DOI:
10.1039/D0RA09289D
(Paper)
RSC Adv., 2021,
11, 2706-2717
A novel red-emitting phosphor Mg2Y2Al2Si2O12:Ce3+/Mn2+ for blue chip-based white LEDs
Received
1st November 2020
, Accepted 7th December 2020
First published on 13th January 2021
Abstract
Traditional white light-emitting diodes (LEDs) (blue chip + YAG:Ce3+ yellow phosphor) have the limitation of red deficiency, which limits their application in the illumination field. The single cation/anion substitution or co-doping of activators can increase the red component; however, the large energy loss is attributed to the ultra-long Stokes shift and energy transfer. This work attempts to utilize the short-distance Stokes shift and a small amount of energy transfer to increase the red component in two steps. First, based on a large number of previous research results, the Mg2Y2Al2Si2O12:Ce3+ phosphor is selected. Second, additional enhancement of the red component in the emission spectrum was achieved by ion co-doping Mn2+ into Mg2Y2Al2Si2O12:Ce3+. The emission peaks for samples Mg2Y2Al2Si2O12:Ce3+,Mn2+ shift from 600 to 635 nm with increase in the concentration of Mn2+, and the emission spectra intensity of Mg1.97Y1.93Al2Si2O12:0.07 Ce3+,0.03 Mn2+ anomalously increased by ∼37%, which was attributed to the increase in the distance between Ce3+ ions because of the doping of Mn2+ ions, and reduction in the concentration of defects in the crystal, resulting in the energy loss decreases of Ce3+. The emission peak of Mg1.97Y1.93Al2Si2O12:0.07 Ce3+,0.03 Mn2+ shifts to 618 nm and the quantum efficiency was as high as 83.07%. Furthermore, this sample has high thermal stability and the emission intensity was still 80.14% at 120 °C. As such, it has great potential in the application of white LEDs.
1 Introduction
Traditional white LEDs (the blue-chip + YAG:Ce3+ yellow phosphor) have superior properties of high brightness and low cost and are extensively used in transportation, lighting displays, medical devices, electronic products and other fields. However, because of the lack of red-light components, color rendering and color temperature of white LEDs is insufficient to meet the increasing lighting requirements. For example, in the field of high-color restored lighting or warm white lighting, it cannot compete with other white lighting solutions.1–6 In general, the red light component of the phosphor can be increased by co-doping other activating ions, e.g., Jia et al. reported the YAG:Ce3+,Mn2+ phosphor with the two emission peaks at 540 nm (Ce3+) and 594 nm (Mn2+), which increases the red component of the emission with increasing Mn2+ concentration.7 However, adding sufficient red components requires Ce3+ to transfer a large amount of energy to enhance the emission intensity of Mn2+ ions.8–11 However, it causes a large amount of energy loss, which reduces the overall luminous intensity of the phosphor. Furthermore, the substitution of anions/cations to adjust the crystal field environment of Ce3+ ions can regulate the emission spectrum of Ce3+ ions to the long-wave direction and increase the red component. A large number of researchers have adjusted the emission spectrum of YAG:Ce3+ by this method and obtained a large number of results to achieve the movement of the emission peak from 532 to 622 nm.12–14 However, with the redshift of the emission spectrum, its Stokes shift increases, the quenching effect increases, and the emission intensity of the phosphor gradually decreases, which limits the application of the phosphor. The energy loss caused by the ultra-long Stokes shift and a large amount of energy transfer is extremely large. Either method cannot obtain high-efficiency phosphors alone; this work attempts to combine two methods to prepare a highly efficient red phosphor in two steps. Firstly, initially improve the red component of Ce3+ ion emission spectrum using structural fine-tuning, while ensuring that the phosphor has relatively strong luminescence intensity. Pan et al. reported an orange (600 nm) emitting phosphor Mg2Y2Al2Si2O12:Ce3+ with quantum efficiency as high as 58.3%;15 as such, the Mg2Y2Al2Si2O12:Ce3+ phosphor is selected based on a large number of previous research results. Second, additional enhancement in the red component of the emission spectrum by co-doping Mn2+ with Mg2Y2Al2Si2O12:Ce3+; moreover, it is desired to obtain a highly efficient red phosphor. Finally, when the doping concentration of Mn2+ is 0.03, the emission peak of Mg2Y2Al2Si2O12:Ce3+,Mn2+ shifts to 618 nm, and the quantum efficiency is as high as 83.07%. Furthermore, the emission intensity of the sample Mg1.97Y1.93Al2Si2O12:0.07 Ce3+,0.03 Mn2+ is still 80.14% at 120 °C, indicating that this sample has a higher thermal stability. By mixing this sample with YAG:Ce3+, a warm white light with a high color index of 78 and abundant red light components is obtained, stimulated by the 460 nm-sized blue chip. It has great potential in applying white LEDs.
2 Sample preparation and characterization
A series of Mg2Y2Al2Si2O12, Mg2−yY1.93Al2Si2O12:0.07 Ce3+,yMn2+(y = 0, 0.01, 0.02, 0.03, 0.04, 0.06, 0.08, 0.12, 0.16) and Mg1.97Y2−xAl2Si2O12:xCe3+,0.03 Mn2+ (x = 0, 0.001, 0.005, 0.01, 0.03, 0.05, 0.07) samples were synthesized by a high temperature solid-state method. The raw materials were MgO (A.R.), Y2O3 (99.999%), Al2O3 (A.R.), SiO2 (A.R.), CeO2 (99.999%), and MnCO3 (A.R.). Firstly, the raw materials were weighed as per the stoichiometric ratio, and then they were placed in an agate mortar and ground for 20 min; the ground powders were placed in a corundum, and then placed in a box furnace for sintering by heating to 1650 °C and held for 5 h. Finally, the samples were naturally cooled to room temperature, and the samples were taken out and placed in an agate mortar for grinding to obtain powder samples for the subsequent measurements.
2.1 Phase structure characterization
The phase structures of the samples were characterized using a Bruker D8 X-ray diffractometer. The radiation source was Cu target Kα (λ = 0.15406 nm), and the working voltage and current were 40 kV and 40 mA, respectively. The scan range 2θ and the step size were 10–80° and 0.02° s−1, respectively.
2.2 Optical properties
The steady-state spectra of the samples were measured using a Hitachi F-4600 fluorescence spectrometer. The sample thermoluminescence spectra (TL) were measured using an FJ-427A1 type thermoluminescence meter, and the measurement range and heating rate were 50–300 °C and 1 °C s−1, respectively. The samples were irradiated for 5 min under UV light and then placed in a dark room for 10 min before thermoluminescence measurements were taken.
2.3 Fluorescence lifetime and quantum efficiency
The lifetimes of samples were monitored using a Horiba FL3 instrument. The lifetime of Ce3+ was achieved using 320 and 455 nm nano-LEDs as exciting sources, and the lifetime of Mn2+ was measured using a Xe lamp as the light source. The quantum efficiencies of samples were measured using the Hitachi F-7000 fluorescence spectrophotometer and a Hitachi 5J0-0148 integrating sphere.
3 Results and discussion
3.1 XRD pattern and crystal structure
Fig. 1(a) shows the unit cell of Mg2Y2Al2Si2O12. There are three different lattice positions in the stability of the garnet structure. The Y/Mg atom has eight coordination oxygen atoms together to form a dodecahedron with two different bond lengths. The length of the four shorter keys is m and the length of four longer keys is n. The Y/Mg atom occupies 24(c) sites in the unit cell. The Mg/Al atom has six coordination oxygen atoms together to form an octahedron, which has the same bond length p and occupies 16(a) sites in the unit cell. The Al/Si atom occupies the 24(d) site and has four coordinating oxygen atoms to form a tetrahedron, which has the same bond length q. Fig. 1(b) shows the XRD patterns of representative samples Mg2Y2Al2Si2O12, Mg1.97Y2Al2Si2O12:0.03 Mn2+, Mg2Y1.93Al2Si2O12:0.07 Ce3+, Mg1.97Y1.93Al2Si2O12:0.07 Ce3+,0.03 Mn2+ and Mg1.84Y1.93Al2Si2O12:0.07 Ce3+,0.16 Mn2+. The XRD diffraction peak positions of the synthetic samples match well with the diffraction peak in the standard card Y3Al5O12 (ICSD#20090), indicating that all the samples have a single-crystal phase and that the Ce3+ and Mn2+ ions do not destroy the crystal structure. The XRD patterns of samples are subjected to refinement using the standard card Y3Al5O12 (ICSD#20090) as a standard, and the obtained refinement data are within the accurate range and are shown in Fig. 2–4.
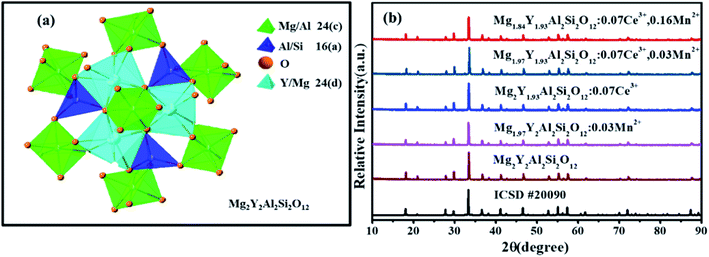 |
| Fig. 1 (a) The unit cell of Mg2Y2Al2Si2O12. (b) XRD patterns of Mg2Y2Al2Si2O12, Mg1.97Y2Al2Si2O12:0.03 Mn2+, Mg2Y1.93Al2Si2O12:0.07 Ce3+, Mg1.97Y1.93Al2Si2O12:0.07 Ce3+,0.03 Mn2+, Mg1.84Y1.93Al2Si2O12:0.07 Ce3+,0.16 Mn2+. | |
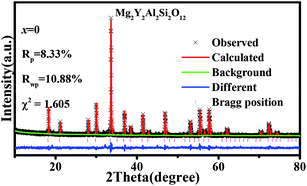 |
| Fig. 2 XRD refinement of Mg2Y2Al2Si2O12. | |
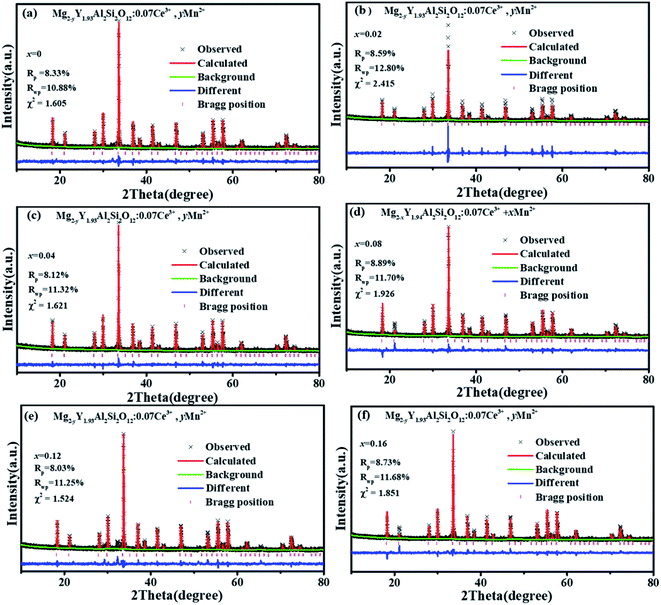 |
| Fig. 3 XRD refinement of Mg2−yY1.93Al2Si2O12:0.07 Ce3+,yMn2+ (y = 0, 0.02, 0.04, 0.12, 0.16). | |
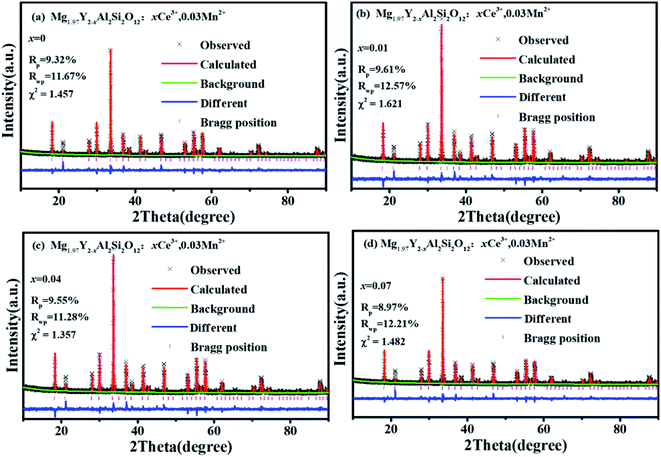 |
| Fig. 4 XRD refinement of Mg1.97Y2−x Al2Si2O12:xCe3+,0.03 Mn2+ (x = 0, 0.01, 0.03, 0.07). | |
3.2 Luminescence properties of Mg2Y1.93Al2Si2O12:0.07 Ce3+ and Mg1.97Y2Al2Si2O12:0.03 Mn2+
Fig. 5(a) and (b) show the excitation spectra and emission spectra of Mg2Y1.93Al2Si2O12:0.07 Ce3+ two luminescence centers. The luminescence centers come from the Ce3+ ions substituting Y3+ ions to occupy eight coordination sites.15 The emission spectra of Ce3+in fluorides and oxides can generally move from the ultraviolet region to the blue region. However, in the environment of strong covalent and crystal field, the 5d energy level of Ce3+ ions can move to a lower energy range; therefore, Ce3+ can emit yellow or even red fluorescence color.7,8,16 In the study of sulfides and nitrides, the 5d energy levels of Ce3+ions can be divided into two to five energy levels, and the number of separations depend on the symmetry of the lattice containing Ce3+ligands. In the cubic symmetry crystal structure, such as garnet structure, the 5d state is split into two energy levels (eg and t2g), and the crystal field can be split further in the twisted cubic crystal.17–22 The unique longwave emission (green yellow-orange) of Ce3+ in the garnet is attributed to the splitting of the crystal field of the energy level eg into a lower 5d1 level and a higher energy 5d2 level with an interval of about 8000 cm−1. It is precise because of this energy level splitting the emission from the highest energy 5d2 level is in the blue-violet spectral region; the emission from the lowest energy 5d1 level is in the green yellow-orange spectral region.23–27 It is seen from Fig. 5(a), under the excitation of 332 nm, the emission spectrum of Ce3+ extends from 350 to 700 nm, and the emission peak is located at 405 nm. By monitoring the 405 nm emission peak, the excitation spectrum ranges from 200 to 400 nm, and the strongest excitation peak is 332 nm, which is formed by the electron transition from the ground state to 5d2 energy level. The tailing of 500–650 nm comes from the emission spectrum of electrons from 5d2 level relaxing to 5d1 level and then downward. In Fig. 5(b), under the excitation of 470 nm, it is seen that the emission spectrum extends from 500 nm to 800 nm and the emission peak is at 600 nm. For the 600 nm emission peak, the corresponding excitation spectrum extends from 200 nm to 550 nm. It is obvious that there are two excitation peaks in the excitation spectrum: one is ∼332 nm, which is attributed to the electronic transition from the ground state to the 5d2 level, and the other one is at 470 nm, which is attributed to the electron transition from the ground state to the 5d1 level.
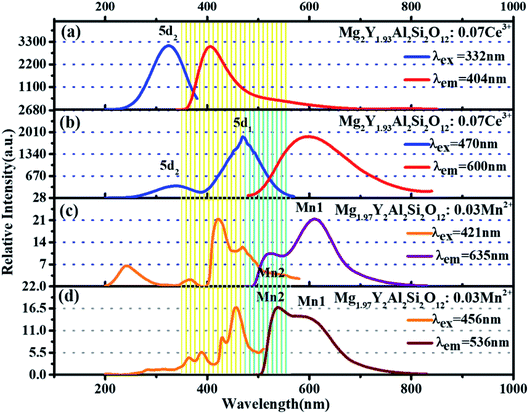 |
| Fig. 5 (a and b) Excitation spectra and emission spectra of Mg2Y1.93Al2Si2O12:0.07 Ce3+. (c and d) Excitation spectra and emission spectra of Mg1.97Y2Al2Si2O12:0.03 Mn2+. | |
In the previous work, it has been proven that Mn2+ ions can occupy the tetracoordinate, hexacoordinate and octagonal sites of Mg2Y2Al2Si2O12 and emit green light (536 nm), red light (635 nm) and deep red light (735 nm). When the amount of Mn2+ is > 0.2, the deep red light will be generated. The purpose of this work is to develop a red-light-compensated phosphor; hence, the amount of Mn2+ ions is not >0.2. Fig. 5(c) and (d) show the excitation spectra and emission spectra of Mg1.97Y2Al2Si2O12:0.03 Mn2+. According to the literature, Mn ions can occupy four, six and eight coordination in garnet structure.42 In this work, Mn2+ occupies eight coordination sites to form the luminescence center Mn1. Under the excitation of 421 nm, the emission spectrum extends from 470 to 700 nm, and the primary peak is located at 635 nm. By monitoring the 635 nm emission peak, the excitation spectrum from 200 nm to 500 nm is obtained, and the peak is at 421 nm. The 536 nm emission peak is derived from the luminescence center Mn2 formed by Mn2+ occupying a tetracoordinate position, as shown in Fig. 5(d). For the luminescence center Mn2, the emission spectrum extends from 500 to 750 nm under the 456 nm excitation radiation; furthermore, the peak is at 536 nm. For the 536 nm emission peak, a broad excitation band is observed from 200 nm to 500 nm, and the primary peak is at 456 nm.
Fig. 5 show that the emission spectrum of Ce3+ overlaps with the excitation spectrum of Mn2+ in the yellow- and green-shaded region and that the luminescence center formed by Ce3+ may transfer energy to the luminescence centers Mn1 and Mn2. Moreover, the emission peak of Mn2+ ion is closer to the long-wave direction; furthermore, the red component of Mg2Y1.93Al2Si2O12:0.07 Ce3+ can be enhanced by co-doping Mn2+. In addition, under the same measurement conditions, the ratio of the relative emission intensity of Ce3+ to Mn2+ is ∼100
:
1.
3.3 Luminescence properties of Mg1.97Y1.93Al2Si2O12:0.07 Ce3+,0.03 Mn2+
Fig. 6(a) shows the emission spectrum of Mg1.97Y1.93Al2Si2O12:0.07 Ce3+,0.03 Mn2+ under the 470 nm excitation and the primary peak is at 618 nm. Compared with the emission peak of Mg2Y1.93Al2Si2O12:0.07 Ce3+ is at 600 nm in Fig. 5(b), the emission spectrum of Mg1.97Y1.93Al2Si2O12:0.07 Ce3+,0.03 Mn2+ results in a significant redshift. No characteristic emission spectrum of Mn2+ is observed in the spectrum, and the characteristic excitation of Mn2+ ions could not be observed by monitoring the emission peaks of 635 (Mn1) and 536 nm (Mn2). It may be because the luminescence intensity of Mn2+ is considerably smaller than the luminescence intensity of Ce3+. To avoid this interference, the emission spectrum of the sample Mg1.97Y1.93Al2Si2O12:0.07 Ce3+,0.03 Mn2+ is measured under the excitation of 332 nm. Fig. 6(b) shows the 635 nm characteristic emission of Mn1 with the 332 nm excitation, which indicates that Mn2+ can normally emit. To further confirm that Mn2+ ions form luminescence centers in Mg1.97Y1.93Al2Si2O12:0.07 Ce3+,0.03 Mn2+, the lifetimes of Mn1 and Mn2 are measured; furthermore, the lifetimes can be calculated using the following formula28–30 |
 | (1) |
I refers to the emission intensity of the illuminating center at time t, t is time, and τ is the lifetime. The calculated lifetimes of Mn1 and Mn2 are 3.63 and 0.97 ms, respectively, as shown in Fig. 6(c). It is confirmed that Mn2+ ions do form the luminescent centers in the sample and that the emission spectra of the sample Mg1.97Y1.93Al2Si2O12:0.07 Ce3+,0.03 Mn2+ is attributed to the interaction of Ce3+ and Mn2+ luminescence centers.
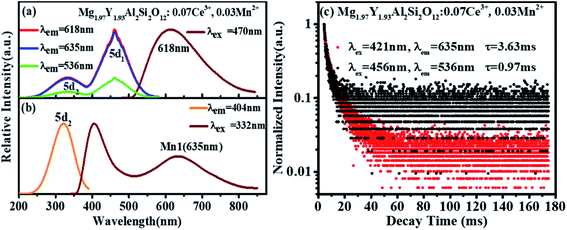 |
| Fig. 6 (a and b) Excitation spectra and emission spectra Mg1.97Y1.93Al2Si2O12:0.07 Ce3+,0.03 Mn2+; (c) lifetime decay spectra of Mn1 and Mn2 in Mg1.97Y1.93Al2Si2O12:0.07 Ce3+,0.03 Mn2+. | |
Fig. 7(a) shows the emission spectra of Mg2−yY1.93Al2Si2O12:0.07 Ce3+,yMn2+ under 470 nm excitation. There is an obvious red shift, which can be observed with Mn2+ concentration increased, and the emission peaks of Mg2−yY1.93Al2Si2O12:0.07 Ce3+,yMn2+ shifting from 600 to 635 nm, as shown in Fig. 7(b). The reasons may be explained below:
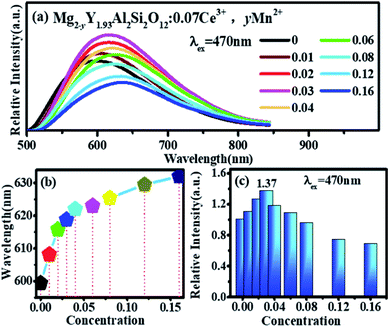 |
| Fig. 7 (a) Emission spectra of Mg2−yY1.93Al2Si2O12:0.07 Ce3+,yMn2+ (y = 0, 0.01, 0.02, 0.03, 0.04, 0.06, 0.08, 0.12, 0.16) (λex = 470 nm). (b) A shift diagram of the emission peaks of Mg2−yY1.93Al2Si2O12:0.07 Ce3+,yMn2+ (y = 0, 0.01, 0.02, 0.03, 0.04, 0.06, 0.08, 0.12, 0.16) (λex = 470 nm). (c) A change in emission intensity of Mg2−yY1.93Al2Si2O12:0.07 Ce3+,yMn2+ (y = 0, 0.01, 0.02, 0.03, 0.04, 0.06, 0.08, 0.12, 0.16) (λex = 470 nm). | |
(1) When doped with Mn2+ ions, the crystal structure of the sample changes, resulting in a change in the emission spectra of Ce3+, which causes the redshift of the emission spectra.
(2) Ce3+ ions transfer energy to the luminescence center of Mn1, and the emission intensity of Mn1 increases, which causes the emission spectra to move toward the long-wave direction.
For specific reasons, the following experiments were performed.
3.4 Analyze the conjecture (1)
Under the 470 nm excitation, the emission spectrum of Mn1 is indistinguishable from the large-area overlap of the emission spectrum of the Ce3+ 5d1 level. To reduce the effect of the emission spectrum of the 5d1 level, the emission spectra of the samples were measured under 332 nm excitation. Fig. 8(a) shows that the emission peak of the right emission spectrum is at 635 nm, which is attributed to the luminescence center Mn1. As the doping concentration of Mn2+ increases, the crystal structure may change; furthermore, the emission spectrum of Mn1 shifts to the long-wave direction. Because Ce3+ and Mn1 occupy the same position, the emission spectrum of Ce3+ may move in the longwave direction with increase in Mn2+ doping concentration. Furthermore, verifying the above conclusions from the perspective of the crystal structure. Many factors describe the change in the crystal field; however, the most influential two factors are the average bond length of the polyhedral unit cell and the degree of distortion of the unit cell. The relationship between the average bond length and the splitting of the luminescent center level orbit can be calculated using the following formula31 |
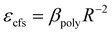 | (2) |
εcfs is the crystal field splitting and βpoly is a constant, which depends on the type of polyhedron; generally, βocta = 1.35 × 109 pm2 cm−1 (octahedron). The βpoly of other polyhedral cells can be derived from the octahedral βocta. βcub = 0.89βocta (cube), βdh = 0.79βocta (dodecahedron). R−2 is the average bond length; therefore, the degree of the orbital splitting of the activated ions increases as R decreases.
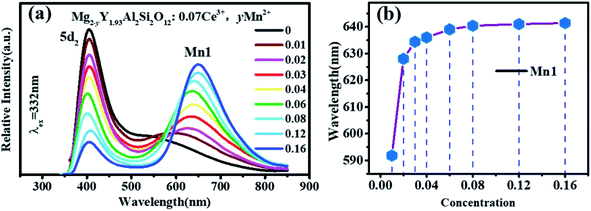 |
| Fig. 8 (a) The emission spectra of the samples Mg2−yY1.93Al2Si2O12:0.07 Ce3+,yMn2+ (y = 0, 0.01, 0.02, 0.03, 0.04, 0.06, 0.08, 0.12, 0.16) at 332 nm excitation; (b) the emission peak shifts of the Mn1 emission spectra. | |
As for the crystal distortion, the change in the bond length and bond angle in the crystal can cause the symmetry of the crystal decreasing and lead to the crystal field to split. Conversely, the symmetry of crystal increasing indicates the distortion degree of the crystal decreasing, which reduces the split in the crystal field. It can be calculated using the formula (3),32,33 and the degree of distortion is affected by the bond length
|
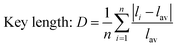 | (3) |
where
li represents the bond length of the central atom and the ligand,
lav represents the average bond length, and
n represents the coordination number.
Table 1 shows the XRD refinement data of Mg
2−yY
1.93Al
2Si
2O
12:0.07 Ce
3+,
yMn
2+ (
y = 0, 0.02, 0.04, 0.08, 0.12, 0.16) and the calculated distance between Ce
3+ ions. The energy level splitting and distortion of Mg
2−yY
1.93Al
2Si
2O
12:0.07 Ce
3+,
yMn
2+ (
y = 0, 0.02, 0.04, 0.08, 0.12, 0.16) was calculated and the data is shown in
Table 2. The crystal field splitting decreases with an increase in the Mn
2+ concentration; however, at the same time, the unit cell distortion gradually increases, which causes the energy level splitting to increase. In fact, the crystal distortion in the dodecahedral structure of the garnet has a stronger influence on the luminescence center, which makes the emission spectrum shift toward the long-wave direction. The above analyses indicate that speculation (1) is correct. When doped with Mn
2+ ions, the crystal structure of the sample changes, resulting in the emission redshift of Ce
3+ and Mn
2+.
Table 1 XRD refinement data of Mg2−yY1.93Al2Si2O12:0.07 Ce3+,yMn2+ (y = 0, 0.02, 0.04, 0.08, 0.12, 0.16) and the calculated distance between Ce3+ ions
x |
0 |
0.02 |
0.04 |
0.08 |
0.12 |
0.16 |
χ2 |
1.605 |
2.415 |
1.621 |
1.926 |
1.524 |
1.851 |
Rwp |
10.88% |
12.8% |
11.32% |
11.7% |
11.25% |
11.68% |
Rp |
8.3% |
8.59% |
8.12% |
8.89% |
8.03% |
8.73% |
a/b/c (Å) |
11.990 |
11.992 |
11.993 |
11.994 |
11.995 |
11.997 |
V (Å3) |
1723.665 |
1724.339 |
1724.819 |
1725.282 |
1725.900 |
1726.763 |
Eight-coordinated bond lengths m (Å) |
2.313 |
2.313 |
2.315 |
2.317 |
2.319 |
2.321 |
Eight-coordinated bond lengths n (Å) |
2.4265 |
2.428 |
2.431 |
2.435 |
2.439 |
2.443 |
Table 2 The energy level splitting and distortion of Mg2−yY1.93Al2Si2O12:0.07 Ce3+,yMn2+ (y = 0, 0.02, 0.04, 0.08, 0.12, 0.16)
Concentration |
Energy level splitting εcfs (109 pm2 cm−1) |
Distortion D |
0 |
0.1899 |
0.0239 |
0.02 |
0.1898 |
0.0243 |
0.04 |
0.1894 |
0.0244 |
0.08 |
0.1889 |
0.0248 |
0.12 |
0.1885 |
0.0253 |
0.16 |
0.1880 |
0.0255 |
3.5 Analyze the conjecture (2)
It can be clearly seen from Fig. 8(a) that the Ce3+ ions transfer energy to Mn1 under excitation at 332 nm. However, under excitation at 470 nm, the emission spectra of Mn1 cannot be distinguished from the 5d1 emission spectra of Ce3+. To confirm that there is an energy transfer between the 5d1 emission spectra of Ce3+ and the emission spectra of Mn1; the lifetime changes of Ce3+ and Mn2+ luminescent centers were measured. The lifetimes of Mn1, Mn2, the electron of 5d1 and 5d2 (using 320 nm and 455 nm external LED pulse lamps) level in Mg1.97Y2−xAl2Si2O12:xCe3+,0.03 Mn2+ and Mg2−yY1.93Al2Si2O12:0.07 Ce3+,yMn2+ were measured with an increase in the Mn2+ concentration. Fig. 9(a) shows the lifetimes of the luminescence center Mn1. The results show that the lifetimes of the luminescence center Mn1 gradually increases with increase in Ce3+ concentration.
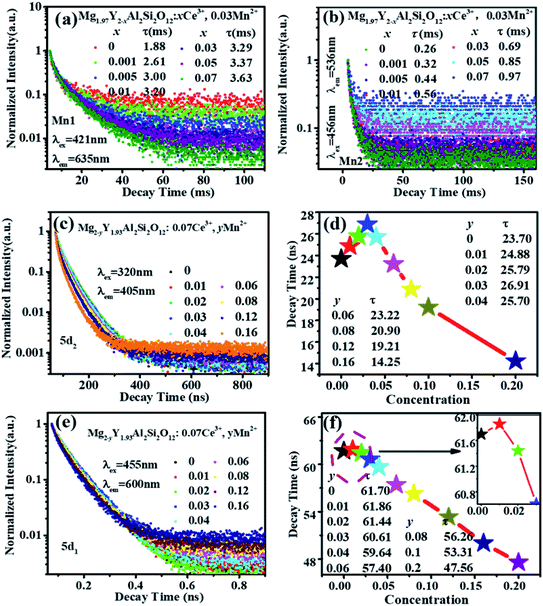 |
| Fig. 9 (a) Lifetimes of Mn1 luminescence center in Mg1.97Y2−xAl2Si2O12:xCe3+,0.03 Mn2+ (λex = 421 nm, λem = 635 nm); (b) the lifetimes of Mn2 luminescence center in Mg1.97Y2−xAl2Si2O12:xCe3+,0.03 Mn2+ (λex = 456 nm, λem = 536 nm); (c and d) the lifetimes of the 5d2 level electrons of Ce3+ in Mg2−yY1.93Al2Si2O12:0.07 Ce3+,yMn2+ and the corresponding line graph (λex = 320 nm, λem = 405 nm); (e and f) the lifetime of the 5d1 level of Ce3+ in Mg2−yY1.93Al2Si2O12:0.07 Ce3+,yMn2+ and the corresponding line graph (λex = 455 nm, λem = 600 nm). | |
Fig. 5 shows that the emission spectrum of Mn2 overlaps with the excitation and emission spectra of Ce3+ ions, indicating that the photon energy emitted by the two is close. Therefore, Mn2 may transfer energy to Ce3+ ions, and Ce3+ may transfer energy to Mn2. The experiment verified the relevant information, and a large number of studies reported that Ce3+ transfers energy to Mn2+ and examples of Mn2+ ion transferring energy to Ce3+ have not been found.34–39 Furthermore, the experiment measured the lifetime change of Mn2 luminescence center with Ce3+ doping, as shown in Fig. 9(b). With increase in Ce3+ ion doping concentration, the lifetime of Mn2 luminescence center has a slight increase, indicating that Ce3+ is in the material and it is possible to transfer energy to Mn2.
Fig. 9 (c–f) show the lifetimes of the 5d1 and 5d2 level electrons of Ce3+ in Mg2−yY1.93Al2Si2O12:0.07 Ce3+,yMn2+. When the Mn2+ concentration is increased, the lifetimes of Ce3+ decrease overall (the abnormality of lifetimes is explained in detail below). Consequently, Ce3+ ions transfer energy to Mn2+ ions, and the emission intensity of Mn1 increases, which causes the emission spectra of the samples Mg2−yY1.93Al2Si2O12:0.07 Ce3+,yMn2+ to move toward the long-wave direction.
Therefore, the primary reasons for the spectral shift are as follows: (1) as the Mn2+ ion concentration increases, the distortion of the dodecahedral unit cell increases such that the 5d1 level of Ce3+ splitting and the crystal field splitting of Mn1 luminescence center increase, the emission spectra move toward the direction of long waves. (2) The Ce3+ luminescence center transferred energy to the Mn1 luminescence center, and the luminescence intensity of Mn1 increases, which causes the emission spectra to move toward the long-wave direction. Under the combined action of the two, the emission spectra move toward the long-wave direction as the Mn2+ concentration increases.
Moreover, as shown in Fig. 8(a) and 6(a), the energy transfer of Ce3+ to Mn1 is more efficient than the Mn2 luminescence center, which indicated that Ce3+ has little effect on the luminescence properties of Mn2. This may be attributed to Mn2 being located at the center of the fully symmetric tetrahedron, which greatly weakens the influence of external Mn2 luminescence center. Conversely, it can be considered that the effect of Mn2 on the overall luminescent property of Mg2−yY1.93Al2Si2O12:0.07 Ce3+,yMn2+ is weak.
In Fig. 7(a) and (c), under the excitation of 470 nm, the emission intensities of Mg2−yY1.93Al2Si2O12:0.07 Ce3+,yMn2+ abnormally increases with increase in Mn2+ concentration. In Fig. 5, under the same test conditions (photomultiplier voltage is 460 V, excitation end slit width is 2.5 nm, and receiving end slit width is 2.5 nm), the relative emission intensity of Mn2+ is much smaller than that of Ce3+. The emission intensities of Mn2+ are negligible compared to the overall Ce3+ emission spectra. Though Ce3+ can transfer energy to the Mn1 luminescence center, the energy utilization efficiency must be generally <100%, which will lose energy. Namely, the number of photons absorbed by the Mn1 luminescence center will be higher than the number of photons radiated. If there are no other factors, as the Mn2+ concentration increases, the transfer energy increases and the energy loss increases under the 470 nm excitation; therefore, the overall emission intensity of the sample should gradually decrease. However, it is observed that the emission intensities of the samples abnormally increase under the 470 nm excitation with an increase in the Mn2+ concentration and reach a maximum at 0.03, as shown in Fig. 7(c). It can be inferred that other factors cause an abnormal increase in the luminescence intensity of samples under the same excitation radiation. The luminescence centers in the sample are only Ce3+ and Mn2+; hence, the possibilities causing the enhanced abnormal luminescence intensity of Mg2−yY1.93Al2Si2O12:0.07 Ce3+,yMn2+ are as follows:
(1) The luminescence intensity of the Mn2+ luminescence center itself (excluding the energy transfer of Ce3+) in the Mg2−yY1.93Al2Si2O12:0.07 Ce3+,yMn2+ is considerably improved compared to luminescence intensity in the Mg2−yY2Al2Si2O12:yMn2+. Thus, the emission intensity of co-doping of Ce3+ and Mn2+ is higher than that of single-doped Ce3+ ions under 470 nm excitation.
(2) Mn2+ doping into Mg2Y1.93Al2Si2O12:0.07 Ce3+ increases the emission intensity of Ce3+ ions itself, which improves the overall luminescence intensity.
Next, it will be proved and explained one by one. Fig. 6 shows that the characteristic excitation and emission of Mn1 and Mn2 cannot be observed under excitation of 470 nm when co-doped with Ce3+ and Mn2+, which indicates that the luminescence efficiency of Mn2+ is relatively weak. Therefore, reasons (1) and (3) are excluded. As such, the reason for the abnormal enhancement of the emission of the sample may be the conjecture (2): Mn2+ ions doping into Mg2Y1.93Al2Si2O12:0.07 Ce3+ increase the emission intensity of Ce3+ ions, which improves the overall luminescence intensity. The details of the energy loss between Ce3+ ions can be described as Ce3+ acts as an active ion in the host because the activated ions at the same excited states are close enough with increasing activator concentration, which can easily result in energy transfer among the energy states. Due to the energy transfer between activator and activator, the energy will go through the quenching center of impurity and be consumed in the lattice vibration of the host, which can further result in a decrease in the emission intensity. In addition, the activating Ce3+ ions and defects in the matrix can be used as quenching centers.
Therefore, three factors can improve the emission intensity of Ce3+ ions: other ions transfer energy to Ce3+; or the energy loss of Ce3+ decreases the quenching center, or Ce3+ transferred energy to other luminescent centers decreases. The change of the Ce3+ lifetimes can reflect the energy change of the Ce3+ luminescence center. This work analyzes the lifetime change of Ce3+ ions to determine which factor affects the energy change of the Ce3+ luminescence center. This relationship can be expressed by the following formula40
|
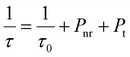 | (4) |
where
τ represents the lifetime of the luminescent center,
τ0 describes the lifetime of the free ion of the luminescent center and is constant.
Pnr represents the probability of a non-radiative transition of the luminescent center under the action of the quenching center (negative), and
Pt means the probability that the illuminating center absorbs (positively) or outward (negatively). In
Fig. 9(d) and (f), the lifetimes of the 5d
1 and 5d
2 level electrons of Ce
3+ in the sample Mg
2−yY
1.93Al
2Si
2O
12:0.07 Ce
3+,
yMn
2+ increase at first, reaching the maximum at
y = 0.03, and then gradually decrease. No luminescence centers can transfer energy to Ce
3+, and Ce
3+ ions also transfer energy to Mn
2+ ions, so
Pt is negative. As the Mn
2+ concentration increases, the
Pt value decreases. When doped with a small number of Mn
2+ ions, the lifetime of Ce
3+ increases, which proves that there is a certain factor that makes the
Pnr to increase, namely, the energy loss of the Ce
3+ ion in the quenching center to decrease. The quenching center of Ce
3+ can be the other Ce
3+ ions surrounding the Ce
3+ luminescence center or the quenching center composed of defects in the matrix structure. So, the reasons for the reduction in energy loss of the corresponding Ce
3+ luminescence center are as the Mn
2+ concentration increases, the distance between Ce
3+ ions increase, which weakens the multipole interaction between Ce
3+ ions; Mn
2+ ions reduce the concentration of defects, the number of Ce
3+ quenching centers and the energy loss of the Ce
3+ luminescence center. Using the volume of the sample unit cell in
Table 1, the distances between the Ce
3+ ions were calculated using the formula for the critical distance between ions
41 |
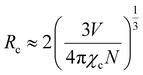 | (5) |
where
Rc is the distance between the doped particles,
V is the unit cell volume,
χc is the doping ion concentration, and N is the number of cations in the unit cell.
χc = 0.06,
N = 8, and the volume is obtained by refinement data, as shown in
Table 1. The distances between Ce
3+ ions are calculated to be 9.0299, 9.0300, 9.0302, 9.0305, 9.0308, 9.0311, which correspond to
x = 0, 0.02, 0.04, 0.08, 0.12, 0.16, respectively. The distances between Ce
3+ ions gradually increase, which means that the interaction probability between Ce
3+ ions decrease, and the energy loss of the Ce
3+ luminescence center is reduced.
The thermoluminescence intensity is used to characterize the number of defects in Mg2−yY1.93Al2Si2O12:0.07 Ce3+,yMn2+ (y = 0, 0.01, 0.02, 0.03, 0.04), as shown in Fig. 10. It can be seen that as the Mn2+ ions are doped into the material, the number of defects in the sample gradually decreases. Meanwhile, the defect-quenching center number of the Ce3+ may be decreasing, which reduces the energy loss of the Ce3+ luminescent center. Briefly, with doping of Mn2+ ions, the distance between Ce3+ particles increase, the interaction between Ce3+ particles decrease, the quenching center of defect formation decreases, and the energy loss of Ce3+ luminescence center decreases. At low concentration, the energy saved by the quenching center is more than the energy lost by the energy transfer, so that the emission intensities increase. Otherwise, when the doping concentration is relatively high, the energy saved by the quenching center is less than the energy lost by the energy transfer, hence the emission intensity decreases.
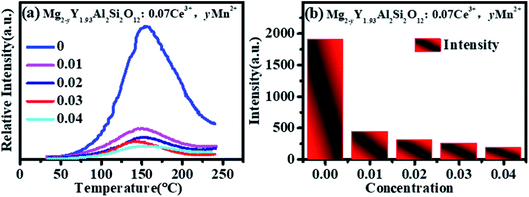 |
| Fig. 10 (a) Thermoluminescence spectra of Mg2−yY1.93Al2Si2O12:0.07 Ce3+,yMn2+ (y = 0, 0.01, 0.02, 0.03, 0.04). (b) The thermoluminescence intensity of Mg2−yY1.93Al2Si2O12:0.07 Ce3+,yMn2+ as a function of Mn2+ concentration. | |
3.6 Temperature stability and quantum efficiency
Fig. 11 shows that the emission intensity of Mg1.97Y1.93Al2Si2O12:0.07 Ce3+,0.03 Mn2+ gradually decreases with an increase in the temperature, under the excitation of 470 nm light. The emission spectrum intensity remains 80.14% at 120 °C, which means that it has a higher temperature stability.
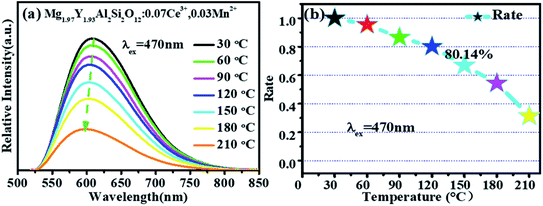 |
| Fig. 11 (a) Temperature spectra of Mg1.97Y1.93Al2Si2O12:0.07 Ce3+,0.03 Mn2+. (b) The emission intensity of Mg1.97Y1.93Al2Si2O12:0.07 Ce3+,0.03 Mn2+ as a function of temperature. | |
Fig. 12 shows the quantum efficiency of Mg2−yY1.93Al2Si2O12:0.07 Ce3+,yMn2+ (y = 0, 0.01, 0.02, 0.03, 0.04). Under the 470 nm excitation, the quantum efficiencies of the samples Mg2−yY1.93Al2Si2O12:0.07 Ce3+,yMn2+ increase at first and then decreases with increasing Mn2+ concentration, which is the same as the changing trend of the emission spectra. When doped with 0.03 Mn2+, the internal quantum efficiency is as high as 83.07%, which reaches the level of commercial application.
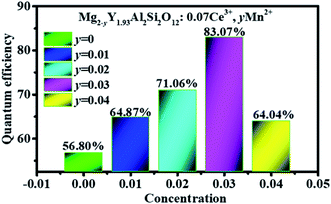 |
| Fig. 12 Quantum efficiency of Mg2−yY1.93Al2Si2O12:0.07 Ce3+,yMn2+ (y = 0, 0.01, 0.02, 0.03, 0.04). | |
To verify that Mg1.97Y1.93Al2Si2O12:0.07 Ce3+,0.03 Mn2+ phosphor can supplement the red component of blue-chip + YAG:Ce3+, the mixed phosphor of YAG:Ce3+ and Mg1.97Y1.93Al2Si2O12:0.07 Ce3+,0.03 Mn2+ was excited by the 460 nm chip, and a warm white LED with the color temperature of 3005 K was successfully obtained, as shown in Fig. 13. Moreover, the color rendering index was raised to 78, and the effect of adding red components was remarkable.
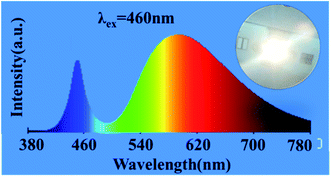 |
| Fig. 13 Mixed phosphor of YAG:Ce3+ and Mg1.97Y1.93Al2Si2O12:0.07 Ce3+,0.03 Mn2+ is excited by the 460 nm chip. | |
4 Conclusions
A series of Mg2Y2Al2Si2O12 and Mg2Y2Al2Si2O12 doped with Ce3+ and Mn2+ were synthesized by the high-temperature solid-phase method. Two phenomena about the emission spectra of the samples Mg2−yY1.93Al2Si2O12:0.07 Ce3+,yMn2+ are emphatically discussed. The one phenomenon is that the emission spectra are shifted from 600 nm to 635 nm under the excitation of 470 nm, wherein, two reasons are causing the spectra red-shift. Firstly, as the Mn2+ ions enter the crystal structure, the energy transfer from the Ce3+ ion to the Mn1, the emission intensity of Ce3+ decreases and the emission intensity of Mn1 increases, so the emission peak shift toward the long-wave direction. Secondly, with the entry of Mn2+ ions, crystal distortion of the dodecahedral unit cells of Ce3+ and Mn2+ increases, which leads to the energy level split of the Ce3+ and Mn1 luminescence center increasing, and the emission spectra shift toward the long wave. Another phenomenon is that the emission spectrum intensity of the sample Mg2Y2Al2Si2O12:Ce3+,Mn2+ anomalously increased by about 37%, compared with the emission spectrum intensity of the sample Mg2Y2Al2Si2O12:Ce3+, which is because that doping of Mn2+ ions increases the distance between the Ce3+ ions, and that lowers the concentration of defects in the crystal, resulting in the reduction in the energy loss of the Ce3+ quenching center. When the doping concentration of Mn2+ is 0.03, the emission peak of the sample Mg1.97Y1.93Al2Si2O12:0.07 Ce3+,0.03 Mn2+ shifts to 618 nm and the quantum efficiency was as high as 83.07%. In addition, this sample has high thermal stability, while the emission intensity was still at 80.14% at 120 °C. Finally, warm white light with a high color index of 78 was obtained with abundant red light components by mixing this phosphor with YAG:Ce3+, stimulated by a blue light chip. It has great potential in the application of white LEDs.
Conflicts of interest
The authors declare no competing financial interest.
Acknowledgements
The work is supported by the National Natural Science Foundation of China (No. 51672066, 51902080), the Funds for Distinguished Young Scientists of Hebei Province, China (No. A2018201101), the Natural Science Foundation of Hebei Province, China (No. E2019201223), the personnel training project of Hebei Province, China (No. A201902005), and the Local science and technology development fund projects guided by the central government, China (No. 206Z1102G).
References
- C. Wang, P. Li, Z. Wang, Y. Sun, J. Cheng, Z. Li, M. Tian and Z. Yang, Crystal structure, luminescence properties, energy transfer and thermal properties of a novel color-tunable, white light-emitting phosphor Ca9−x−yCe(PO4)7:xEu2+,yMn2+, Phys. Chem. Chem. Phys., 2016, 18(41), 28661–28673 RSC.
- M. Shang, C. Li and J. Lin, How to produce white light in a single-phase host?, Chem. Soc. Rev., 2014, 43(5), 1372–1386 RSC.
- S. Ye, F. Xiao, Y. Pan, Y. Ma and Q. Zhang, Phosphors in phosphor-converted white light-emitting diodes: Recent advances in materials, techniques and properties, Mater. Sci. Eng., R, 2010, 71(1), 1–34 CrossRef.
- C. C. Lin and R. S. Liu, Advances in phosphors for light-emitting diodes, J. Phys. Chem. Lett., 2011, 2(11), 1268–1277 CrossRef CAS.
- H. R. Chen, C. Cai and Z. W. Zhang, Enhancing the luminescent efficiency of Y3Al5O12:Ce3+ by coating graphitic carbon nitride: toward white light-emitting diodes, J. Alloys Compd., 2019, 801, 10–18 CrossRef CAS.
- L. J. Yin, Y. L. Liang and S. H. Zhang, A novel strategy to motivate the luminescence efficiency of a phosphor: drilling nanoholes on the surface, Chem. Commun., 2018, 54, 3480–3483 RSC.
- Y. Jia, Y. Huang, Y. Zheng, N. Guo, H. Qiao, Q. Zhao, W. Lv and H. You, Color point tuning of Y 3Al5O12:Ce3+ phosphor via Mn2+–Si4+ incorporation for white light generation, J. Mater. Chem., 2012, 22(30), 15146–15152 RSC.
- H. Liao, M. Zhao and M. S. Molokeev, Learning from a mineral structure toward an ultra-narrow-band blue-emitting silicate phosphor RbNa3(Li3SiO4)4:Eu2+, Angew. Chem., Int. Ed., 2018, 130(36), 11902–11905 CrossRef.
- M. Zhao, H. Liao and M. S. Molokee, Emerging ultra-narrow-band cyan-emitting phosphor for white LEDs with enhanced color rendition, Light: Sci. Appl., 2019, 8, 38 CrossRef.
- Y. Li, Y. Shi and G. Zhu, A single-component white-emitting CaSr2Al2O6:Ce3+,Li+,Mn2+ phosphor via energy transfer, Inorg. Chem., 2015, 45(14), 7668–7675 CrossRef.
- M. Jiao, Y. Jia and L. Wei, A single-phase white-emitting Ca2SrAl2O6:Ce3+,Li+,Mn2+ phosphor with energy transfer for UV-excited WLEDs, Dalton Trans., 2014, 43, 3202–3209 RSC.
- G. Blasse and A. Bril, A new phosphor for flying-spot cathode-ray tubes for color television: yellow-emitting Y3Al5O12–Ce3+, Appl. Phys. Lett., 1967, 11, 53–55 CrossRef CAS.
- A. A. Setlur, W. J. Heward, M. E. Hannah and U. Happek, Incorporation of Si4+–N3− into Ce3+-doped garnets for warm white LED phosphors, Chem. Mater., 2008, 20, 6277–6283 CrossRef CAS.
- X. Wang, G. Zhou, H. Zhang, H. Li, Z. Zhang and Z. Sun, Luminescent properties of yellowish orange Y3Al5−xSixO12−xNx: Ce phosphors and their applications in warm white light-emitting diodes, J. Alloys Compd., 2012, 519, 149–155 CrossRef CAS.
- Z. Pan, Y. Xu, Q. Hu, W. Li, H. Zhou and Y. Zheng, Combination cation substitution tuning of yellow-orange emitting phosphor Mg2Y2Al2Si2O12:Ce3+, RSC Adv., 2015, 5(13), 9489–9496 RSC.
- Y. C. Wu, D. Y. Wang and T. M. Chen, et al,. A novel tunable green-to yellow-emitting β-YFS:Ce3+ phosphor for solid-state lighting, ACS Appl. Mater. Interfaces, 2011, 3(8), 3195–3199 CrossRef CAS.
- P. Dorenbos, 5d-level energies of Ce3+ and the crystalline environment. I. Fluoride compounds, Phys. Rev. B: Condens. Matter Mater. Phys., 2000, 62(23), 15640 CrossRef CAS.
- P. Dorenbos, 5d-level energies of Ce3+ and the crystalline environment. II. Chloride, bromide, and iodide compounds, Phys. Rev. B: Condens. Matter Mater. Phys., 2000, 62(23), 15650 CrossRef CAS.
- P. Dorenbos, 5d-level energies of Ce3+ and the crystalline environment. III. Oxides containing ionic complexes, Phys. Rev. B: Condens. Matter Mater. Phys., 2001, 64(12), 125117 CrossRef.
- P. Dorenbos, Relating the energy of the [Xe] 5d1 configuration of Ce3+ in inorganic compounds with anion polarizability and cation electronegativity, Phys. Rev. B: Condens. Matter Mater. Phys., 2002, 65(23), 235110 CrossRef.
- P. Dorenbos, Crystal field splitting of lanthanide 4fn−15d-levels in inorganic compounds, J. Alloys Compd., 2002, 341(1–2), 156–159 CrossRef CAS.
- P. Dorenbos, 5d-level energies of Ce3+ and the crystalline environment. IV. Aluminates and “simple” oxides, J. Lumin., 2002, 99(3), 283–299 CrossRef CAS.
- Z. Xia and A. Meijerink, Ce3+-Doped garnet phosphors: composition modification, luminescence properties and applications, Chem. Soc. Rev., 2017, 46, 275 RSC.
- J. Ueda, K. Aishima and S. Tanabe, Temperature and compositional dependence of optical and optoelectronic properties in Ce3+-doped Y3Sc2Al3−xGaxO12 (x = 0, 1, 2, 3), Opt. Mater., 2013, 35, 1952–1957 CrossRef CAS.
- A. Setlur and A. Srivastava, On the relationship between emission color and Ce3+ concentration in garnet phosphors, Opt. Mater., 2007, 29, 1647–1652 CrossRef CAS.
- L. Zhang, J. Zhang, X. Zhang, Z. Hao, H. Zhao and Y. Luo, New yellow-emitting nitride phosphor SrAlSi4N7:Ce3+ and important role of excessive AlN in material synthesis, ACS Appl. Mater. Interfaces, 2013, 5, 12839–12846 CrossRef CAS.
- Y. C. Wu, D. Y. Wang, T. M. Chen, C. S. Lee, K. J. Chen and H. C. Kuo, A novel tunable green-to yellow-emitting β-YFS:Ce3+ phosphor for solid-state lighting, ACS Appl. Mater. Interfaces, 2011, 3, 3195–3199 CrossRef CAS.
- S. Lee, C. Huang, T. Chan and T. Chen, Crystal structure evolution and luminescence properties of color tunable solid solution phosphors Ca2+xLa8−x(SiO4)6−x(PO4)xO2:Eu2+, Dalton Trans., 2016, 45(3), 1007–1015 RSC.
- D. L. Dexter and J. H. Schulman, Theory of concentration quenching in inorganic phosphors, J. Chem. Phys., 1954, 22(6), 1063–1070 CrossRef CAS.
- T. Li, P. Li, Z. Wang, S. Xu, Q. Bai and Z. Yang, Structure, luminescence properties and energy transfer of Tb3+–Eu3+ co-doped LiBaB9O15 phosphors, Dalton Trans., 2015, 44(38), 16840–16846 RSC.
- J. Ueda, K. Aishima, S. Nishiura and S. Tanabe, Afterglow luminescence in Ce3+-doped Y3Sc2Ga3O12 ceramics, Appl. Phys. Express, 2011, 4, 042602 CrossRef.
- Y. Luo and Z. Xia, Effect of Al/Ga Substitution on Photo-luminescence and Phosphorescence Properties of Garnet-Type Y3Sc2Ga3−xAlxO12:Ce3+ Phosphor, J. Phys. Chem. C, 2014, 118, 23297–23305 CrossRef CAS.
- L. Chen, C. C. Lin, C. W. Ye and R. S. Liu, Light converting inorganic phosphors for white light-emitting diodes, Materials, 2010, 3, 2172–2195 CrossRef CAS.
- J. Si, L. Wang and L. Liu, Structure, luminescence and energy transfer in Ce3+ and Mn2+ codoped γ-AlON phosphors, J. Mater. Chem. C, 2019, 7, 733–742 RSC.
- W. Lv, Y. Jia and Q. Zhao, A novel tunable Na2Ba6(Si2O7)(SiO4)2:Ce3+,Mn2+ phosphor with excellent thermal stability for white light emitting diodes, RSC Adv., 2014, 4, 14074–14080 RSC.
- Z. Wang, S. Lou and P. Li, Enhanced orange–red emission of Sr3La(PO4)3:Ce3+,Mn2+ via energy transfer, J. Lumin., 2014, 156, 87–90 CrossRef CAS.
- K. Li, M. Shang and Y. Zhang, Photoluminescence properties of single-component white-emitting CaBi(PO4)7:Ce3+,Tb3+,Mn2+ phosphors for UVLEDs, J. Mater. Chem. C, 2015, 3(27), 7096–7104 RSC.
- N. Guo, Y. Huang and Y. Jia, A novel orange-yellow-emitting Ba3Lu(PO4)3:Eu2+,Mn2+ phosphor with energy transfer for UV-excited white LEDs, Dalton Trans., 2013, 42(4), 941–947 RSC.
- Z. Wang, S. Lou and P. Li, Enhanced orange–red emission of Sr3La(PO4)3:Ce3+,Mn2+ via energy transfer, J. Lumin., 2014, 156, 87–90 CrossRef CAS.
- G. Blasse, Energy transfer in oxidic phosphors, Phys. Lett. A, 1968, 28(6), 444–445 CrossRef CAS.
- J. Kacher, C. Landon, B. L. Adams and D. Fullwood, Bragg's law diffraction simulations for electron backscatter diffraction analysis, Ultramicroscopy, 2009, 109(9), 1148–1156 CrossRef CAS.
- J. A. Hodges, Temperature dependent epr measurements of Mn2+ in diamagnetic garnets, J. Phys. Chem. Solids., 1974, 35(10), 1385–1392 CrossRef.
|
This journal is © The Royal Society of Chemistry 2021 |