999 Search PubMed.
- L. Xiang, T. Xiao, C. H. Mo, H. M. Zhao, Y. W. Li, H. Li, Q. Y. Cai, D. M. Zhou and M. H. Wong, Ecotox. Environ. Safe., 2018, 154, 84–91 CrossRef CAS.
- M. Wu, G. Li, X. Chen, J. Liu, M. Liu, C. Jiang and Z. Li, Sci. Total Environ., 2018, 616, 236–244 CrossRef.
- M. Wu, J. Liu, W. Li, M. Liu, C. Jiang and Z. Li, Ecotoxicol. Environ. Saf., 2017, 144, 409–415 CrossRef CAS.
- P. L. E. Bodelier, A. P. Hahn, I. R. Arth and P. Frenzel, Biogeochemistry, 2000, 51, 225–257 CrossRef.
- H. Liu, X. Yi, J. Bi, P. Wang, D. Liu and Z. Zhou, J. Hazard. Mater., 2019, 365, 97–106 CrossRef CAS.
- M. A. Dar, G. Kaushik and J. F. Villarreal-Chiu, J. Environ. Manage., 2019, 239, 124–136 CrossRef CAS.
- K. L. Williams, J. J. Gladfelder, L. L. Quigley, D. B. Ball and R. S. Tjeerdema, J. Agric. Food Chem., 2017, 65, 9200–9207 CrossRef CAS.
- D. O. Carter, D. Yellowlees and M. Tibbett, Pedobiologia, 2007, 51, 295–299 CrossRef CAS.
- S. Gao, K. K. Tanji, S. C. Scardaci and A. T. Chow, Soil Sci. Soc. Am. J., 2002, 66, 805–817 CrossRef CAS.
- M. Noll, D. Matthies, P. Frenzel, M. Derakshani and W. Liesack, Environ. Microbiol., 2005, 7, 382–395 CrossRef CAS.
- H. Zhu and H. M. Selim, Soil Sci., 2002, 167, 513–523 CrossRef CAS.
- C. M. Cooke, G. Shaw, J. N. Lester and C. D. Collins, Sci. Total Environ., 2004, 329, 197–213 CrossRef CAS.
- A. Verma, S. Kumar and S. Kumar, J. Environ. Chem. Eng., 2016, 4, 4587–4599 CrossRef CAS.
- Y. P. Teoh, M. A. Khan and T. S. Y. Choong, Chem. Eng. J., 2013, 217, 248–255 CrossRef CAS.
- G. Mckay and J. Chem, Technol. Biot., 1983, 33, 196–204 Search PubMed.
- R. Rojas, E. Vanderlinden, J. Morillo, J. Usero and H. E. Bakouri, Sci. Total Environ., 2014, 488, 124–135 CrossRef.
- J. L. Marco Browna, L. Guza, M. S. Olivellia, B. Schamperab, R. M. Torres Sánchezc, G. Curutcheta and R. Candal, Chem. Eng. J., 2018, 333, 495–504 CrossRef.
- M. Doğan, H. Abak and M. Alkan, J. Hazard. Mater., 2009, 164, 172–181 CrossRef.
- D. M. Pavlovic, L. Curkovic, D. Blazek and J. Zupan, Sci. Total Environ., 2014, 497, 543–552 CrossRef.
- M. Vhahangwele and G. W. Mugera, J. Environ. Chem. Eng., 2015, 3, 2416–2425 CrossRef CAS.
- I. Langmuir, J. Am. Chem. Soc., 1918, 40, 1361–1402 CrossRef CAS.
- L. Xiang, X. D. Wang, X. H. Chen, C. Mo, Y. W. Li, H. Li, Q. Y. Cai, D. M. Zhou, M. H. Wong and Q. X. Li, J. Agric. Food Chem., 2019, 67, 4734–4745 CrossRef CAS.
- K. M. Doretto, L. M. Peruchi and S. Rath, Sci. Total Environ., 2014, 476–477, 406–414 CrossRef CAS.
- L. Xiang, T. Xiao, P. F. Yu, H. M. Zhao, C. Mo, Y. W. Li, H. Li, Q. Y. Cai, D. M. Zhou and M. H. Wong, J. Agric. Food Chem., 2018, 66, 11569–11579 CrossRef CAS.
- A. Walker and D. V. Crawford, Isotopes and radiation in soil organic matter studies, Int. Atomic Energy Agency, Vienna, 1968 Search PubMed.
- J. L. Zhou, S. Rowland and R. F. C. Mantoura, Water Res., 1994, 28, 571–579 CrossRef CAS.
- R. R. Engebretson, T. Amos and R. V. Wandruszka, Environ. Sci. Technol., 1996, 30, 990–997 CrossRef CAS.
- Standardization Administration of China, Test guidelines on the environmental safety assessment for chemical pesticides-Part 4: Adsorption/desorption in soils, GB/T 31270.4-2014, 2014 Search PubMed.
- Y. Wu, J. He and L. Yang, Environ. Sci. Technol., 2010, 44, 6319–6324 CrossRef CAS.
- C. Lei, Y. Y. Hu and M. Z. He, Chem. Eng. J., 2013, 219, 361–370 CrossRef CAS.
- Y. Zhang, G. W. Price, R. Jamieson, D. Burton and K. Khosravi, Chemosphere, 2017, 174, 628–637 CrossRef CAS.
- M. Kumar and L. Philip, Chemosphere, 2006, 62, 1064–1077 CrossRef CAS.
- X. G. Liu, F. S. Dong, J. Xu, S. K. Yuan and Y. Q. Zheng, J. Integr. Agric., 2014, 13, 2471–2478 CrossRef CAS.
- E. Barriuso, D. A. Laird, W. C. Koskinen and R. H. Dowdy, Soil Sci. Soc. Am. J., 1994, 58, 1632–1638 CrossRef CAS.
Footnote |
† Electronic supplementary information (ESI) available. See DOI: 10.1039/d0ra09553b |
|
This journal is © The Royal Society of Chemistry 2021 |