DOI:
10.1039/D1QO00366F
(Research Article)
Org. Chem. Front., 2021,
8, 2970-2976
Corannulene-based nanographene containing helical motifs†
Received
10th March 2021
, Accepted 30th March 2021
First published on 31st March 2021
Abstract
The synthesis and structural analyses of corannulene-PAH hybrids 1 with a [4] helicene subunit and 2 with a [7] helicene subunit have been reported. The structures of 1 and 2 were confirmed by single-crystal X-ray diffraction. The experimental data in combination with theoretical calculations revealed that the terminal ring of helicene and the π-surface of the corannulene unit of 2 adopt a convex conformation, which is a more stable conformation. Meanwhile, 2 displays a red-shift in fluorescence emission but a shorter fluorescence lifetime, in comparison with 1. The optical resolution of 2 was successfully carried out by chiral HPLC, offering enantiopure P-2 and M-2, which were further characterized by CD and CPL spectroscopy.
Introduction
Distorted polycyclic aromatic hydrocarbons (PAHs) or nanographene bearing curved π-conjugated structures have received significant attention due to their unique optical and electronic properties that result from their contorted structures, making them attractive for applications in materials science.1 Corannulene (C20H10), a segment of C60, is regarded as an ideal precursor for developing π-extended distorted PAHs or nanographenes because it has been mass-producible2 and exhibits distinguishing features that involve convex/concave π-surfaces, unusual reactivity, thermodynamic bowl-to-bowl inversion, and electron-accepting ability.3 Thus, tremendous effort has been devoted to discover new features and applications of π-extended distorted PAHs based on the curved structure of corannulene.4 Consequently, a variety of distorted PAHs or nanographenes fused with corannulene have been developed, and they exhibit potential applications not only in semiconductor materials5 as organic field-effect transistors and light-emitting devices, but also in bioimaging6 as potential fluorescent probes. However, there are only limited studies on the chirality of these corannulene-based PAHs or nanographenes.4c,7 For example, Siegel and Baldridge groups disclosed the indenocorannulene chirality owing to the concave/convex π-surface of the corannulene unit.8 Sygula and co-workers developed a corannulene cyclotrimer with three [5] helicene subunits and three corannulene units, which displayed chirality and uncovered the influence of the [5] helicene subunits on the bowl-to-bowl inversion barrier.7a Recently, Scott and co-workers synthesized a corannulene-[6] helicene and revealed the effect of concave/convex π-surfaces on the dynamics of the [6] helicene moiety.4c More recently, we have also reported helical hybrids of corannulene and dibenzocoronene, which exhibit achiral properties due to the formation of meso-double helicenes.9 These findings demonstrate that the incorporation of helical motifs and corannulene into a fused chiral π-system may produce appealing properties,4c,7 such as crystal packing, and photophysical and electronic properties. Thus, we were motivated to develop two corannulene-PAH hybrids 1 and 2 bearing different helical lengths (Fig. 1) as models to shed light on the influence of the helical π-system on their properties. We anticipated that the different lengths of the helical motif would significantly change the molecule behaviour and consequently exert great influence on the properties of distorted nanographenes.
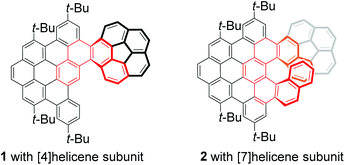 |
| Fig. 1 Molecular structures of 1 and 2. | |
In this work, we report a straightforward bottom-up synthesis of two corannulene–PAH hybrids 1 and 2 with [4] and [7] helicene subunits, respectively (Fig. 1). The structures of 1 and 2 were unambiguously confirmed by X-ray diffraction crystallography and they exhibited different packing motifs in solid phases. The absorption and emission spectra in combination with theoretical calculations revealed the structural diversity and dynamic behaviors. Moreover, owing to the stability of the [7] helicene subunit, two enantiomers of 2 were obtained by chiral HPLC, and their circular dichroism (CD) and circularly polarized luminescence (CPL) spectra were recorded.
Results and discussion
The synthesis of 1 is shown in Scheme 1a. First, cyclopentadienone 39 and ethynylcorannulene 410 undergo the Diels–Alder reaction to afford the intermediate compound 5 in 65% yield. Then, the Scholl reaction of 5 with DDQ as an oxidant resulted in 1 in a good yield of 88% under strongly acidic conditions (MeSO3H) at 0 °C for 1 hour.
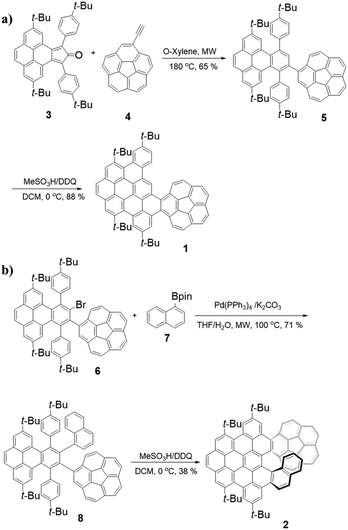 |
| Scheme 1 Synthetic routes of 1 (a) and 2 (b). | |
The synthesis of 2 is shown in Scheme 1b. In the beginning, palladium-catalyzed Suzuki–Miyaura coupling reaction of 6
9 and 1-naphthylboronic ester 7
11 gave a product 8 in 71% yield. Similarly, 8 was further treated with a combination of DDQ and MeSO3H, yielding the desired 2 in 38% yield. The decrease in the yield of 2 can be attributed to the greater steric congestion during the formation of the [7] helicene subunit in comparison with 1 containing a short helical motif. All compounds are characterized by HRMS and NMR spectroscopy (see the ESI†).
The structures of compounds 1, 2 and 5 in solid phases were unequivocally investigated by single-crystal X-ray diffraction (Fig. 2, Fig. S1–3 and Tables S1–3†). As shown in Fig. 2a and c, the crystal structures indicated that the nanographenes 1 and 2 are hybrids of the planar PAH domain and corannulene unit. The PAH domain was slightly twisted due to the repulsion between the PAH domain and the corannulene unit, leading to the formation of [4] helicene and [7] helicene subunits for 1 and 2 (labelled in blue in Fig. 2a and c), respectively. The dihedral angles of the [4] helicene subunit in 1 and the [7] helicene subunit in 2 calculated from the four inner carbon atoms are 37° and 41° (Fig. S10 and 11†), respectively. Meanwhile, for the [7] helicene subunit in 2, the centroid–centroid distance of the overlapping terminal rings is 4.10 Å, which is longer than that of the pristine [7] carbohelicene (3.80 Å),12 presumably due to the convex π-surface of the corannulene unit in 2. In the corannulene unit, the bowl depths of 1 and 2 are 0.87 Å (Fig. S2c and S3c†), which is the same as that of the parent corannulene (0.87 Å).13 This observation indicates that the fusion of PAH and corannulene has no effect on the bowl-depth of the corannulene unit. Importantly, in the case of 1, two nanographenes display a dimer-like stacking of an antiparallel orientation, which leads to a plane-convex π–π interaction between the planar PAH domain and the corannulene unit with a distance of 3.40 Å (Fig. 2b and Fig. S5†). The propagation of such an antiparallel dimer-like stacking along the crystallographic a axis leads to the formation of a double-layer, in which the corannulene units are arranged in a head-to-end layered fashion and display uniform directions. However, the propagation of the double-layer along the crystallographic b axis results in bowl-openings to bowl-opening stackings with a distance of 5.1 Å (Fig. 2b and Fig. S5†). In the case of 2, the nanographenes also adopt a head-to-end layered orientation along the crystallographic c axis. However, the bowl-openings of the corannulene units display an alternate upside-down orientation along the crystallographic c axis (Fig. 2d). Additionally, in the adjacent layers along the crystallographic b axis, two molecules display stackings of antiparallel orientation in which the bowl-openings of the corannulene units show a uniform direction. The average distances (6.6 Å) of the adjacent layers of 2 are the same and completely different from those of 1. Such observations are ascribed to different steric hindrances that originate from the different lengths of the sub-helical units in the nanographenes 1 and 2 (Fig. 2d).
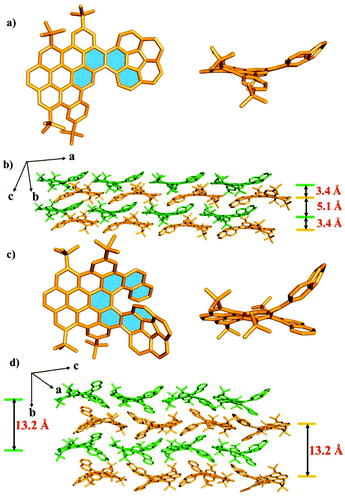 |
| Fig. 2 X-ray crystal structures of the obtained molecules. 1 (a) and 2 (c) from the top and side views. Molecular packing of 1 (b) and 2 (d). Hydrogen atoms are omitted for clarity. | |
Thanks to the unique bowl shape of corannulene, one expects that the terminal ring of helicene would face either the convex or concave π-surface of the bowl if the helical subunit is long enough. In fact, only the convex conformation was observed in the solid phase of 2 as demonstrated by X-ray crystallography (Fig. 2). To further shed light on the dynamic behaviours of 1 and 2, DFT calculations at the B3LYP/6-31G(d) level were performed on both the convex and concave forms of 1 and 2. DFT calculations displayed substantial disparities between the thermodynamic stabilities of the convex and concave conformations (Fig. S19 and 20†). Compared with concave-1 and concave-2, convex-1 and convex-2 are more stable by 3.2 kcal mol−1 (Fig. 3a) and 6.2 kcal mol−1 (Fig. 3b), respectively, clearly demonstrating that the convex form is the more stable conformation. For 1 and 2, these results indicate that the concave conformations are subjected to larger steric congestion than the convex conformations. Furthermore, barriers for bowl-to-bowl inversions from the convex to concave conformation were estimated to be 11.2 (Fig. S19†) and 11.8 (Fig. S20†) kcal mol−1 for 1 and 2, respectively. These values are very close to that for the pristine corannulene (11.5 kcal mol−1),13,14 and these results further confirm the negligible steric influence of the bowl-to-bowl inversions. They also suggest that fast interconversions between the convex and concave conformations can occur in solution but the convex conformations predominate as demonstrated in the solid state. A similar phenomenon has been reported by Scott and co-workers.4c
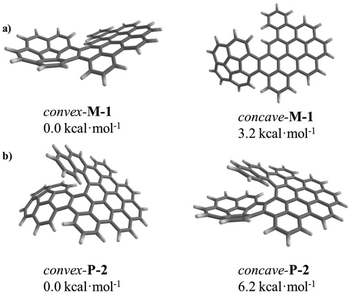 |
| Fig. 3 The terminal rings of the helicene subunits face the convex and concave π-surfaces of the bowl in theory and their relative free energies were calculated by DFT calculations at the B3LYP/6-31G(d) level of the theory in the gas phase. Free energy is thermally corrected and expressed in kcal mol−1. t-Bu groups are omitted for clarity. | |
Next, the photophysical properties of compounds 1 and 2, which were determined by UV-vis absorption and fluorescence emission spectroscopy, are shown in Fig. 4, and the data are summarized in Table 1. As shown in Fig. 4, 1 and 2 display similar absorption and emission contours. For 1, obvious absorption bands were observed in a range of 300–490 nm, with the sharpest absorption peak observed at 350 nm, and two moderate absorption peaks observed at 420 and 442 nm. Notably, the absorption bands of 2 display redshifts, with the sharpest absorption at 398 nm and two shoulder peaks at 452 and 479 nm. These redshifts could be ascribed to the expanded π-conjugation of 2. On the other hand, the emission spectra of 1 and 2 display the maximum peaks located at 487 and 508 nm, respectively. In detail, the emission of 2 shows a redshift of 21 nm, as compared with that of 1. This observation is in line with the absorption result and further confirms the expansion of the conjugated π-system nature in 2. Additionally, the photoluminescence quantum yields (ΦF) of 1 and 2 were determined to be 34% and 22%, respectively. The fluorescence lifetimes (τ) of 1 and 2 were determined to be 12.63 ns and 9.77 ns by single-exponential decay fitting (Fig. S15†), respectively.
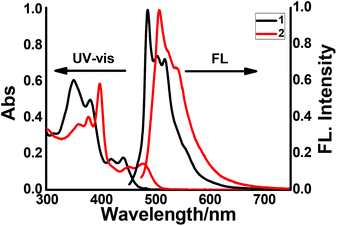 |
| Fig. 4 UV-vis and emission spectra of 1 and 2 were recorded in chloroform solution at room temperature. (All spectra were collected in 1.0 × 10−5 M chloroform.) | |
Table 1 Absorption and emission data for 1 and 2
|
λ
abs
,
[nm] |
E
g(opt)
[eV] |
λ
em
,
[nm] |
Stokes shift [nm] |
λ
abs and fluorescence were measured in chloroform (1.0 × 10−5 M) at room temperature.
Absorption maximum at the longest wavelength.
Estimated from absorption onset, Eg(opt) = 1240/λonest.
Emission maximum (λex = 360 nm for 1 and 408 nm for 2).
|
1
|
350 |
2.53 |
487 |
137 |
2
|
398 |
2.36 |
508 |
110 |
To better investigate the spectroscopic properties of 1 and 2, a time-dependent density functional theory (TD-DFT) study was performed at the B3LYP/6-31G(d)/PCM level based on the crystal structures of 1 and 2. As shown in the top panel of Fig. 5, in the case of 1, the frontier-molecular-orbital result indicates that the LUMO is localized at all molecules, while the LUMO for 2 is much more localized at the PAH domain. However, the HOMO plots of 1 and 2 are strongly delocalized on the PAH domain. Basically, the energy of the HOMO in 2 increased but the energy of the LUMO decreased, as compared with those of 1, leading to a decrease of the HOMO–LUMO gap. Additionally, the calculated HOMO–LUMO gaps for 1 and 2 are 3.04 eV and 2.85 eV, respectively, matching with the experimental values of 2.53 eV for 1 and 2.36 eV for 2. These calculations are in agreement with the experimental bathochromic shift of absorption for 2 with respect to 1.
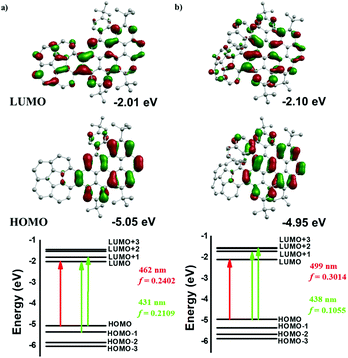 |
| Fig. 5 LUMO (top) and HOMO (middle) energy density and energy diagrams (bottom) of 1 (a) and 2 (b) were calculated by TD-DFT at the B3LYP/6-31G(d)/PCM level. The values of f represent the oscillator strengths. | |
The energy diagrams of the dominant excitation are shown in the bottom panel of Fig. 5. TD-DFT calculations confirm the origin of the spectral shifts of 1 and 2, respectively. In the case of 1, the absorption band at 442 nm is characterized by the pure HOMO → LUMO transition (f = 0.2402, λcalc = 462 nm), and the absorption band at 420 nm is assigned to a large contribution of the HOMO → LUMO+1 transition and a small contribution of the HOMO−1 → LUMO transition (f = 0.2109, λcalc = 431 nm) (Table S4†). For 2, the absorption band at 479 nm is attributed to the pure HOMO → LUMO transition (f = 0.3014, λcalc = 499 nm). On the other hand, the absorption band at 452 nm comes from two degenerate excited states from a large contribution of the HOMO → LUMO+1 transition and a small contribution of the HOMO → LUMO+2 transition (f = 0.1055, λcalc = 438 nm) (Table S5†). Furthermore, the HOMO and LUMO also can be considered as a pair of π and π*-molecular orbitals.4i Therefore, the absorption appearing at 442 nm for 1 and 479 nm for 2 can be attributed to the π–π* transition, which further reflects the expanded π-conjugation in these nanographenes.
Subsequently, on the basis of the crystal structures, the local aromaticity of the individual rings in 1 and 2 was analysed using the average nucleus-independent chemical shift (NICS(1)ZZ)15 (Fig. 6 and Tables S7, 8†). For the corannulene unit, the NICS(1)ZZ values for the central five-membered ring are positive (13.35 for 1 and 13.45 for 2, green numbers), whereas those for all the benzene rings are negative. These values strongly suggested that the five-membered ring possesses substantial antiaromatic characteristics, whereas the benzene rings are all aromatic. For the PAH domains, the central benzene rings have small NICS(1)ZZ values of −1.76 for 1 and −1.61 for 2 (blue numbers), indicating the nonaromaticity of the central benzene rings, similar to our previous observations for corannulene based dibenzocoronene.9 Furthermore, three benzene rings in 1 exhibit medium NICS(1)ZZ values (−5.72, −4.41 and −6.54, red numbers) and four benzene rings in 2 show medium NICS(1)ZZ values (−2.65, −4.20, −3.08 and −3.76, red numbers), revealing that these benzene rings in 1 and 2 show low aromaticity, while other benzene rings have large NICS(1)ZZ values, suggesting the aromaticity of these benzene rings. Moreover, the plots of anisotropy of the induced current density (ACID)16 reveal the clockwise (diamagnetic) ring currents at the peripheries of corannulene and PAH domains (Fig. S21†), which is an indicator of aromaticity. The anticlockwise (paramagnetic) ring currents at the five-membered rings suggested the antiaromaticity. The plots of ACID are in agreement with NICS(1)ZZ calculations.
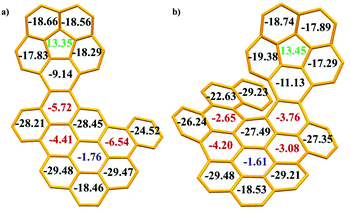 |
| Fig. 6 The average of NICS(1)ZZ values of 1 (a) and 2 (b) were calculated at the GIAO-B3LYP/6-311+G(2d,p)/PCM level based on the crystal structures of 1 and 2. Hydrogen atoms and t-Bu groups are omitted for clarity. | |
The presence of enantiomers in 1 and 2 motivated us to separate their stereoisomers. However, according to DFT calculations, the helix inversion barrier of 1 (4.7 kcal mol−1) (Fig. S19†) is similar to that of pristine [4] helicene (4.1 kcal mol−1),17 and is small enough for inversion at ambient temperature, suggesting that full chiral resolution of 1 would be unfeasible. For 2, the helix inversion barrier should be higher than that of pristine [7] helicene (42.0 kcal mol−1),18 implying that the racemization process is more difficult. So, the chiral resolution of 2 was achieved using an HPLC system equipped with a COSMOSIL Cholester column (eluent
:
DCM/methanol = 70/30, 2.0 mL min−1). Two distinct peaks were detected (Fig. S14†), corresponding to the two enantiomers of 2, and they existed at a ratio of 50
:
50. The CD spectra of the two isolated fractions displayed mirror symmetry, indicating that they are enantiomers with opposite helicity (Fig. 7a). The simulated CD spectra of P-2 and M-2 were in good agreement with the experimental results (Fig. 7a and b). Accordingly, the first peak and the second peak are assigned to P-2 and M-2, respectively (Fig. S14†). Moreover, P-2 and M-2 exhibit circularly polarized luminescence (CPL) activities. The CPL spectra of P-2 and M-2 are mirror images. The value of the luminescence dissymmetry factor (|glum|) was about 4.3 × 10−4 (Fig. 7c), which is typically of an order of 10−5 to 10−3 for small organic molecules.19
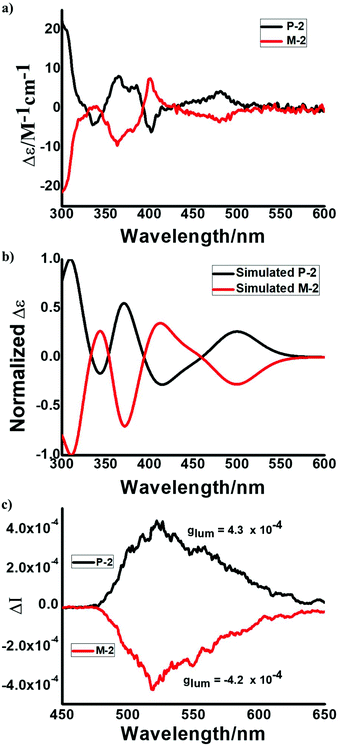 |
| Fig. 7 (a) Experimental CD spectra of P-2 (black solid line) and M-2 (red solid line). (b) Simulated CD spectra of P-2 (black solid line) and M-2 (red solid line) were conducted by TD-DFT at the B3LYP/6-31G(d)/PCM level. (c) CPL spectra of P-2 and M-2. (All spectra were collected at 1.0 × 10−5 M in chloroform.) | |
Conclusions
In conclusion, we have achieved the concise bottom-up synthesis of π-conjugated distorted corannulene-PAH hybrids 1 and 2 containing helical motifs and uncovered their structural features. X-ray crystallographic analyses revealed that 1 and 2 are in a convex conformation and they adopt a head-to-end layered orientation. Particularly, in the case of 1, the antiparallel dimer stacking leads to a double-layer and the corannulene units display uniform directions along the crystallographic a axis, while the propagation of the double-layer reveals face-to-face stackings along the crystallographic b axis. For 2, the corannulene units display an alternate upside-down orientation along the crystallographic c axis and two molecules adopt stackings of antiparallel orientation, in which the bowl-openings of the corannulene units show a uniform direction along the crystallographic b axis. Furthermore, the theoretical calculations showed that convex-1 and convex-2 are more stable by 3.2 kcal mol−1 and 6.2 kcal mol−1, in comparison with concave-1 and concave-2, respectively. The enantiomers of 2 were separated by chiral HPLC, and the chirality of thus obtained two fractions of 2 was identified on the basis of their CD spectra supported by TD-DFT calculations. Furthermore, challenges in terms of synthesizing large π-extended corannulene-based chirality materials and developing their practical optoelectronic applications are the topics of ongoing work in our laboratory.
Conflicts of interest
There are no conflicts to declare.
Acknowledgements
We acknowledge the financial support from the National Natural Science Foundation of China (21971020 and 21672026) and Beijing Natural Science Foundation (2212008).
References
-
(a) Y. T. Wu and J. S. Siegel, Aromatic Molecular-Bowl Hydrocarbons: Synthetic Derivatives, Their Structures, and Physical Properties, Chem. Rev., 2006, 106, 4843–4867 CrossRef CAS PubMed;
(b) V. M. Tsefrikas and L. T. Scott, Geodesic Polyarenes by Flash Vacuum Pyrolysis, Chem. Rev., 2006, 106, 4868–4884 CrossRef CAS PubMed;
(c) A. Narita, X.-Y. Wang, X. Feng and K. Müllen, New advances in nanographene chemistry, Chem. Soc. Rev., 2015, 44, 6616–6643 RSC;
(d) R. Chen, R.-Q. Lu, P.-C. Shi and X.-Y. Cao, Corannulene derivatives for organic electronics: From molecular engineering to applications, Chin. Chem. Lett., 2016, 27, 1175–1183 CrossRef CAS;
(e) J. Shi, Y. Li and Y. Li, Aryne multifunctionalization with benzdiyne and benztriyne equivalents, Chem. Soc. Rev., 2017, 46, 1707–1719 RSC;
(f) M. Rickhaus, M. Mayor and M. Jurá½·ček, Chirality in curved polyaromatic systems, Chem. Soc. Rev., 2017, 46, 1643–1660 RSC;
(g) Q. Xu, C. Wang, D. Zheng, Y. Wang, X. Chen, D. Sun and H. Jiang, A quadruple helicene with a rubicene core: synthesis, structural analyses and properties, Sci. China: Chem., 2021, 64, 590–598 CrossRef CAS.
- A. M. Butterfield, B. Gilomen and J. S. Siegel, Kilogram-Scale Production of Corannulene, Org. Process Res. Dev., 2012, 16, 664–676 CrossRef CAS.
-
(a) T. Hayama, K. K. Baldridge, Y.-T. Wu, A. Linden and J. S. Siegel, Steric Isotope Effects Gauged by the Bowl-Inversion Barrier in Selectively Deuterated Pentaarylcorannulenes, J. Am. Chem. Soc., 2008, 130, 1583–1591 CrossRef CAS PubMed;
(b) C. Bruno, R. Benassi, A. Passalacqua, F. Paolucci, C. Fontanesi, M. Marcaccio, E. A. Jackson and L. T. Scott, Electrochemical and Theoretical Investigation of Corannulene Reduction Processes, J. Phys. Chem. B, 2009, 113, 1954–1962 CrossRef CAS PubMed;
(c) K. Kawasumi, Q. Zhang, Y. Segawa, L. T. Scott and K. Itami, A grossly warped nanographene and the consequences of multiple odd-membered-ring defects, Nat. Chem., 2013, 5, 739–744 CrossRef CAS PubMed;
(d) K. Kato, Y. Segawa, L. C. Scott and K. Itami, Synthesis, Properties, and Packing Structures of Corannulene-Based p-Systems Containing Heptagons, Chem. – Asian J., 2015, 10, 1635–1639 CrossRef CAS PubMed;
(e) V. Rajeshkumar, Y. T. Lee and M. C. Stuparu, Corannulenecarbaldehyde: High-Yielding Synthesis by Rieche Formylation and Facile Access to a Variety of Corannulene Derivatives, Eur. J. Org. Chem., 2016, 36–40 CrossRef CAS;
(f) Z. Lin, C. Li, D. Meng, Y. Li and Z. Wang, Hybrid Corannulene–Perylene Dyes: Facile Synthesis and Optoelectronic Properties, Chem. – Asian J., 2016, 11, 2695–2699 CrossRef CAS PubMed.
-
(a) M. Yanney, F. R. Fronczek and A. Sygula, A 2: 1 Receptor/C60 Complex as a Nanosized Universal Joint, Angew. Chem., Int. Ed., 2015, 54, 11153–11156 CrossRef CAS PubMed;
(b) A. K. Dutta, A. Linden, L. Zoppi, K. K. Baldridge and J. S. Siegel, Extended Corannulenes: Aromatic Bowl/Sheet Hybridization, Angew. Chem., Int. Ed., 2015, 54, 10792–10796 CrossRef CAS;
(c) T. Fujikawa, D. V. Preda, Y. Segawa, K. Itami and L. T. Scott, Corannulene-Helicene Hybrids: Chiral π–Systems Comprising Both Bowl and Helical Motifs, Org. Lett., 2016, 18, 3992–3995 CrossRef CAS;
(d) K. G. U. R. Kumarasinghe, F. R. Fronczek, H. U. Valle and A. Sygula, Bis-corannulenoanthracene: An Angularly Fused Pentacene as a Precursor for Barrelene-Tethered Receptors for Fullerenes, Org. Lett., 2016, 18, 3054–3057 CrossRef CAS PubMed;
(e) Y. Tokimaru, S. Ito and K. Nozaki, Synthesis of Pyrrole-Fused Corannulenes: 1,3-Dipolar Cycloaddition of Azomethine Ylides to Corannulene, Angew. Chem., Int. Ed., 2017, 56, 15560–15564 CrossRef CAS PubMed;
(f) K. Kato, Y. Segawa, L. T. Scott and K. Itami, A Quintuple [6] Helicene with a Corannulene Core as a C 5-Symmetric Propeller-Shaped p-System, Angew. Chem., Int. Ed., 2018, 57, 1337–1341 CrossRef CAS PubMed;
(g) F. Huang, L. Ma, Y. Che, H. Jiang, X. Chen and Y. Wang, Corannulene-Based Coordination Cage with Helical Bias, J. Org. Chem., 2018, 83, 733–739 CrossRef CAS PubMed;
(h) W. Sun, Y. Wang, L. Ma, L. Zheng, W. Fang, X. Chen and H. Jiang, Self-Assembled Carcerand-like Cage with a Thermoregulated Selective Binding Preference for Purification of High-Purity C60 and C70, J. Org. Chem., 2018, 83, 14667–14675 CrossRef CAS PubMed;
(i) J. M. Fernández-García, P. J. Evans, S. M. Rivero, I. Fernández, D. García-Fresnadillo, J. Perles, J. Casado and N. Martín, π–Extended Corannulene-Based Nanographenes: Selective Formation of Negative Curvature, J. Am. Chem. Soc., 2018, 140, 17188–17196 CrossRef PubMed;
(j) W. Sun, L. Ye, J. Liu, L. Zheng, W. Guo, S. Han, C. Shao and H. Jiang, Self-Assembled of Corannulene-Based Molecular Cage with Fullerenes as Template, Chin. J. Org. Chem., 2019, 39, 2867–2874 CrossRef CAS;
(k) E. M. Muzammil, D. Halilovic and M. C. Stuparu, Synthesis of corannulene-based nanographenes, Commun. Chem., 2019, 2, 58–70 CrossRef;
(l) A. K. Rogachev, Y. Zhu, Z. Zhou, S. Liu, Z. Wei and M. A. Petrukhina, Dimerization of indenocorannulene radicals: imposing stability through increasing strain and curvature, Org. Chem. Front., 2020, 7, 3591–3598 RSC;
(m) Q. Xu, C. Wang, D. Zheng, J. He, Y. Wang, X. Chen and H. Jiang, A Distorted Hybrid Corannulene-Dibenzobistetracene, J. Org. Chem., 2021, 86 DOI:10.1021/acs.joc.0c03065.
-
(a) B. M. Schmidt, S. Seki, B. Topolinski, K. Ohkubo, S. Fukuzumi, H. Sakurai and D. Lentz, Electronic Properties of Trifluoromethylated Corannulenes, Angew. Chem., Int. Ed., 2012, 51, 11385–11388 CrossRef CAS;
(b) R.-Q. Lu, W. Xuan, Y.-Q. Zheng, Y.-N. Zhou, X.-Y. Yan, J.-H. Dou, R. Chen, J. Pei, W. Weng and X.-Y. Cao, A corannulene-based donor–acceptor polymer for organic field-effect transistors, RSC Adv., 2014, 4, 56749–56755 RSC;
(c) R.-Q. Lu, Y.-N. Zhou, X.-Y. Yan, K. Shi, Y.-Q. Zheng, M. Luo, X.-C. Wang, J. Pei, H. Xia, L. Zoppi, K. K. Baldridge, J. S. Siegel and X.-Y. Cao, Thiophene-fused bowl-shaped polycyclic aromatics with a dibenzo[a,g]corannulene core for organic field-effect transistors, Chem. Commun., 2015, 51, 1681–1684 RSC;
(d) Y. Wang, Y. Li, W. Zhu, J. Liu, X. Zhang, R. Li, Y. Zhen, H. Dong and W. Hu, Co-crystal engineering: a novel method to obtain one-dimensional (1D) carbon nanocrystals of corannulene–fullerene by a solution process, Nanoscale, 2016, 8, 14920–14924 RSC;
(e) K. Shoyama, D. Schmidt, M. Mahl and F. Würthner, Electron-Poor Bowl-Shaped Polycyclic Aromatic Dicarboximides: Synthesis, Crystal Structures, and Optical and Redox Properties, Org. Lett., 2017, 19, 5328–5331 CrossRef CAS PubMed;
(f) R.-Q. Lu, Y. Liu, S. Wu, M. Saha, H. Qu, R. Chen, L.-L. Yang, X.-Y. Wang, Y. Wang, W. Weng, Y. Zhao and X.-Y. Cao, Impacts of Stereoisomerism on Molecular Packing and Charge Transport of Imide-Fused Corannulene Derivatives, Cryst. Growth Des., 2018, 18, 4240–4244 CrossRef CAS;
(g) D. Meng, G. Liu, C. Xiao, Y. Shi, L. Zhang, L. Jiang, K. K. Baldridge, Y. Li, J. S. Siegel and Z. Wang, Corannurylene Pentapetalae, J. Am. Chem. Soc., 2019, 141, 5402–5408 CrossRef CAS PubMed;
(h) G. Gao, M. Chen, J. Roberts, C. Xiao, G. Zhang, S. Parkin, C. Risko and L. Zhang, Rational Functionalization of a C70 Buckybowl To Enable a C70: Buckybowl Cocrystal for Organic Semiconductor Applications, J. Am. Chem. Soc., 2020, 142, 2460–2470 CrossRef CAS PubMed.
- H.-A. Lin, Y. Sato, Y. Segawa, T. Nishihara, N. Sugimoto, L. T. Scott, T. Higashiyama and K. Itami, A Water-Soluble Warped Nanographene: Synthesis and Applications for Photoinduced Cell Death, Angew. Chem., Int. Ed., 2018, 57, 2874–2878 CrossRef CAS PubMed.
-
(a) M. Yanney, F. R. Fronczek, W. P. Henry, D. J. Beard and A. Sygula, Cyclotrimerization of Corannulyne: Steric Hindrance Tunes the Inversion Barriers of Corannulene Bowls, Eur. J. Org. Chem., 2011, 6636–6639 CrossRef CAS;
(b) V. Rajeshkumar and M. C. Stuparu, A photochemical approach to aromatic extension of the corannulene nucleus, Chem. Commun., 2016, 52, 9957–9960 RSC.
- Y. Wang, O. Allemann, T. S. Balaban, N. Vanthuyne, A. Linden, K. K. Baldridge and J. S. Siegel, Chiral Atropisomeric Indenocorannulene Bowls: Critique of the Cahn–Ingold–Prelog Conception of Molecular Chirality, Angew. Chem., Int. Ed., 2018, 57, 6470–6474 CrossRef CAS PubMed.
- Q. Xu, C. Wang, Y. Zhao, D. Zheng, C. Shao, W. Guo, X. Deng, Y. Wang, X. Chen, J. Zhu and H. Jiang, Tuning the Properties of Corannulene-Based Polycyclic Aromatic Hydrocarbons by Varying the Fusing Positions of Corannulene, Org. Lett., 2020, 22, 7397–7402 CrossRef CAS PubMed.
- J. Mack, P. Vogel, D. Jones, N. Kaval and A. Sutton, The development of corannulene-based blue emitters, Org. Biomol. Chem., 2007, 5, 2448–2452 RSC.
- H.-J. Zhao, Z.-Z. Zhou, Y. Zhang, X. Su, X.-M. Chen and Y.-M. Liang, Visible-light-mediated borylation of aryl and alkyl halides with a palladium complex, Org. Biomol. Chem., 2020, 18, 4390–4394 RSC.
- M. J. Fuchter, J. Schaefer, D. K. Judge, B. Wardzinski, M. Weimar and I. Krossing, [7]-Helicene: a chiral molecular tweezer for silver(I) salts, Dalton Trans., 2012, 41, 8238–8241 RSC.
- J. C. Hanson and C. E. Norman, The crystal and molecular structure of corannulene, C20H10, Acta Crystallogr., Sect. B: Struct. Sci., 1976, 32, 1147–1153 CrossRef.
- L. T. Scott, M. M. Hashemi and M. S. Bratcher, Corannulene Bowl-to-Bowl Inversion Is Rapid at Room Temperature, J. Am. Chem. Soc., 1992, 114, 1920–1921 CrossRef CAS.
-
(a) R. Herges and D. Geuenich, Delocalization of Electrons in Molecules, J. Phys. Chem. A, 2001, 105, 3214–3220 CrossRef CAS;
(b) D. Geuenich, K. Hess, F. Kohler and R. Herges, Anisotropy of the Induced Current Density (ACID), a General Method To Quantify and Visualize Electronic Delocalization, Chem. Rev., 2005, 105, 3758–3772 CrossRef CAS;
(c) J. Z. Dobrowolski and P. F. J. Lipińsk, On splitting of the NICS(1) magnetic aromaticity index, RSC Adv., 2016, 6, 23900–23904 RSC;
(d) M. Antić, B. Furtula and S. Radenković, Aromaticity of Nonplanar Fully Benzenoid Hydrocarbons, J. Phys. Chem. A, 2017, 121, 3616–3626 CrossRef PubMed.
-
(a) E. Steiner, P. W. Fowler and L. W. Jenneskens, Counter-Rotating Ring Currents in Coronene and Corannulene, Angew. Chem., Int. Ed., 2001, 40, 362–366 CrossRef CAS;
(b) Y. Morita, A. Ueda, S. Nishida, K. Fukui, T. Ise, D. Shiomi, K. Sato, T. Takui and K. Nakasuji, Curved Aromaticity of a Corannulene-Based Neutral Radical: Crystal Structure and 3 D Unbalanced Delocalization of Spin, Angew. Chem., Int. Ed., 2008, 47, 2035–2038 CrossRef CAS;
(c) S. N. Spisak, J. Li, A. Y. Rogachev, Z. Wei, O. Papaianina, K. Amsharov, A. V. Rybalchenko, A. A. Goryunkov and M. A. Petrukhina, From Corannulene to Indacenopicene: Effect of Carbon Framework Topology on Aromaticity and Reduction Limits, Organometallics, 2016, 35, 3105–3111 CrossRef CAS;
(d) T. K. Dickens and R. B. Mallion, Topological Ring Currents and Bond Currents in Some Neutral and Anionic Altans and Iterated Altans of Corannulene and Coronene, J. Phys. Chem. A, 2018, 122, 7666–7678 CrossRef CAS.
- K. Kato, Y. Segawa and K. Itami, Symmetric Multiple Carbohelicenes, Synlett, 2019, 30, 370–377 CrossRef CAS.
-
(a) X.-Y. Wang, X.-C. Wang, A. Narita, M. Wagner, X.-Y. Cao, X. Feng and K. Müllen, Synthesis, Structure, and Chiroptical Properties of a Double [7]Heterohelicene, J. Am. Chem. Soc., 2016, 138, 12783–12786 CrossRef CAS PubMed;
(b) K. Kato, Y. Segawa and K. Itami, Symmetric Multiple Carbohelicenes, Synlett, 2019, 30, 370–377 CrossRef CAS.
-
(a) E. M. Sánchez-Carnerero, A. R. Agarrabeitia, F. Moreno, B. L. Maroto, G. Muller, M. J. Ortiz and S. de la Moya, Circularly Polarized Luminescence from Simple Organic Molecules, Chem. – Eur. J., 2015, 21, 13488–13500 CrossRef;
(b) M. Li, W.-B. Lin, L. Fang and C.-F. Chen, Recent Progress on Circularly Polarized Luminescence of Chiral Organic Small Molecules, Acta Chim. Sin., 2017, 75, 1150–1163 CrossRef;
(c) H. Tanaka, Y. Inoue and T. Mori, Circularly Polarized Luminescence and Circular Dichroisms in Small Organic Molecules: Correlation between Excitation and Emission Dissymmetry Factors, ChemPhotoChem, 2018, 2, 386–402 CrossRef CAS;
(d) D. Zheng, L. Zheng, C. Yu, Y. Zhan, Y. Wang and H. Jiang, Significant Enhancement of Circularly Polarized Luminescence Dissymmetry Factors in Quinoline Oligoamide Foldamers with Absolute Helicity, Org. Lett., 2019, 21, 2555–2559 CrossRef;
(e) W.-L. Zhao, M. Li, H.-Y. Lu and C.-F. Chen, Advances in helicene derivatives with circularly polarized luminescence, Chem. Commun., 2019, 55, 13793–13803 RSC;
(f) D. Zheng, C. Yu, L. Zheng, Y. Zhan and H. Jiang, Absolute control of helicity at the C-termini in quinoline oligoamide foldamers by chiral oxazolylaniline moieties, Chin. Chem. Lett., 2020, 31, 673–676 CrossRef CAS;
(g) H. Kubo, T. Hirose, T. Nakashima, T. Kawai, J. Hasegawa and K. Matsuda, Tuning Transition Electric and Magnetic Dipole Moments: [7]Helicenes Showing Intense Circularly Polarized Luminescence, J. Phys. Chem. Lett., 2021, 12, 686–695 CrossRef CAS.
Footnotes |
† Electronic supplementary information (ESI) available. CCDC 1976758, 1976760 and 1976761. For ESI and crystallographic data in CIF or other electronic format see DOI: 10.1039/d1qo00366f |
‡ These authors contributed equally. |
|
This journal is © the Partner Organisations 2021 |