DOI:
10.1039/D0RA08496D
(Paper)
RSC Adv., 2021,
11, 2293-2297
A DFT study on the C–H oxidation reactivity of Fe(IV)–oxo species with N4/N5 ligands derived from L-proline†
Received
6th October 2020
, Accepted 8th December 2020
First published on 8th January 2021
Abstract
The hydroxylation of hexane by two FeIVO complexes bearing a pentadentate ligand (N5, Pro3Py) and a tetradentate ligand (N4, Pro2PyBn) derived from L-proline was studied by DFT calculations. Theoretical results predict that both FeIVO complexes hold triplet ground states. The hydrogen atom abstraction (HAA) processes by both FeIVO species proceed through a two-state reactivity, thus indicating that HAA occurs via a low-barrier quintet surface. Beyond the conventional rebound step, the dissociation path is also calculated and is found to potentially occur after HAA.
Introduction
Non-haem iron enzymes and their synthetic analogues have attracted considerable interest due to their ability for diverse catalytic oxidation in biological and chemical syntheses.1–5 In these oxidative transformations, Fe(IV)
O species are often implicated as key oxidizing intermediates.6–10 Of crucial interest is the effect of the spin state of the FeIV(O) unit; quintet (S = 2) or triplet (S = 1) ground states might be involved as predicted by density functional theory (DFT) calculations.11,12 For instance, a high-spin iron(IV)–oxo intermediate was spectroscopically characterized in α-ketoglutarate-dependent taurine dioxygenase (TauD).13 Additionally, the first X-ray crystal structure of a synthetic non-haem iron(IV)–oxo complex, namely [(TMC)FeIV(O)]2+ (TMC = 1,4,8,11-tetramethyl-1,4,8,11-tetraazacyclotetradecane), was reported in 2003.14 Inspired by these great achievements in both biological and biomimetic systems, a great number of mononuclear non-haem Fe(IV)
O complexes supported by polydentate ligands have been synthesized and intensively investigated to elucidate their structural, spectroscopic and reactive properties.6–10,15–19 These Fe(IV)
O complexes exhibit versatile reactivity (for example, enabling alkane hydroxylation) that markedly depends on the use of supporting ligands.16 In recent years, the Fe(IV)
O complex with a Me3NTB (tris(N-methylbenzimidazol-2-yl)-methyl)amine) ligand has been demonstrated as the most powerful oxidant among the intermediate-spin (S = 1) iron(IV)–oxo complexes.20 Moreover, a highly reactive high-spin (S = 2) complex [(TQA)FeIV(O) (CH3CN)]2+ (TQA = tris(quinolin-2-ylmethyl)amine) was prepared by replacing the pyridines of the TPA ligand with weaker-field quinolones.21 This intriguing difference in the spin state is strongly regulated by the structure of the supporting ligand, thus tuning the reactivity in the activation of C–H bonds.22 On the other hand, DFT studies have indicated a small energy gap in oxo–iron(IV) models between triplet and quintet ground states, thus enabling “two-state-reactivity” (TSR).23,24 For example, during the reaction of [N4PyFeIV(O)]2+ with cyclohexane, the triplet ground state displays a high activation barrier in the hydrogen atom abstraction (HAA) step, while the reaction proceeds via the quintet surface with a much lower barrier.23–25 Nevertheless, a broader analysis is needed in computational studies. Recently, we have prepared a series of linear polydentate nitrogen ligands, and their iron and manganese complexes have demonstrated an excellent performance in the enantioselective oxidation of C
C and C–H bonds.26 Among these ligands, two derived from L-proline, namely Pro3Py (pentadentate ligand, N5) and Pro2PyBn (tetradentate ligand, N4), have similar structures (Scheme 1).27–29 Herein, we report calculations on the hydroxylation of cyclohexane (CYH) by synthetic non-haem FeIVO complexes with both N4/N5 supporting ligands (Pro3Py and Pro2PyBn ligands, Scheme 1). To understand the selectivity between rebound and dissociation, the substrate radical dissociation step after HAA by both FeIVO species is also calculated with DFT.
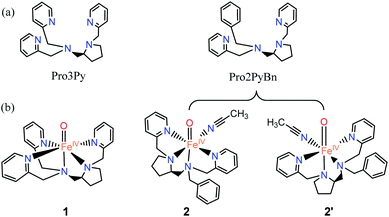 |
| Scheme 1 (a) Pentadentate ligand (N5, Pro3Py) and the tetradentate ligand (N4, Pro2PyBn). (b) FeIVO species: [Pro3PyFeIV(O)]2+ 1, [Pro2PyBnFeIV(O) (CH3CN)]2+ 2 and its isomer 2′. | |
Calculation method
All DFT calculations were performed with the Gaussian 16 suite of quantum chemical packages.30 The spin-unrestricted B3LYP functional31–34 corrected with Grimme's D3 dispersion and Becke–Johnson damping,35,36 UB3LYP-D3(BJ), was used in all calculations. Two basis sets were employed: (i) SDD37 for the Fe atom and 6-31G*38 for remaining atoms. This basis set is denoted as B1 and is used to optimize transition states and minima. (ii) The Def2-TZVPP39 basis set for all atoms, denoted as B2, is used for single-point energy corrections. Transition states were ascertained by vibrational frequency analysis to possess only one imaginary frequency. All optimizations and single-point calculations including solvation were performed using the self-consistent reaction field (SCRF) calculations in the polarizable continuum model (PCM); the experimental solvent acetonitrile (ε = 35.688)40,41 was used. All geometries were fully optimized without symmetry. Frequency calculations were performed to ascertain that minima had no imaginary frequency and transition states had only one imaginary frequency. The spin density iso-surfaces were plotted using the Multiwfn software.42 Energies in the following text were the electronic energies corrected by zero-point vibrational energy (−20 °C) at the UB3LYP-D3(BJ)/B1 level.
Results and discussion
Geometry of 1 and 2
Fig. 1 shows the key geometric parameters and spin-state energy gaps of [Pro3PyFeIV(O)]2+ 1 and [Pro2PyBnFeIV(O) (CH3CN)]2+ 2. In particular, 1 contains two pyridine rings parallel to the Fe–O axis, and the third ring is perpendicular to the Fe–O axis. For the N4 ligand Pro2PyBn, FeIV(O) species 2 contains one pyridine ring parallel to Fe–O, and the incoming solvent acetonitrile acts as a sixth ligand. In addition, FeIV(O) species 2 has an isomer 2′, in which the oxo moiety is trans to the nitrogen of pyrrolidine (Scheme 1b). On the basis of DFT calculations at the UB3LYP-D3(BJ)/B1 level, these FeIV(O) complexes (1, 2 and 2′) have triplet ground states and low-lying quintet excited states, consistent with previous reports. The bond length of Fe–O is 1.627 Å in both 31 and 32, which is approximately 0.2 Å shorter than that of triplet [FeIV(O) (TMC) (CH3CN)]2+ (1.646 Å)3 and 0.1 Å shorter than that of triplet [FeIV(O) (N4Py)]2+ (1.639 Å).23–25,43 The energies of singlet spin states 1 and 2 are very high and thus can be ruled out in the C–H activation reaction.
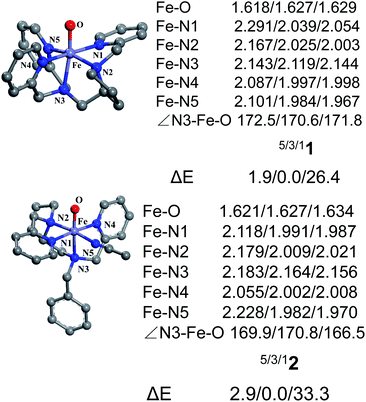 |
| Fig. 1 Key geometric parameters for [Pro3PyFeIV(O)]2+ 1 and [Pro2PyBnFeIV(O) (CH3CN)]2+ 2 at the UB3LYP-D3(BJ)/B1 level and spin-state energy gaps at the UB3LYP-D3(BJ)/B2//B1 level (including ZPE and solvation corrections). | |
H-Atom abstraction reactivity of 1 and 2 with cyclohexane
As shown in Fig. 2, H-atom abstraction is the rate-determining step in cyclohexane hydroxylation by [Pro3PyFeIV(O)]2+ 1, which is in accordance with experimental findings.29 During the Fe–O bond elongation, spin reversion takes place, thus switching the reaction pathway from triplet to quintet spin states in the transition state. For [Pro3PyFeIV(O)]2+ 1, barriers in triplet and quintet states are found to be 14.0 and 7.8 kcal mol−1, respectively, thus making the quintet state more accessible in the HAA process. This clearly indicates that a TSR process is involved in the activation of the C–H bond by 1, following well-established patterns shown by non-haem synthetic FeIVO species.23–25 In the case of 2 [Pro2PyBnFeIV(O) (CH3CN)]2+, the same trends are also observed based on the calculated data (Fig. 3), wherein barriers in the triplet and quintet states are 13.0 and 9.6 kcal mol−1, respectively. As for isomer 2′, barriers are almost the same as 2 (9.4 kcal mol−1, shown in Fig. S4†). In comparison, [Pro3PyFeIV(O)]2+ 1 seems to be more reactive than [Pro2PyBnFeIV(O) (CH3CN)]2+ 2 and 2′. Following the HAA process, IMs (Fig. 2 and 3) from both 1 and 2 rebound to form alcohol complexes without any distinct barriers in both triplet and quintet states.
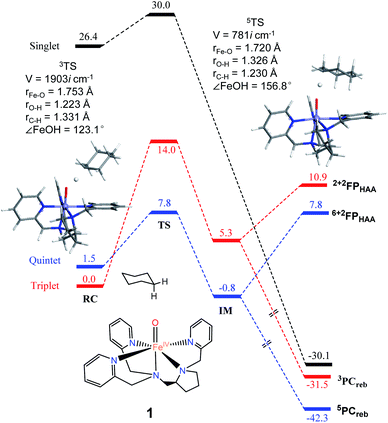 |
| Fig. 2 The key geometric parameters of transition state structures and energy profile for cyclohexane hydroxylation by 1 at the UB3LYP-D3(BJ)/B2//B1 level with solvent correction. RC: reactant cluster, TS: transition state, IM: intermediate, PCreb: rebound production, FP: free production after H-atom abstraction. | |
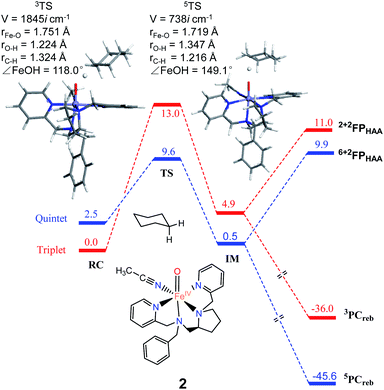 |
| Fig. 3 The key geometric parameters of transition state structures and energy profile for cyclohexane hydroxylation by 2 at the UB3LYP-D3(BJ)/B2//B1 level with solvent correction. RC: Reactant cluster, TS: transition state, IM: intermediate, PCreb: rebound productions, FP: free productions after H-atom abstraction. | |
In addition to the reaction pathway, electronic structures involved in the HAA step are also of great interest. Lower energies on the quintet surface for both FeIVO species 1 and 2 follow well-established patterns shown by previously reported non-haem synthetic FeIVO complexes.23–25 Electron shift diagrams are shown in Scheme 2 for triplet and quintet spin states. In Scheme 2a, the triplet sideway trajectory, a β-spin electron shifts to the π*xz/yz d orbital, producing doublet FeIIIOH coupled to the α-spin electron of the radical substrate (in ϕC). In the quintet upright trajectory, α-spin electron from the substrate shifts to the σ*z2 orbital of the FeIVO moiety, produces a β-spin radical substrate, thereby strengthening stabilizing exchange interactions with other unpaired electrons. This is a type of exchange-enhanced reactivity (EER).11,44 According to the model of orbital overlap at TS (Scheme 2 middle panel), these sideway or upright trajectories are also called π paths or σ paths. Note that TS has the same configuration as IM, and spin natural orbitals and natural orbitals for quintet and triplet TS are shown in Fig. 4 and S2,† respectively. For 3TS, the ϕC orbital contains a small amount of π* of the FeO moiety; the number of electrons in this ϕC is 1.00. However, the occupation of ϕC becomes negative in 5TS, and the ϕC orbital contains a small amount of σ*z2 of the FeO moiety. Moreover, the spin density plot (Fig. 5) also shows that CYH has the opposite spin density in 3TS and 5TS. These pictures directly reflect the difference of electron shift in triplet and quintet H-abstraction process.
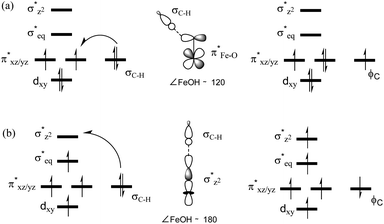 |
| Scheme 2 Electron evolution diagrams during the conversion of (a) 3RC to 3IM and (b) 5RC to 5IM and orbital overlap cartoons in the middle panel. | |
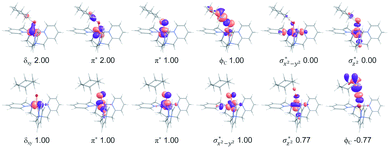 |
| Fig. 4 Natural d orbitals and their occupations in 3TS (top panel) and spin natural orbitals and their occupations in 5TS (bottom panel) for the H-abstraction reaction of 1 with cyclohexane. | |
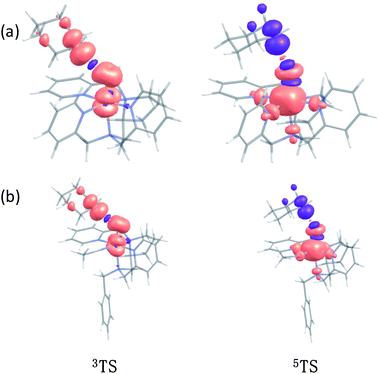 |
| Fig. 5 The spin density plot of (a) 3TS, 5TS for CYH + 1; (b) 3TS, 5TS for CYH + 2 contour value = 0.005. | |
The Mulliken charge of H becomes more positive during H-abstraction, but no distinct changes of Mulliken spin in H is observed (Table S2†), which indicates a proton transformation. Inversely, no changes are found in the Mulliken charge of the CYH radical substrate, and the spin of the CYH radical substrate becomes more positive at the triplet and more negative at the quintet. These data indicate that the H-abstraction and electron shift are concerted proton-coupled electron transfer (PCET) processes. It is consistent with previously reported results.25,45–47
Post H-atom abstraction
In biological enzymes and biomimetic models, the C–H bond activation of alkanes by FeIVO species has been well-documented to give alcohol products via the HAA/oxygen rebound mechanism.48 Beyond the rebound step, instead, the substrate radical may escape from the cage and go through the dissociation mechanism.49,50 The non-rebound pathway has been observed in some non-haem FeIVO complexes, such as [N4PyFeIV(O)]2+ and [(Bn-TPEN)-FeIVO]2+.18,25 Based on experimental and theoretical studies, the dissociation of the substrate radical has been shown to be feasible. In the present study, as shown in Fig. 2, 3 and S4,† energies for radical dissociation are not much higher than the activation energy of H-abstraction, which means that the dissociation mechanism might occur after the HAA step.25
Conclusions
In conclusion, DFT calculations at the UB3LYP-D3(BJ)/Def2-TZVPP//SDD/6-31G* level have been carried out to study the hydroxylation of hexane by two FeIVO complexes bearing a pentadentate ligand (N5, Pro3Py) and a tetradentate ligand (N4, Pro2PyBn) derived from L-proline. Theoretical results have revealed the activation of the C–H bond by FeIVO species 1 (N5) and 2 (N4) via a TSR process, thus making HAA occur in a lower barrier at the quintet spin state. These computational predictions are in agreement with well-documented [N4PyFeIV(O)]2+ species.23–25 On the basis of theoretical data, the dissociation of substrate radicals formed after HAA by both FeIVO species is possible. Additionally, the EER principle predicts the dominance of the quintet spin state during the entire reaction of the C–H hydroxylation of cyclohexane.
Conflicts of interest
There are no conflicts to declare.
Acknowledgements
We acknowledge the financial support of this work from the National Natural Science Foundation of China (21773273, 21802153 and 21902166), the Dalian National Laboratory for Clean Energy (DNL) Cooperation Fund, CAS (Grant no. DNL201904), Key Research Program of Frontier Sciences, CAS (QYZDJ-SSW-SLH051); and Natural Science Foundation of Jiangsu Province (BK20170420).
Notes and references
- M. M. Abu-Omar, A. Loaiza and N. Hontzeas, Chem. Rev., 2005, 105, 2227–2252 CAS.
- L. Que and W. B. Tolman, Nature, 2008, 455, 333–340 CrossRef CAS.
- D. Kumar, B. Karamzadeh, G. N. Sastry and S. P. de Visser, J. Am. Chem. Soc., 2010, 132, 7656–7667 CAS.
- K. Ray, F. F. Pfaff, B. Wang and W. Nam, J. Am. Chem. Soc., 2014, 136, 13942–13958 CrossRef CAS.
- S. Sahu and D. P. Goldberg, J. Am. Chem. Soc., 2016, 138, 11410–11428 CrossRef CAS.
- C. Krebs, D. Galonić Fujimori, C. T. Walsh and J. M. Bollinger, Acc. Chem. Res., 2007, 40, 484–492 CrossRef CAS.
- W. Nam, Y.-M. Lee and S. Fukuzumi, Acc. Chem. Res., 2014, 47, 1146–1154 CrossRef CAS.
- W. Nam, Acc. Chem. Res., 2015, 48, 2415–2423 CrossRef CAS.
- M. Puri and L. Que, Acc. Chem. Res., 2015, 48, 2443–2452 CAS.
- V. A. Larson, B. Battistella, K. Ray, N. Lehnert and W. Nam, Nat. Rev. Chem., 2020, 4, 404–419 CrossRef CAS.
- D. Usharani, D. Janardanan, C. Li and S. Shaik, Acc. Chem. Res., 2013, 46, 471–482 CrossRef CAS.
- D. Schröder, S. Shaik and H. Schwarz, Acc. Chem. Res., 2000, 33, 139–145 CrossRef.
- J. C. Price, E. W. Barr, B. Tirupati, J. M. Bollinger and C. Krebs, Biochemistry, 2003, 42, 7497–7508 CrossRef CAS.
- J.-U. Rohde, J.-H. In, M. H. Lim, W. W. Brennessel, M. R. Bukowski, A. Stubna, E. Münck, W. Nam and L. Que, Science, 2003, 299, 1037 CrossRef CAS.
- X. Engelmann, I. Monte-Pérez and K. Ray, Angew. Chem., Int. Ed., 2016, 55, 7632–7649 CrossRef CAS.
- J. Kaizer, E. J. Klinker, N. Y. Oh, J.-U. Rohde, W. J. Song, A. Stubna, J. Kim, E. Münck, W. Nam and L. Que, J. Am. Chem. Soc., 2004, 126, 472–473 CrossRef CAS.
- J. P. Bigi, W. H. Harman, B. Lassalle-Kaiser, D. M. Robles, T. A. Stich, J. Yano, R. D. Britt and C. J. Chang, J. Am. Chem. Soc., 2012, 134, 1536–1542 CrossRef CAS.
- K.-B. Cho, X. Wu, Y.-M. Lee, Y. H. Kwon, S. Shaik and W. Nam, J. Am. Chem. Soc., 2012, 134, 20222–20225 CrossRef CAS.
- S. Ye, C. Kupper, S. Meyer, E. Andris, R. Navrátil, O. Krahe, B. Mondal, M. Atanasov, E. Bill, J. Roithová, F. Meyer and F. Neese, J. Am. Chem. Soc., 2016, 138, 14312–14325 CrossRef CAS.
- M. S. Seo, N. H. Kim, K.-B. Cho, J. E. So, S. K. Park, M. Clémancey, R. Garcia-Serres, J.-M. Latour, S. Shaik and W. Nam, Chem. Sci., 2011, 2, 1039–1045 RSC.
- A. N. Biswas, M. Puri, K. K. Meier, W. N. Oloo, G. T. Rohde, E. L. Bominaar, E. Münck and L. Que, J. Am. Chem. Soc., 2015, 137, 2428–2431 CrossRef CAS.
- S. H. Bae, M. S. Seo, Y.-M. Lee, K.-B. Cho, W.-S. Kim and W. Nam, Angew. Chem., Int. Ed., 2016, 55, 8027–8031 CrossRef CAS.
- D. Kumar, H. Hirao, L. Que and S. Shaik, J. Am. Chem. Soc., 2005, 127, 8026–8027 CrossRef CAS.
- H. Hirao, D. Kumar, L. Que and S. Shaik, J. Am. Chem. Soc., 2006, 128, 8590–8606 CrossRef CAS.
- D. Janardanan, D. Usharani, H. Chen and S. Shaik, J. Phys. Chem. Lett., 2011, 2, 2610–2617 CrossRef CAS.
- W. Sun and Q. Sun, Acc. Chem. Res., 2019, 52, 2370–2381 CrossRef CAS.
- J. Du, D. Xu, C. Zhang, C. Xia, Y. Wang and W. Sun, Dalton Trans., 2016, 45, 10131–10135 RSC.
- J. Du, C. Miao, C. Xia, Y.-M. Lee, W. Nam and W. Sun, ACS Catal., 2018, 8, 4528–4538 CrossRef CAS.
- J. Du, J. Zhang, J. Zhu, C. Xia and W. Sun, New J. Chem., 2018, 42, 8315–8319 CAS.
- M. J. Frisch, G. W. Trucks, H. B. Schlegel, G. E. Scuseria, M. A. Robb, J. R. Cheeseman, G. Scalmani, V. Barone, G. A. Petersson, H. Nakatsuji, X. Li, M. Caricato, A. V. Marenich, J. Bloino, B. G. Janesko, R. Gomperts, B. Mennucci, H. P. Hratchian, J. V. Ortiz, A. F. Izmaylov, J. L. Sonnenberg, Williams, F. Ding, F. Lipparini, F. Egidi, J. Goings, B. Peng, A. Petrone, T. Henderson, D. Ranasinghe, V. G. Zakrzewski, J. Gao, N. Rega, G. Zheng, W. Liang, M. Hada, M. Ehara, K. Toyota, R. Fukuda, J. Hasegawa, M. Ishida, T. Nakajima, Y. Honda, O. Kitao, H. Nakai, T. Vreven, K. Throssell, J. A. Montgomery Jr, J. E. Peralta, F. Ogliaro, M. J. Bearpark, J. J. Heyd, E. N. Brothers, K. N. Kudin, V. N. Staroverov, T. A. Keith, R. Kobayashi, J. Normand, K. Raghavachari, A. P. Rendell, J. C. Burant, S. S. Iyengar, J. Tomasi, M. Cossi, J. M. Millam, M. Klene, C. Adamo, R. Cammi, J. W. Ochterski, R. L. Martin, K. Morokuma, O. Farkas, J. B. Foresman and D. J. Fox, Gaussian 16, Revision A.03, Gaussian, Inc., Wallingford CT, 2016 Search PubMed.
- A. D. Becke, J. Chem. Phys., 1992, 96, 2155–2160 CrossRef CAS.
- A. D. Becke, J. Chem. Phys., 1992, 97, 9173–9177 CrossRef CAS.
- A. D. Becke, J. Chem. Phys., 1993, 98, 5648–5652 CrossRef CAS.
- C. Lee, W. Yang and R. G. Parr, Phys. Rev. B, 1988, 37, 785–789 CrossRef CAS.
- S. Grimme, J. Antony, S. Ehrlich and H. Krieg, J. Chem. Phys., 2010, 132, 154104 CrossRef.
- S. Grimme, S. Ehrlich and L. Goerigk, J. Comput. Chem., 2011, 32, 1456–1465 CrossRef CAS.
- M. Dolg, U. Wedig, H. Stoll and H. Preuss, J. Chem. Phys., 1987, 86, 866–872 CrossRef CAS.
- R. Ditchfield, W. J. Hehre and J. A. Pople, J. Chem. Phys., 1971, 54, 724–728 CrossRef CAS.
- F. Weigend and R. Ahlrichs, Phys. Chem. Chem. Phys., 2005, 7, 3297–3305 RSC.
- V. Barone and M. Cossi, J. Phys. Chem. A, 1998, 102, 1995–2001 CrossRef CAS.
- M. Cossi, N. Rega, G. Scalmani and V. Barone, J. Comput. Chem., 2003, 24, 669–681 CrossRef CAS.
- T. Lu and F. Chen, J. Comput. Chem., 2012, 33, 580–592 CrossRef CAS.
- E. J. Klinker, J. Kaizer, W. W. Brennessel, N. L. Woodrum, C. J. Cramer and L. Que Jr, Angew. Chem., Int. Ed., 2005, 44, 3690–3694 CrossRef CAS.
- S. Shaik, H. Chen and D. Janardanan, Nat. Chem., 2011, 3, 19–27 CrossRef CAS.
- D. R. Weinberg, C. J. Gagliardi, J. F. Hull, C. F. Murphy, C. A. Kent, B. C. Westlake, A. Paul, D. H. Ess, D. G. McCafferty and T. J. Meyer, Chem. Rev., 2012, 112, 4016–4093 CrossRef CAS.
- J. J. Warren, T. A. Tronic and J. M. Mayer, Chem. Rev., 2010, 110, 6961–7001 CrossRef CAS.
- D. Janardanan, Y. Wang, P. Schyman, L. Que Jr and S. Shaik, Angew. Chem., Int. Ed., 2010, 49, 3342–3345 CrossRef CAS.
- J. T. Groves and G. A. McClusky, J. Am. Chem. Soc., 1976, 98, 859–861 CrossRef CAS.
- K.-B. Cho, H. Hirao, S. Shaik and W. Nam, Chem. Rev., 2016, 45, 1197–1210 CAS.
- M. Maldonado-Domínguez and M. Srnec, J. Am. Chem. Soc., 2020, 142, 3947–3958 CrossRef.
Footnote |
† Electronic supplementary information (ESI) available. See DOI: 10.1039/d0ra08496d |
|
This journal is © The Royal Society of Chemistry 2021 |
Click here to see how this site uses Cookies. View our privacy policy here.