DOI:
10.1039/D1QM00141H
(Research Article)
Mater. Chem. Front., 2021,
5, 3139-3148
DNA nanolantern as biocompatible drug carrier for simple preparation of a porphyrin/G-quadruplex nanocomposite photosensitizer with high photodynamic efficacy†
Received
26th January 2021
, Accepted 12th February 2021
First published on 18th February 2021
Abstract
To overcome the undesirable immune response and adverse side effects, and improve the therapeutic effect, developing a nanocarrier with good biocompatibility and high biosecurity to achieve highly efficient loading of drugs is still urgently needed. For the carriers of photodynamic therapy (PDT) photosensitizers, one of the special requirements is that the photosensitizers are better loaded on the carrier surface. In this paper, we give a paradigm of applying a pure DNA assembly as a biocompatible nanocarrier for highly efficient photosensitizer loading. Via simple DNA hybridization reactions, a pure DNA assembly (termed as a DNA nanolantern) with abundant modifiable sites was prepared and developed as a good nanocarrier for the loading of a porphyrin/G-quadruplex composite photosensitizer on the surface. The as-prepared DNA-based nanocomposite photosensitizer (DNA-NCPS) was characterized by various techniques and demonstrated to hold the charming features of nanoscale size, good monodispersity, robust biostability, strong light absorption in the biological transparent window, high tumor accumulation and cell internalization efficiency, excellent biological safety, low dark toxicity and high phototoxicity, thus showing great promise for PDT applications. Its PDT potential was verified by both in vitro cell and in vivo animal experiments. This study exhibits the great potential of pure DNA nanoassemblies in drug-loading, demonstrating their promising applications in therapy, diagnosis and theranostics.
Introduction
Cancer is one of the main causes of human death. Due to the influence of various factors such as bad living habits and environmental pollution, the risk of human cancer is increasing.1–3 Traditional treatment methods, like chemotherapy and radiotherapy, may induce drug resistance of tumors or damage normal cells, thus causing serious side effects. Moreover, some unique characteristics of tumors, including rapid proliferation, easy metastasis and heterogeneity, also increase the difficulties of treatment.4–8 As a minimally invasive treatment strategy, photodynamic therapy (PDT) has shown great promise in clinical applications. PDT has the advantages of low invasiveness, low toxicity, no drug resistance, targeted therapy and controllability, thus overcoming the disadvantages of traditional treatment methods, such as easy relapse and adverse side effects. Under the combined action of a photosensitizer, oxygen and a laser of appropriate wavelength, cytotoxic reactive oxygen species (ROS), especially singlet oxygen (1O2), are generated through electron transfer or energy transfer pathways, resulting in the ablation of tumor cells.9–14
The therapeutic effect of PDT is related to external conditions such as illumination time, laser intensity and the type of photosensitizer.11 Choosing a suitable photosensitizer is vital for PDT. Because of the excellent optical properties and photostability, porphyrin compounds have been developed as a kind of typical photosensitizer in PDT applications.15–18 However, most porphyrin-based photosensitizers still suffer from the disadvantages of high hydrophobicity, ease of aggregation, short excitation light wavelength and poor target accumulation capability.19–26 To address these, increasing numbers of nanocarriers like metal–organic frameworks (MOFs),27–29 liposomes30,31 and micelles32–34 have been developed for the loading and delivery of photosensitizers.23,24 However, most of the nanocarriers are prepared by non-endogenous substances, which may cause an undesirable immune response and adverse side effects. In addition, due to the limited lifetime and short diffusion radius of 1O2, only the photosensitizers on the nanocarrier surface make a contribution to PDT, since the 1O2 produced by interior photosensitizers is difficult to diffuse out of the nanocarriers. Unfortunately, some nanocarriers have to embed the photosensitizers inside. Therefore, there is an urgent need to develop a nanocarrier with good biocompatibility and high biosecurity to achieve the highly efficient and robust loading of photosensitizers on the surface.
DNA is one of the important biological macromolecules in cells. In recent years, with the rapid development of DNA nanotechnology, a variety of delicately designed and accurately characterized DNA nanoassemblies35–41 (including DNA tetrahedra,42,43 DNA octahedra,44,45 DNA cubes,46,47 DNA nanotubes,48,49 DNA origami,50,51 DNA nanobricks,52,53etc.) have emerged one after another. These pure DNA-based nanoassemblies are highly biocompatible and will not cause undesirable immune response, and thus might be developed as desirable carriers for the loading and delivery of drugs. Herein, using DNA nanolanterns,54 a spherical DNA nanoassembly that is prepared by a simple heating and cooling operation of a mixture of four short-stranded DNAs, as a model carrier, and a water-soluble porphyrin/G-quadruplex complex as a model photosensitizer,55–58 a DNA-based nanocomposite photosensitizer (DNA-NCPS) was prepared, and its size, dispersibility, biostability, light-induced 1O2 generation capability, cell internalization efficiency, cytotoxicity and biocompatibility were characterized. Its PDT application potential was also demonstrated by both in vitro cell and in vivo animal experiments.
Experimental
Preparation of DNA nanolanterns
DNA nanolanterns were prepared by a “one-pot method”. Identical concentrations of four oligonucleotides (A1, A2, P1 and P2, Table S1, ESI†) were mixed in Tris–HCl buffer (10 mM, pH 7.0) containing 25 mM MgCl2. After being mixed thoroughly with a vortex shaker, the mixture was heated at 95 °C for 5 min in a Gene amplifier PCR system and then incubated on ice for 10 min. The as-prepared DNA nanolanterns were used immediately or stored at 4 °C for further use. Polyacrylamide gel electrophoresis (PAGE) analysis was used to verify the successful preparation of DNA nanolanterns. 20 μL of DNA nanolantern solution was mixed with 4 μL of 6 × loading buffer (0.25% bromophenol blue, 0.25% xylene cyanol, 30% glycerol and 10 mM EDTA) and then analysed with 10% polyacrylamide gel in 1 × TAE buffer. The electrophoresis separation was conducted at 120 V for 1 h at room temperature. After being stained with Gel Red stain for 20 min, the gel was photographed by a Gel Image System (Amersham Biosciences).
Preparation of TMPipEOPP/t-KRAS complexes
The cationic porphyrin TMPipEOPP (Scheme S1, ESI†) was designed and prepared by our group.58 The oligonucleotide of t-KRAS (Table S1, ESI†) was obtained from Sangon Biotech. Co., Ltd (Shanghai, China). 2 μM t-KRAS was diluted in 10 mM Tris–HCl buffer (pH 7.0, 10 mM KCl). The obtained solution was heated at 95 °C for 5 min, and then cooled to 25 °C and incubated at this temperature for 30 min. After incubation at 4 °C overnight, 2 μM TMPipEOPP was added and mixed thoroughly. The prepared TMPipEOPP/t-KRAS complex was used immediately or stored at 4 °C for further use.
Preparation of DNA-NCPS
50 μL of DNA nanolanterns (1 μM, single-stranded DNA concentration) and 50 μL of TMPipEOPP/t-KRAS composite photosensitizer (2 μM, TMPipEOPP concentration) were mixed. The mixture was thoroughly mixed and incubated at 37 °C for 1 h. Then, 2 mM Na2EDTA was added and the mixture was further incubated at 37 °C for 1 h to remove excess MgCl2. The mixture was centrifuged for 15 min (14
000 rpm, 4 °C). After being washed three times with ultrapure water, the obtained DNA-NCPS was resuspended in 10 mM Tris–HCl buffer (pH 7.0) for further use.
Biostability evaluation of DNA-NCPS
PAGE and UV-Vis spectroscopy were used to evaluate the biostability of DNA-NCPS. The prepared DNA-NCPS (2 μM for TMPipEOPP concentration) was incubated with 0.4 U mL−1 deoxyribonuclease I (DNase I, final concentration) or 10% (v/v) fetal bovine serum (FBS) at 37 °C for different times. At designated time points, 20 μL of reaction mixture was mixed with 4 μL of 6 × loading buffer for electrophoresis analysis, and 50 μL was used to record the UV-Vis absorption spectrum.
Cell internalization assay of DNA-NCPS
HeLa (human cervical carcinoma) cells were cultured in DMEM medium supplemented with 10% FBS. 0.5 μM TMPipEOPP/t-KRAS complex or DNA-NCPS (TMPipEOPP concentration) was added in the culture medium, and the cells were further incubated for 1, 4, or 8 h. After being washed with PBS buffer three times, the cells were fixed in 4% paraformaldehyde and then imaged by an Olympus IX-81 microscope. 405 and 458 nm were used as the excitation wavelengths to observe the fluorescence of free TMPipEOPP and TMPipEOPP/t-KRAS complex, respectively.
Light-induced 1O2 generation
1,3-Diphenylisobenzofuran (DPBF) was used as an indicator to probe the 1O2 generation in solution. TMPipEOPP/t-KRAS complex or DNA-NCPS (0.5 μM for TMPipEOPP concentration) was mixed with 0.02 mg mL−1 DPBF in the presence of 1% (v/v) Tween-20. The mixture was irradiated with a 650 nm or 690 nm laser, and its absorbance at 417 nm was measured at different time points.
Intracellular 1O2 generation was examined by using 2′,7′-dichlorodihydrofluorescein diacetate (DCFH-DA) as the fluorescent probe of 1O2. HeLa cells were incubated with 0.5 μM DNA-NCPS (or TMPipEOPP/t-KRAS) for 4 h, and then with DCFH-DA (10 μM) for 30 min. After being washed with PBS buffer three times, the cells were subjected to laser (650 or 690 nm) irradiation treatment for 5 min, and the 2′,7′-dichlorofluorescein (DCF) fluorescence in the cells was recorded by an Olympus IX-81 microscope.
Cytotoxicity assay
The phototoxicity and dark toxicity of DNA-NCPS were evaluated using the standard MTT (3-(4,5-dimethylthiazol-2-yl)-2,5-diphenyltetrazolium bromide) assay. As for phototoxicity assay, HeLa cells (5000 cells per well) were seeded in a 96-well microplate. After a 24 h-incubation at 37 °C, 0.5 μM DNA-NCPS or TMPipEOPP/t-KRAS complex was added and allowed to react for another 4 h. Then, the cells were transferred to fresh DMEM medium and subjected to laser (650 or 690 nm) irradiation treatment for 4 min. 24 h later, 5 mg mL−1 MTT solution was added. After a further incubation for 4 h, 100 μL of dimethyl sulfoxide was added and the absorbance (at 490 nm) of each cell sample was measured with a Versamax plate reader. As for the dark toxicity assay, HeLa cells were incubated with different concentrations of DNA-NCPS (or TMPipEOPP/t-KRAS) for different times, and a standard MTT assay was used to measure the cell viability.
In vivo PDT applications
HeLa-tumor-bearing nude mice were employed to evaluate the in vivo antitumor activity of DNA-NCPS. Corresponding experiments were performed with the approval of the Animal Ethics Committee of Tianjin University. 18 mice were divided into three groups, which were respectively administered with saline, TMPipEOPP/t-KRAS or DNA-NCPS (100 μL, 25 μM TMPipEOPP concentration) by tail vein injection. After about 4 h of administration, three mice in each group were subjected to 690 nm laser irradiation (100 mW cm−2) at the tumor sites, and the other three were not. Each mouse was irradiated three times (5 min each time) with 5 min intervals. Every day, the body weight and tumor volume of all mice were measured.
Histological analysis
After PDT treatment, 6 representative mice, which have received different therapies, were sacrificed. Their tumors and major organs were excised, collected and fixed with 4% paraformaldehyde. After being cut into slices, haematoxylin and eosin (H&E) staining was performed on the tumor slices to investigate the tumor pathological changes, and H&E staining organ slices were used to evaluate the biocompatibility of DNA-NCPS.
Results and discussion
Preparation of DNA-NCPS
Our DNA-NCPS was prepared by simply mixing a porphyrin/G-quadruplex composite photosensitizer with pure DNA nanoassemblies termed as DNA nanolanterns (Scheme 1). First, “one-pot” synthesis of the DNA nanolanterns was conducted by mixing four short-stranded DNAs (A1, A2, P1, and P2, Table S1, ESI†), which would alternately hybridize to form nanoscale three-dimensional DNA nanoassemblies with high yield and good reproducibility. To facilitate the modification of the DNA nanolanterns in the next step, P1 and P2 were each designed to contain a “A13CA5CA5” tail at the 5′-end. These tails might be used as modifiable sites of the DNA nanolanterns for subsequent drug loading. Since such tails can be easily added to the 5′- and 3′-ends of the four short-stranded DNAs, abundant modifiable sites will be introduced to the DNA nanolanterns and the number can be arbitrarily adjusted. In addition, these modifiable sites are mainly distributed on the nanolantern surface, thus making subsequent drug loading on the carrier surface possible, which is of great importance for the loading of PDT photosensitizers. This is the reason why we select DNA nanolanterns as the model DNA nanoassembly for PDT photosensitizer loading.
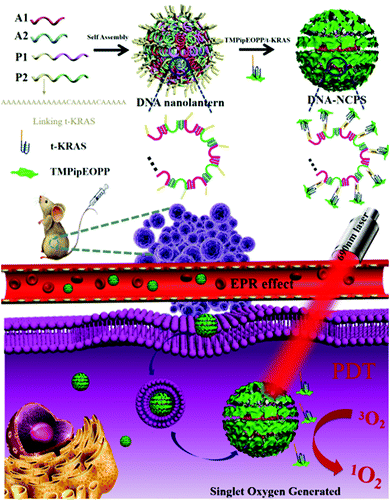 |
| Scheme 1 Preparation of DNA-NCPS and its use in PDT treatment. | |
Porphyrin/G-quadruplex composite photosensitizers are a kind of photosensitizer developed by our group.59 Herein, KRAS, a G-rich oligonucleotide that can fold into a G-quadruplex structure, was used to bind with TMPipEOPP, a water-soluble cationic porphyrin designed and synthesized by our group,58 to form a stable porphyrin/G-quadruplex (TMPipEOPP/KRAS) composite photosensitizer. When a “T5GT5GT13” tail was added to the 5′-end of KRAS, the TMPipEOPP/KRAS complex could be easily assembled on the DNA nanolantern surface via simple DNA hybridization between the “A13CA5CA5” and “T5GT5GT13” tails. as the as-prepared DNA-NCPS might be efficiently delivered to the tumor sites via the enhanced permeability and retention (EPR) effect and perform site-specific tumor PDT treatment under light irradiation in the biological transparent window. Although there are already many DNA-based nanocarriers,60–62 few are composed solely of DNA for photodynamic therapy.63,64 Such pure DNA nanocarriers might have excellent biological safety. In the prepared DNA-NCPS, the TMPipEOPP molecules uniformly distribute on the surface, which can not only reduce the adverse impact of porphyrin aggregation on PDT treatment, but also overcome the limitations of 1O2 lifetime and diffusion distance, thus increasing the availability of the TMPipEOPP/KRAS composite photosensitizer in PDT treatment.
Characterization of DNA-NCPS
Several techniques were used to characterize the successful preparation of DNA-NCPS. First, the successful assembly of the DNA nanolantern was verified by 10% natural polyacrylamide gel electrophoresis (PAGE) analysis. As shown in Fig. 1a, none of A1, A2, P1 and P2 alone gave obvious DNA bands, which is consistent with the poor staining ability of the dye (Gel Red) to single-stranded DNA. A similar result was given by simply mixing the four oligonucleotides. However, when the mixture was heated to 95 °C and then incubated on ice for 10 min, a bright DNA band was observed, suggesting the formation of DNA nanolanterns, whose hydrodynamic diameter was determined to be 89 ± 5 nm by dynamic light scattering (DLS) assay (Fig. 1b). After incubation of the as-prepared DNA nanolanterns with TMPipEOPP/t-KRAS complex at 37 °C for 1 h (t-KRAS is KRAS with a 5′-tail, Table S1, ESI†), the complex could be assembled on the DNA nanolantern surface to give the final product of DNA-NCPS, accompanied by the increase of the DLS hydrodynamic diameter to 108 ± 6 nm. Correspondingly, the Zeta potential was decreased from −4.56 ± 0.8 mV for the TMPipEOPP/t-KRAS complex and −15.1 ± 0.7 mV for the DNA nanolanterns to −19.5 ± 0.8 mV for DNA-NCPS (Fig. 1c). The negatively charged surface can not only assure the monodisperse state of DNA-NCPS, but also prevent protein-induced DNA-NCPS aggregation, thus making it suitable for biomedical applications. Monodisperse DNA-NCPS could be clearly observed from the atomic force microscopy (AFM) image (Fig. 1d and e).
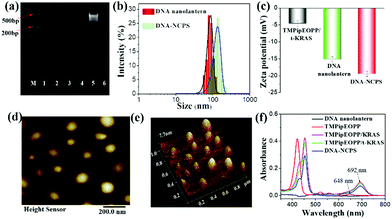 |
| Fig. 1 Characterization of DNA-NCPS preparation by different techniques. (a) PAGE characterization of DNA nanolantern preparation. Lane 1, A1; lane 2, A2; lane 3, P1; lane 4, P2; lane 5, A1 + A2 + P1 + P2 (DNA nanolantern); lane 6, A1 + A2 + P1 + P2 (without heating and cooling operation). (b) DLS characterization of the DNA nanolanterns and DNA-NCPS. (c) Zeta potential characterization of the TMPipEOPP/t-KRAS, DNA nanolanterns and DNA-NCPS. (d) AFM characterization of DNA-NCPS. (e) Three-dimensional AFM image of DNA-NCPS. (f) UV-Vis absorption spectra of the DNA nanolanterns, TMPipEOPP, TMPipEOPP/KRAS, TMPipEOPP/t-KRAS and DNA-NCPS. | |
UV-Vis absorption spectroscopy was further used to verify the formation of a TMPipEOPP/t-KRAS complex and its assembly on DNA nanolanterns. As shown in Fig. 1f, free TMPipEOPP showed a strong Soret absorption band at 421 nm and several relatively weak Q-bands. The Soret band absorption is usually used for porphyrin photosensitizer-based PDT treatment.65–68 To increase the light penetration depth and reduce light toxicity. The Q-band absorption at around 650 nm is also used in some reports.69–73 However, the poor light absorption capability at this wavelength will inevitably weaken the PDT efficiency. Our cationic porphyrin TMPipEOPP (Scheme S1, ESI†) can specifically bind to the DNA G-quadruplex (e.g., KRAS), resulting in the red-shift of the Soret band from 421 nm to 454 nm, accompanied by the emergence of a strong light absorption at around 692 nm. That is, the formation of the TMPipEOPP/G-quadruplex (e.g., TMPipEOPP/KRAS) complex will red-shift the light absorption of free TMPipEOPP from the boundary of the biological transparent window (650–900 nm) to the interior, thus making highly efficient PDT possible. In addition, the greatly increased light absorption will provide the PDT with improved efficiency. Herein, to facilitate the assembly of the TMPipEOPP/KRAS complex on the surface of the DNA nanolanterns, a “T5GT5GT13” tail was added to the 5′-end of KRAS to give a new oligonucleotide of t-KRAS. The addition of such a tail showed little effect on the binding between the G-quadruplex and TMPipEOPP. That is, TMPipEOPP binds with KRAS and t-KRAS with the same binding stoichiometry (1
:
1), comparable binding affinities and similar absorption spectral changes (Fig. S1, ESI†). More importantly, our DNA-NCPS showed an identical absorption spectral characteristic to the TMPipEOPP/t-KRAS complex, thus providing solid evidence for the successful assembly of the TMPipEOPP/t-KRAS complex on the DNA nanolantern surface. By comparing the TMPipEOPP/t-KRAS absorption signals in solution before and after the assembly reaction, the loading efficiency of the TMPipEOPP/t-KRAS complex on the DNA nanolantern surface was calculated to be about 82%.
Biostability of DNA-NCPS and its 1O2 generation capability
A good PDT photosensitizer should be biostable and have high 1O2 generation efficiency under the irradiation of excitation light. DNA is easily attacked by nucleases, which are nearly ubiquitous in biological systems. Therefore, the biostability of DNA-based materials must be evaluated before their biological applications. Deoxyribonuclease I (DNase I) is a representative endonuclease; it is capable of cleaving both single-stranded and double-stranded DNAs. Considering that the DNase I concentration in human serum is about 0.36 ± 0.20 U mL−1, we treated the DNA nanolanterns, TMPipEOPP/t-KRAS complex and DNA-NCPS with 0.4 U mL−1 DNase I to assess their biostability. As shown in Fig. 2a, the DNA nanolantern band in the PAGE image was barely changed after treatment with DNase I for 8 h, indicating the excellent resistance to nucleases of this DNA-based nanocarrier. The TMPipEOPP/t-KRAS complex also showed excellent stability against DNase I. After incubation with 0.4 U mL−1 DNase I for 24 h, the characteristic absorption bands of the TMPipEOPP/t-KRAS complex could still be clearly observed in the UV-Vis spectrum (Fig. 2b), and the light absorption at 692 nm only showed a little decrease. This result is consistent with our previous observation that the formation of the porphyrin/G-quadruplex complex can protect the G-quadruplex from degradation by nucleases.59 After demonstrating the excellent stability of the DNA nanolanterns and TMPipEOPP/t-KRAS complex, the stability of their assembly was then tested. Unsurprisingly, the resultant DNA-NCPS also showed strong anti-nuclease degradation capability, which could be well demonstrated by both electrophoresis and UV-Vis spectral analysis (Fig. 2c and d). Besides nuclease treatment, the excellent biostability of DNA-NCPS was also demonstrated by treatment with 10% (v/v) fetal bovine serum (FBS) (Fig. S2, ESI†). Collectively, DNA-NCPS is highly biostable, it will keep intact in biological systems and retain its strong light absorption capability, thus showing promise for PDT applications.
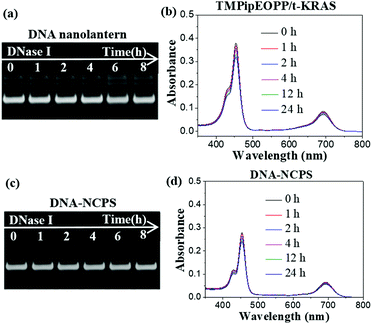 |
| Fig. 2 Biostability of the DNA nanolanterns, TMPipEOPP/t-KRAS complex and DNA-NCPS. (a and c) PAGE images of (a) the DNA nanolanterns and (c) DNA-NCPS after incubation with 0.4 U mL−1 DNase I for different times. (b and d) UV-Vis absorption spectra of (b) the TMPipEOPP/t-KRAS complex and (d) DNA-NCPS after incubation with 0.4 U mL−1 DNase I for different times. | |
Next, the light-induced 1O2 generation capability of DNA-NCPS was tested by recoding the 1O2-dependent hypochromicity of the DPBF (1,3-diphenylisobenzofuran) probe (Fig. 3). Irradiating the DNA-NCPS/DPBF mixture with either a 650 nm or 690 nm laser could result in obvious absorption decrease at 417 nm, the characteristic absorption of DPBF, thus demonstrating the 1O2 generation by DNA-NCPS upon light irradiation. The 1O2 generation efficiency upon 690 nm laser irradiation was much higher than that upon 650 nm laser irradiation, confirming that the use of the TMPipEOPP/G-quadruplex composite photosensitizer red-shifts the optimal excitation light wavelength of PDT treatment from 650 nm (for free TMPipEOPP) to 690 nm (for DNA-NCPS), and thus making highly efficient PDT treatment in the biological transparent window possible.
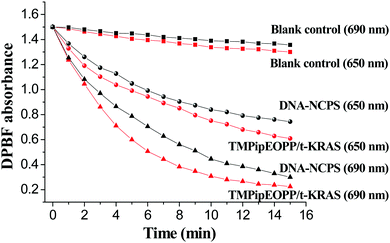 |
| Fig. 3 Time-dependent changes in DPBF absorbance at 417 nm in the presence of DNA-NCPS or TMPipEOPP/t-KRAS complex upon irradiation with a 650 or 690 nm laser. | |
However, the DPBF hypochromicity caused by DNA-NCPS was a little weaker than that caused by the TMPipEOPP/t-KRAS complex, which might be attributed to the different dispersive levels of photosensitizers in solution. When TMPipEOPP/t-KRAS complex is used, both TMPipEOPP/t-KRAS complex and DPBF are highly dispersive in solution. Thus, the 1O2 produced by the TMPipEOPP/t-KRAS complex can rapidly attack the DPBF molecules nearby. In contrast, TMPipEOPP/t-KRAS complexes are highly concentrated on the DNA-NCPS surface. The produced 1O2 has to diffuse from the DNA-NCPS surface to the solution to react with DPBF. Due to the limitation of 1O2 lifetime and diffusion distance, the produced 1O2 might not be fully utilized. Taking this factor into account, the limited difference between DNA-NCPS and TMPipEOPP/t-KRAS-based systems suggests that the TMPipEOPP/t-KRAS complexes are mainly distributed on the surface of DNA-NCPS, which makes them work efficiently in PDT.
Cellular uptake of DNA-NCPS
Highly efficient cell internalization of photosensitizer is another decisive factor of PDT treatment. By recording time-dependent fluorescence change of the TMPipEOPP/t-KRAS complex in HeLa cells, the cell uptake behaviour of DNA-NCPS was investigated and compared with that of the TMPipEOPP/t-KRAS complex. After incubation with the TMPipEOPP/t-KRAS complex for 4 h, only very weak red fluorescence was observed in the confocal laser scanning microscopy (CLSM) image of HeLa cells (Fig. 4), and no significant fluorescence change was observed with the increase of incubation time to 8 h. This result suggested that the TMPipEOPP/t-KRAS complex could not be efficiently internalized by tumor cells, which is consistent with the poor cell penetration of short-stranded DNAs. In contrast, HeLa cells treated with DNA-NCPS showed bright red fluorescence at 4 h. When the incubation time was extended to 8 h, the fluorescence was still strong. It should be noted that the fluorescence excited at 458 nm (the excitation wavelength of the TMPipEOPP/t-KRAS complex, Fig. S3, ESI†) was much brighter than that excited at 405 nm (the excitation wavelength of free TMPipEOPP). This observation suggested that TMPipEOPP keeps in the complex with t-KRAS after being delivered into cells, thus further demonstrating the high biostability of the DNA-NCPS and TMPipEOPP/t-KRAS complex. As a result, 690 nm-excited highly efficient PDT treatment can be assured.
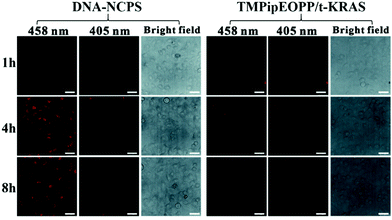 |
| Fig. 4 CLSM images of HeLa cells treated with DNA-NCPS or TMPipEOPP/t-KRAS complex for different times. λex = 458 nm or 405 nm. The concentrations of the two photosensitizers were both 0.5 μM (TMPipEOPP concentration). Scale bars are 25 μm. | |
In vitro PDT performance
After demonstrating that the TMPipEOPP/t-KRAS composite photosensitizer can be efficiently delivered into cancer cells by our DNA-based nanocarrier, we next evaluated the 1O2 generation ability of DNA-NCPS in tumor cells using 2′,7′-dichlorodihydrofluorescein diacetate (DCFH-DA) as the fluorescent indicator of 1O2 (Fig. 5). Both the TMPipEOPP/t-KRAS complex and DNA-NCPS-treated cells showed brighter fluorescence of 2′,7′-dichlorofluorescein (DCF), the oxidation product of DCFH-DA by 1O2, upon 690 nm laser irradiation than upon 650 nm irradiation, demonstrating the higher sensitivity of the photosensitizers to 690 nm excitation light. In addition, under 690 nm laser irradiation, HeLa cells treated with DNA-NCPS emitted much stronger fluorescence than those treated with the TMPipEOPP/t-KRAS complex, which was consistent with the better cell internalization capability of DNA-NCPS than the TMPipEOPP/t-KRAS complex.
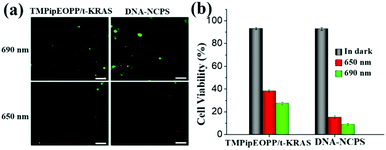 |
| Fig. 5 (a) Light-induced 1O2 generation in HeLa cells treated with the TMPipEOPP/t-KRAS complex or DNA-NCPS. Scale bar is 25 μm. (b) In vitro cytotoxicity of the TMPipEOPP/t-KRAS complex or DNA-NCPS in the dark and upon 650 or 690 nm laser irradiation. The photosensitizer concentrations were all 0.5 μM (TMPipEOPP concentration). | |
In order to further verify the feasibility of DNA-NCPS for PDT applications, the cytotoxicity of the DNA-NCPS and TMPipEOPP/t-KRAS complex was evaluated and compared. Without light irradiation treatment, neither the DNA-NCPS nor TMPipEOPP/t-KRAS complex induced obvious cell death. Even when their concentrations were increased by 5 times (2.5 μM of TMPipEOPP concentration), or the incubation time was extended to 48 h, more than 85% cell viability was maintained (Fig. S4, ESI†), which indicated that our DNA-NCPS has good biological safety and low dark toxicity. In contrast, remarkable phototoxicity was given by both the DNA-NCPS and TMPipEOPP/t-KRAS complex. In addition, under the same conditions, DNA-NCPS showed higher levels of light-induced tumor cell-killing effects than the TMPipEOPP/t-KRAS complex, and a short-duration irradiation (4 min) of DNA-NCPS-treated HeLa cells with a 690 nm laser could significantly reduce the cell viability to 8.9%.
In vivo PDT applications
Encouraged by in vitro cell experiments, we then used tumor-bearing mice to evaluate the PDT performance of DNA-NCPS in vivo. When the tumors grew to about 50–70 mm3, 18 mice were randomly divided into three groups and respectively treated with saline, TMPipEOPP/t-KRAS or DNA-NCPS. Three mice in each group received 690 nm light irradiation treatment and the other three did not. In all mice, only the three that received DNA-NCPS-based PDT treatment gave smaller and smaller tumor volumes (Fig. 6a–c), demonstrating the high PDT efficiency of DNA-NCPS, which not only inhibited the continued growth of the tumor, but also led to the noninvasive ablation of the tumor. In contrast, all other mice gave larger and larger tumors during treatment, including the three mice that received TMPipEOPP/t-KRAS complex-based PDT treatment. The greatly different PDT outcomes of DNA-NCPS and TMPipEOPP/t-KRAS are caused by their different tumor cell uptake efficiencies. DNA-NCPS has better tumor cell internalization efficiency than the TMPipEOPP/t-KRAS complex. Besides this, nanoscale DNA-NCPS can reach the tumor site much more easily than the TMPipEOPP/t-KRAS complex due to the EPR effect.
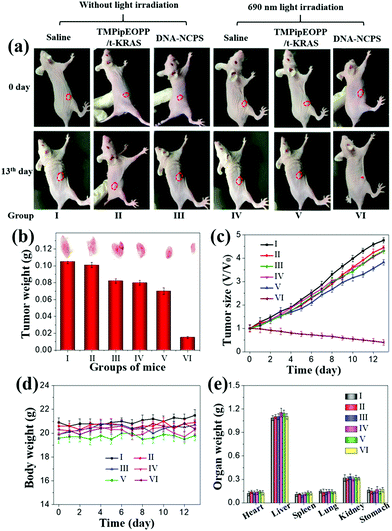 |
| Fig. 6 (a) Representative pre- (0 day) and post-treatment (13th day) photographs of tumor-bearing mice. The tumor sites are circled in red. (b) Photograph of tumors and their weights after different treatments. (c) Tumor volume changes during treatment. V0 and V are the tumor volume before and after treatment. (d) Body weight changes of the mice during treatment. (e) Main organ weights of the mice after treatment. The mice in different groups were treated as labelled in (a). | |
All tested mice showed similar body weight changes and there was no significant difference in their main organs (Fig. 6d–e). From the hematoxylin and eosin (H&E) staining experiment (Fig. 7), it could be found that only the mice that received DNA-NCPS-based PDT treatment showed obvious tumor cell necrosis and tissue fragmentation in their tumor slices, while no detectable cell damage was found in the main organs of all tested mice. All these results further confirm the excellent biocompatibility and powerful PDT potential of DNA-NCPS, thus suggesting that it might be used as a promising PDT photosensitizer for highly efficient PDT with negligible side effects.
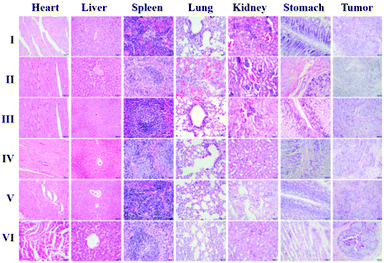 |
| Fig. 7 H&E staining of tumors and main organs of mice after different treatments. The mice in different groups were treated as shown in Fig. 6. | |
Conclusions
In summary, by using a pure DNA assembly as a nanocarrier, a DNA-based nanocomposite photosensitizer was developed via simple DNA hybridization reaction. The as-prepared DNA-NCPS shows great promise in PDT application due to the charming features of nanoscale size, good monodispersity, robust biostability, strong light absorption in the biological transparent window, high tumor accumulation and cell internalization efficiency, excellent biological safety, low dark toxicity and high phototoxicity. Its PDT application potential was well demonstrated by in vitro and in vivo experiments. Herein, we used four short-stranded DNAs to prepare DNA nanolanterns. In fact, DNA nanolanterns might be prepared by different numbers of short-stranded DNAs. In addition, when different numbers of DNA strands are used, DNA nanolanterns with different sizes might be prepared, which will further increase the design flexibility of DNA nanocarriers. Collectively, this study gives a paradigm of applying pure DNA nanoassemblies to construct highly biocompatible drug-delivery systems. With the rapid development of DNA nanotechnology, combined with the unique advantages of DNA in drug-loading applications (e.g., ease of modification, unparalleled programmability, theoretically available aptamers for any target and so on), increasing numbers of pure DNA-based nanoassemblies will be developed for the payload of different drugs (PDT photosensitizers, chemotherapeutic drugs and photothermal reagents), imaging probes, targeted groups and their co-loading, thus showing great promise for therapeutic, diagnostic and theranostic applications.
Conflicts of interest
There are no conflicts to declare.
Acknowledgements
This work was supported by the National Key R&D Program of China (2019YFA0210103), the State Key Laboratory of Medicinal Chemical Biology (2020032) and the National Natural Science Foundation of China [No. 21371130 and 21874075].
Notes and references
- J. Soleymani, M. Hasanzadeh, N. shadjou, M. H. Somi and A. Jouyban, The role of nanomaterials on the cancer cells sensing based on folate receptor: analytical approach, TrAC, Trends Anal. Chem., 2020, 125, 115834 CrossRef CAS.
- H. K. Kordasht and M. Hasanzadeh, Aptamer based recognition of cancer cells: recent progress and challenges in bioanalysis, Talanta, 2020, 220, 121436 CrossRef CAS PubMed.
- W. Sang, Z. Zhang, Y. L. Dai and X. Y. Chen, Recent advances in nanomaterial-based synergistic combination cancer immunotherapy, Chem. Soc. Rev., 2019, 48, 3771–3810 RSC.
- W. Tang, W. P. Fan, J. Lau, L. M. Deng, Z. Y. Shen and X. Y. Chen, Emerging blood-brain-barrier-crossing nanotechnology for brain cancer theranostics, Chem. Soc. Rev., 2019, 48, 2967–3014 RSC.
- W. M. Sharman, J. E. van Lier and C. M. Allen, Targeted photodynamic therapy via receptor mediated delivery systems, Adv. Drug Delivery Rev., 2004, 56, 53–76 CrossRef CAS PubMed.
- A. Juzeniene and J. Moan, The history of PDT in norway part one: identifification of basic mechanisms of general PDT, Photodiagn. Photodyn. Ther., 2007, 4, 3–11 CrossRef CAS PubMed.
- W. P. Fan, B. Yung, P. Huang and X. Y. Chen, Nanotechnology for multimodal synergistic cancer therapy, Chem. Rev., 2017, 117, 13566–13638 CrossRef CAS PubMed.
- J. J. Hu, Q. Lei and X. Z. Zhang, Recent advances in photonanomedicines for enhanced cancer photodynamic therapy, Prog. Mater. Sci., 2020, 114, 100685 CrossRef CAS.
- T. J. Dougherty, C. J. Gomer, B. W. Henderson, G. Jori, D. Kessel, M. Korbelik, J. Moan and Q. Peng, Photodynamic therapy, J. Natl. Cancer Inst., 1988, 90, 889–905 CrossRef PubMed.
- S. S. Lucky, K. C. Soo and Y. Zhang, Nanoparticles in photodynamic therapy, Chem. Rev., 2015, 115, 1990–2042 CrossRef CAS PubMed.
- P. Agostinis, K. Berg, K. A. Cengel, T. H. Foster, A. W. Girotti, S. O. Gollnick, S. M. Hahn, M. R. Hamblin, A. Juzeniene, D. Kessel, M. Korbelik, J. Moan, P. Mroz, D. Nowis, J. Piette, B. C. Wilson and J. Golab, Photodynamic therapy of cancer: an update, Ca-Cancer J. Clin., 2011, 61, 250–281 CrossRef PubMed.
- D. van Straten, V. Mashayekhi, H. S. de Bruijn, S. Oliveira and D. J. Robinson, Oncologic photodynamic therapy: basic principles, current clinical status and future directions, Cancers, 2017, 9, 19 CrossRef PubMed.
- M. S. Baptista, J. Cadet, P. Di Mascio, A. A. Ghogare, A. Greer, M. R. Hamblin, C. Lorente, S. C. Nunez, M. S. Ribeiro, A. H. Thomas, M. Vignoni and T. M. Yoshimura, Type I and type II photosensitized oxidation reactions: guidelines and mechanistic pathways, Photochem. Photobiol., 2017, 93, 912–919 CrossRef CAS PubMed.
- R. R. Allison and K. Moghissi, Photodynamic therapy (PDT): PDT mechanisms, Clin. Endosc., 2013, 46, 24–29 CrossRef PubMed.
- J. P. Celli, B. Q. Spring, I. Rizvi, C. L. Evans, K. S. Samkoe, S. Verma, B. W. Pogue and T. Hasan, Imaging and photodynamic therapy: mechanisms, monitoring, and optimization, Chem. Rev., 2010, 110, 2795–2838 CrossRef CAS PubMed.
- A. E. O’Connor, W. M. Gallagher and A. T. Byrne, Porphyrin and nonporphyrin photosensitizers in oncology: preclinical and clinical advances in photodynamic therapy, Photochem. Photobiol., 2009, 8, 1053–1074 CrossRef PubMed.
- J. Tian, B. X. Huang, M. H. Nawaz and W. A. Zhang, Recent advances of multi-dimensional porphyrin-based functional materials in photodynamic therapy, Coord. Chem. Rev., 2020, 420, 213410 CrossRef CAS.
- M. Ethirajan, Y. Chen, P. Joshi and R. K. Pandey, The role of porphyrin chemistry in tumor imaging and photodynamic therapy, Chem. Soc. Rev., 2011, 40, 340–362 RSC.
- F. Helmich, C. C. Lee, M. M. L. Nieuwenhuizen, J. C. Gielen, P. C. M. Christianen, A. Larsen, G. Fytas, P. E. L. G. Leclère, A. P. H. J. Schenning and E. W. Meijer, Dilution-induced self-assembly of porphyrin aggregates: a consequence of coupled equilibria, Angew. Chem., Int. Ed., 2010, 49, 3939–3942 CrossRef CAS PubMed.
- Q. Zhao, Y. Wang, Y. S. Xu, Y. Yan and J. B. Huang, Out-of-plane coordinated porphyrin nanotubes with enhanced singlet oxygen generation efficiency, Sci. Rep., 2016, 6, 31339 CrossRef CAS PubMed.
- A. L. Wirotius, E. Ibarboure, L. Scarpantonio, M. Schappacher, N. D. McClenaghan and A. Deffieux, Hydrosoluble dendritic poly(ethylene oxide)s with zinc tetraphenylporphyrin branching points as photosensitizers, Polym. Chem., 2013, 4, 1903–1912 RSC.
- C. Escudero, J. Crusats, I. Díez-Pérez, Z. El-Hachemi and J. M. Ribó, Folding and hydrodynamic forces in j-aggregates of 5-phenyl-10,15,20-tris(4-sulfophenyl)porphyrin, Angew. Chem., Int. Ed., 2006, 45, 8032–8035 CrossRef CAS PubMed.
- X. D. Xue, A. Lindstrom and Y. P. Li, Porphyrin-based nanomedicines for cancer treatment, Bioconjugate Chem., 2019, 30, 1585–1603 CrossRef CAS PubMed.
- Y. Zhou, X. Liang and Z. Dai, Porphyrin-loaded nanoparticles for cancer theranostics, Nanoscale, 2016, 8, 12394–12405 RSC.
- S. Hecht, H. Ihre and J. M. J. Frechet, Porphyrin core star polymers: synthesis, modification, and implication for site isolation, J. Am. Chem. Soc., 1999, 121, 9239–9240 CrossRef CAS.
- L. R. H. High, S. J. Holder and H. V. Penfold, Synthesis of star polymers of atyrene and alkyl (meth)acrylates from a porphyrin initiator core via ATRP, Macromolecules, 2007, 40, 7157–7165 CrossRef CAS.
- Z. M. He, X. L. Huang, C. Wang, X. L. Li, Y. J. Liu, Z. J. Zhou, S. Wang, F. W. Zhang, Z. J. Wang, O. Jacobson, J. J. Zhu, G. C. Yu, Y. L. Dai and X. Y. Chen, A catalase-like metal-organic framework nanohybrid for O2-evolving synergistic chemoradiotherapy, Angew. Chem., Int. Ed., 2019, 58, 8752–8756 CrossRef CAS PubMed.
- Y. B. Wang, W. B. Wu, J. J. Liu, P. N. Manghnani, F. Hu, D. Ma, C. Teh, B. Wang and B. Liu, Cancer-cell-activated photodynamic therapy assisted by Cu(II)-based metal-organic framework, ACS Nano, 2019, 13, 6879–6890 CrossRef CAS PubMed.
- G. X. Lan, K. Y. Ni, Z. Xu, S. S. Veroneau, Y. Song and W. B. Lin, Nanoscale metal-organic framework overcomes hypoxia for photodynamic therapy primed cancer immunotherapy, J. Am. Chem. Soc., 2018, 140, 5670–5673 CrossRef CAS PubMed.
- A. K. Rengan, A. B. Bukhari, A. Pradhan, R. Malhotra, R. Banerjee, R. Srivastava and A. De,
In vivo analysis of biodegradable liposome gold nanoparticles as efficient agents for photothermal therapy of cancer, Nano Lett., 2015, 15, 842–848 CrossRef CAS.
- M. Amiri, T. Gholami, O. Amiri, A. Pardakhti, M. Ahmadi, A. Akbari, A. Amanatfard and M. SalavatiNiasari, The magnetic inorganic-organic nanocomposite based on ZnFe2O4-imatinib-liposome for biomedical applications, in vivo and in vitro study, J. Alloys Compd., 2020, 849, 156604 CrossRef CAS.
- S. Y. Li, C. Li, S. B. Jin, J. Liu, X. D. Xue, A. S. Eltahan, J. D. Sun, J. J. Tan, J. C. Dong and X. J. Liang, Overcoming resistance to cisplatin by inhibition of glutathione s-transferases (GSTs) with ethacraplatin micelles in vitro and in vivo, Biomaterials, 2017, 144, 119–129 CrossRef CAS PubMed.
- J. Z. Zhu, T. T. Xiao, J. L. Zhang, H. L. Che, Y. X. Shi, X. Y. Shi and J. C. M. van Hest, Surface-charge-switchable nanoclusters for magnetic resonance imaging-guided and glutathione depletion-enhanced photodynamic therapy, ACS Nano, 2020, 14, 11225–11237 CrossRef CAS PubMed.
- S. Zhong, C. Chen, G. L. Yang, Y. C. Zhu, H. L. Cao, B. J. Xu, Y. Q. Luo, Y. Gao and W. A. Zhang, Acid-triggered nanoexpansion polymeric micelles for enhanced photodynamic therapy, ACS Appl. Mater. Interfaces, 2019, 11, 33697–33705 CrossRef CAS PubMed.
- Y. He, T. Ye, M. Su, C. Zhang, A. E. Ribbe, W. Jiang and C. D. Mao, Hierarchical self-assembly of DNA into symmetric supramolecular polyhedr, Nature, 2008, 452, 198–201 CrossRef CAS PubMed.
- S. M. Douglas, H. Dietz, T. Liedl, B. Högberg, F. Graf and W. M. Shih, Self-assembly of DNA into nanoscale three-dimensional shapes, Nature, 2009, 459, 414–418 CrossRef CAS.
- Z. Z. Yang, X. Peng, P. Yang, Y. Zhuo, Y. Q. Chai, W. B. Liang and R. Yuan, A janus 3D DNA nanomachine for simultaneous and sensitive fluorescence detection and imaging of dual microRNAs in cancer cells, Chem. Sci., 2020, 11, 8482–8488 RSC.
- A. S. Walsh, H. F. Yin, C. M. Erben, M. J. A. Wood and A. J. Turberfield, DNA cage delivery to mammalian cells, ACS Nano, 2011, 5, 5427–5432 CrossRef CAS PubMed.
- J. Wang, D. X. Wang, J. Y. Ma, Y. X. Wang and D. M. Kong, Three-dimensional DNA nanostructures to improve the hyperbranched hybridization chain reaction, Chem. Sci., 2019, 10, 9758–9767 RSC.
- G. Y. Sun, Y. C. Du, Y. X. Cui, J. Wang, X. Y. Li, A. N. Tang and D. M. Kong, Terminal deoxynucleotidyl transferase-catalyzed preparation of pH-responsive DNA nanocarriers for tumor-targeted drug delivery and therapy, ACS Appl. Mater. Interfaces, 2019, 11, 14684–14692 CrossRef CAS PubMed.
- R. P. Goodman, R. M. Berry and A. J. Turberfield, The single-step synthesis of a DNA tetrahedron, Chem. Commun., 2004, 1372–1373 RSC.
- J. Wang, D. X. Wang, A. N. Tang and D. M. Kong, Highly integrated, biostable, and self-powered DNA motor enabling autonomous operation in living bodies, Anal. Chem., 2019, 91, 5244–5251 CrossRef CAS PubMed.
- Y. G. Ke, J. Sharma, M. H. Liu, K. Jahn, Y. Liu and H. Yan, Scaffolded DNA origami of a DNA tetrahedron molecular container, Nano Lett., 2009, 9, 2445–2447 CrossRef CAS PubMed.
- Y. He, M. Su, P. A. Fang, C. Zhang, A. E. Ribbe, W. Jiang and C. D. Mao, On the chirality of self-assembled DNA octahedra, Angew. Chem., Int. Ed., 2010, 49, 748–751 CrossRef CAS PubMed.
- L. Zhong, S. Cai, Y. Huang, L. Yin, Y. Yang, C. Lu and H. Yang, DNA octahedron-based fluorescence nanoprobe for dual tumor-related mRNAs detection and imaging, Anal. Chem., 2018, 90, 12059–12066 CrossRef CAS PubMed.
- M. B. Scheible, L. L. Ong, J. B. Woehrstein, R. Jungmann, P. Yin and F. C. Simmel, A compact DNA cube with side length 10 nm, Small, 2015, 11, 5200–5205 CrossRef CAS.
- T. G. W. Edwardson, K. M. M. Carneiro, C. K. McLaughlin, C. J. Serpell and H. F. Sleiman, Site-specific positioning of dendritic alkyl chains on DNA cages enables their geometry-dependent self-assembly, Nat. Chem., 2013, 5, 868–875 CrossRef CAS PubMed.
- F. Xu, T. Wu, X. Shi and L. Pan, A study on a special DNA nanotube assembled from two single-stranded tiles, Nanotechnology, 2019, 30, 115602 CrossRef CAS PubMed.
- S. Agarwal and E. Franco, Enzyme-driven assembly and disassembly of hybrid DNA–RNA nanotubes, J. Am. Chem. Soc., 2019, 141, 7831–7841 CrossRef CAS PubMed.
- B. Saccà and C. M. Niemeyer, DNA origami: the art of folding DNA, Angew. Chem., Int. Ed., 2012, 51, 58–66 CrossRef PubMed.
- Y. X. Zhao, A. Shaw, X. H. Zeng, E. Benson, A. M. Nystrom and B. Hogberg, DNA origami delivery system for cancer therapy with tunable release properties, ACS Nano, 2012, 6, 8684–8691 CrossRef CAS PubMed.
- T. Torring, N. V. Voigt, J. Nangreave, H. Yan and K. V. Gothelf, DNA origami: a quantum leap for self-assembly of complex structures, Chem. Soc. Rev., 2011, 40, 5636–5646 RSC.
- B. Q. Ding, H. Wu, W. Xu, Z. A. Zhao, Y. Liu, H. B. Yu and H. Yan, Interconnecting gold Iislands with DNA origami nanotubes, Nano Lett., 2010, 10, 5065–5069 CrossRef CAS.
- D. X. Wang, J. Wang, Y. X. Cui, Y. X. Wang, A. N. Tang and D. M. Kong, Nanolantern-based DNA probe and signal amplifier for tumor-related biomarker detection in living cells, Anal. Chem., 2019, 91, 13165–13173 CrossRef CAS PubMed.
- B. Wu, D. M. Kong and L. N. Zhu, Specific recognition and stabilization of monomeric and multimeric g-quadruplexes by cationic porphyrin TMPipEOPP under molecular crowding conditions, Nucleic Acids Res., 2013, 41, 4324–4335 CrossRef PubMed.
- X. X. Huang, L. N. Zhu, B. Wu, Y. F. Huo, N. N. Duan and D. M. Kong, Two cationic porphyrin isomers showing different multimeric g-quadruplex recognition specificity against monomeric g-quadruplexes, Nucleic Acids Res., 2014, 42, 8719–8731 CrossRef CAS PubMed.
- L. N. Zhang, R. Zhang, Y. X. Cui, K. K. Liu, D. M. Kong, X. Z. Li and L. N. Zhu, Highly specific g-quadruplex recognition covering physiological pH range by a new water-soluble cationic porphyrin with low self-aggregation tendency, Dyes Pigm., 2017, 145, 404–417 CrossRef CAS.
- L. N. Zhu, S. J. Zhao, B. Wu, X. Z. Li and D. M. Kong, A new cationic porphyrin derivative (TMPipEOPP) with large side arm substituents: a highly selective g-quadruplex optical probe, PLoS One, 2012, 7, e35586 CrossRef CAS PubMed.
- M. Cheng, Y. X. Cui, J. Wang, J. Zhang, L. N. Zhu and D. M. Kong, G-quadruplex/porphyrin composite photosensitizer: a facile way to promote absorption redshift and photodynamic therapy efficacy, ACS Appl. Mater. Interfaces, 2019, 11, 13158–13167 CrossRef CAS PubMed.
- Y. X. Cui, G. Y. Sun, Y. H. Li, A. N. Tang, L. N. Zhu and D. M. Kong, DNA-based pH-responsive core-shell drug nanocarrier for tumor-targeted chemo-photodynamic therapy, Adv. Mater. Interfaces, 2020, 7, 2000292 CrossRef CAS.
- W. Y. Ye, X. H. Chen, X. Li, Y. M. Liu, F. Jia, Q. Jin and J. Ji, Structure-switchable DNA programmed disassembly of nanoparticles for smart size tunability and cancer-specific drug release, ACS Appl. Mater. Interfaces, 2020, 12, 22560–22571 CrossRef CAS PubMed.
- Y. Yang, W. J. Zhu, L. Z. Feng, Y. Chao, X. Yi, Z. L. Dong, K. Yang, W. H. Tan, Z. Liu and M. W. Chen, G-quadruplex-based nanoscale coordination polymers to modulate tumor hypoxia and achieve nuclear-targeted drug delivery for enhanced photodynamic therapy, Nano Lett., 2018, 18, 6867–6875 CrossRef CAS.
- Q. S. Chen, C. Y. Li, X. H. Yang, J. Huang, S. Y. Liu, W. Liu, J. B. Liu and K. M. Wang, Self-assembled DNA nanowires as quantitative dual-drug nanocarriers for antitumor chemophotodynamic combination therapy, J. Mater. Chem. B, 2017, 5, 7529–7537 RSC.
- X. X. Zhuang, X. W. Ma, X. D. Xue, Q. Jiang, L. L. Song, L. R. Dai, C. Q. Zhang, S. B. Jin, K. N. Yang, B. Q. Ding, P. C. Wang and X. J. Liang, A photosensitizer-loaded DNA origami nanosystem for photodynamic therapy, ACS Nano, 2016, 3, 3486–3495 CrossRef PubMed.
- A. Naik, R. Rubbiani, G. Gasser and B. Spingler, Visible-light-induced annihilation of tumor cells with platinum-porphyrin conjugates, Angew. Chem., Int. Ed., 2014, 53, 6938–6941 CrossRef CAS PubMed.
- J. Park, Q. Jiang, D. W. Feng and H. C. Zhou, Controlled generation of singlet oxygen in living cells with tunable ratios of the photochromic switch in metal-organic frameworks, Angew. Chem., Int. Ed., 2016, 55, 7188–7193 CrossRef CAS PubMed.
- Y. Qin, L. J. Chen, F. Y. Dong, S. T. Jiang, G. Q. Yin, X. P. Li, Y. Tian and H. B. Yang, Light-controlled generation of singlet oxygen within a discrete dual-sage metallacycle for cancer therapy, J. Am. Chem. Soc., 2019, 141, 8943–8950 CrossRef CAS PubMed.
- Y. A. Shieh, S. J. Yang, M. F. Wei and M. J. Shieh, Aptamer-based tumor-targeted drug delivery for photodynamic therapy, ACS Nano, 2010, 4, 1433–1442 CrossRef CAS.
- B. X. Huang, P. Wang, Y. J. Ouyang, R. Q. Pang, S. Y. Liu, C. Y. Hong, S. H. Ma, Y. Gao, J. Tian and W. A. Zhang, Pillar[5]arene-based switched supramolecular photosensitizer for self-amplifified and pH-activated photodynamic therapy, ACS Appl. Mater. Interfaces, 2020, 12, 41038–41046 CrossRef.
- N. Zheng, D. Xie, C. S. Wang, Z. Y. Zhang, Y. B. Zheng, Q. Lu, Y. G. Bai, Y. Li, A. G. Wang and W. Z. Song, Water-soluble, zwitterionic poly-photosensitizers as carrier-free, photosensitizer-self-delivery system for in vivo photodynamic therapy, ACS Appl. Mater. Interfaces, 2019, 11, 44007–44017 CrossRef CAS PubMed.
- B. B. Sun, R. Chang, S. P. Cao, C. Q. Yuan, L. Y. Zhao, H. W. Yang, J. B. Li, X. H. Yan and J. C. M. van Hest, Acid-activatable transmorphic peptide-based nanomaterials for photodynamic therapy, Angew. Chem., Int. Ed., 2020, 59, 20582–20588 CrossRef CAS.
- P. F. Zhang, J. Q. Wang, H. Chen, L. Zhao, B. B. Chen, C. C. Chu, H. Liu, Z. N. Qin, J. Y. Liu, Y. Z. Tan, X. Y. Chen and G. Liu, Tumor microenvironment-responsive ultrasmall nanodrug generators with enhanced tumor delivery and penetration, J. Am. Chem. Soc., 2018, 140, 14980–14989 CrossRef PubMed.
- J. F. Wang, Y. Zhong, X. Wang, W. T. Yang, F. Bai, B. B. Zhang, L. Alarid, K. F. Bian and H. Y. Fan, pH-dependent assembly of porphyrin-silica nanocomposites and their application in targeted photodynamic therapy, Nano Lett., 2017, 17, 6916–6921 CrossRef CAS PubMed.
Footnotes |
† Electronic supplementary information (ESI) available: Materials and instruments, oligonucleotides used in this work, chemical structure of TMPipEOPP, UV-Vis spectra, excitation and emission spectra and the dark cytotoxicities of the TMPipEOPP/t-KRAS complex and DNA-NCPS. See DOI: 10.1039/d1qm00141h |
‡ Yonghui Li and Junqing Chu contributed equally to this work. |
|
This journal is © the Partner Organisations 2021 |