DOI:
10.1039/D0QM00974A
(Research Article)
Mater. Chem. Front., 2021,
5, 1418-1427
Supramolecular nanoparticles constructed from pillar[5]arene-based host–guest complexation with enhanced aggregation-induced emission for imaging-guided drug delivery†
Received
23rd November 2020
, Accepted 4th December 2020
First published on 9th December 2020
Abstract
Fluorophores with aggregation-induced emission (AIE) characteristics have attracted more and more attention due to their unparalleled advantages in terms of sensitivity and photostability, and have been extensively utilized for disease diagnosis and therapy. However, it remains challenging to restrict the intramolecular rotation of the AIE luminogens (AIEgens) both in solution and aggregated states. Herein, we utilize host–guest chemistry to achieve this meaningful goal by using a carboxylate-modified pillar[5]arene H as a supramolecular host and an AIEgen containing a tetraphenylethene core G as a guest. The AIE effect of the fluorophore is effectively enhanced upon the formation of an inclusion host–guest complex H⊃G, and the fluorescence of H⊃G is much more intensive than that of free G. Supramolecular nanoparticles (SNPs) with high emission are prepared from H⊃G using a nanoprecipitation method, which can be used as a fluorescent probe for living cell imaging showing superior photostability against laser excitation. Intriguingly, the formed SNPs act as nanocarriers to encapsulate doxorubicin (DOX) to deactivate both the fluorescence of SNPs and DOX caused by the energy transfer relay (ETR) effect, which is mediated by Förster resonance energy transfer and aggregation-caused quenching. The release of loaded DOX after cellular internalization interrupts the ETR effect to light up the silenced fluorescence, thus allowing in situ visualization of drug release. More importantly, the anticancer efficacy of the loaded drug is greatly maintained using this sophisticated supramolecular system, showing promising potential in imaging-guided drug delivery.
Introduction
Fluorophores, such as cyanine, 2,1,3-benzothiadiazole, rhodamine, fluorescein and boron-dipyrromethene dyes with bright emission have been demonstrated as promising fluorescent probes and even therapeutic agents for biological and biomedical applications.1–8 Typically, these fluorophores work in very dilute states due to the aggregation-caused quenching (ACQ) effect whose emissions are significantly quenched at high concentration or in the aggregate state, arising from the formation of excimers and exciplexes in the condensed phase mainly triggered by the intermolecular π–π stacking and hydrophobic interactions.9–13 As a consequence, their fluorescence signals are easily photobleached when harsh laser beams are applied as the excitation light sources, which greatly undermines their sensitivity and poses formidable hurdles for further utilization.14–20 In sharp comparison to the ACQ effect, novel organic luminogens with an extraordinary aggregation-induced emission (AIE) behavior have been developed and attracted wide attention over the past few years.21–27 With tetraphenylethene (TPE) and hexaphenylsilole being typical examples, these AIE luminogens (AIEgens) are poorly emissive in solution while become intensely fluorescent on molecular aggregation.28–33 The mechanism behind this phenomenon is involved in the restriction of intramolecular rotation (RIR) of the aromatic rings, which is exactly opposite to the ACQ systems.34–40 More importantly, the AIEgen-based fluorophores exhibit unique advantages in photostability that are more resistant to laser irradiations.
For some special biomedical and theranostic applications requiring fluorophores with high sensitivity and contrast, it is extremely important to fabricate new AIEgens whose fluorescence brightly emits even in dilute solution. We anticipated that encapsulating AIE-type chromophores within a rigid cavity would provide a sophisticated strategy to effectively restrict the phenyl rotation at the molecular level, thus achieving the AIE effect. Macrocycles including cyclodextrins, cucurbiturils, calixarenes and pillararenes are brilliant supramolecular hosts with the ability to wrap guest molecules in their inner cavity upon the formation of inclusion complexes,41–49 providing feasible platforms to reduce the intramolecular rotation of the AIEgens by taking advantage of non-covalent interactions. Benefiting from their highly symmetrical and rigid pillar architectures, versatile functionalizations, and fantastic host–guest recognitions, pillar[n]arenes have attracted more and more attention from scientists since their discovery.50–54 Fruitful supramolecular systems, including supramolecular polymers, sensors, liquid crystals, molecular machines, drug delivery systems, metal–organic frameworks and theranostic agents, have been successfully constructed by exploiting their outstanding properties.55–61 However, the fabrications of AIE-based nanomaterials with multiple functions exploiting the pillar[n]arenes-based platforms is scant.
Herein, we prepare supramolecular nanoparticles with enhanced AIE effect by employing pillar[5]arene-based host–guest complexation and further utilize them as fluorescent nanocarriers for imaging-guided drug delivery (Scheme 1). A pillar[5]arene modified by one carboxylate group (H) is used as a supramolecular host and a TPE-cored chromophore containing a pyridine (G) as a supramolecular guest. The fluorescence intensity of G significantly bursts by restricting the intramolecular rotation of G upon the formation of the inclusion complex H⊃G mainly driven by the proton transfer-assisted electrostatic interactions. Through a nanoprecipitation method, supramolecular nanoparticles (SNPs) possessing bright emission are obtained, of which photostability is remarkably high. More interestingly, a dual-fluorescence-quenched ternary system SNPs@DOX is constructed by loading an anticancer drug doxorubicin (DOX) into the SNPs caused by the energy transfer relay (ETR) effect, which is mediated by Förster resonance energy transfer (FRET) from the SNPs to DOX followed by the ACQ effect. Triggered by low intracellular pH, the loaded DOX molecules effectively release from the SNPs@DOX, and thus the silenced fluorescence is fully recovered by interrupting the ETR effect. Benefiting from the AIE effect and non-covalent interactions, this supramolecular system will possibly be applied in imaging-guided drug delivery, allowing visualization of the drug release in real-time.
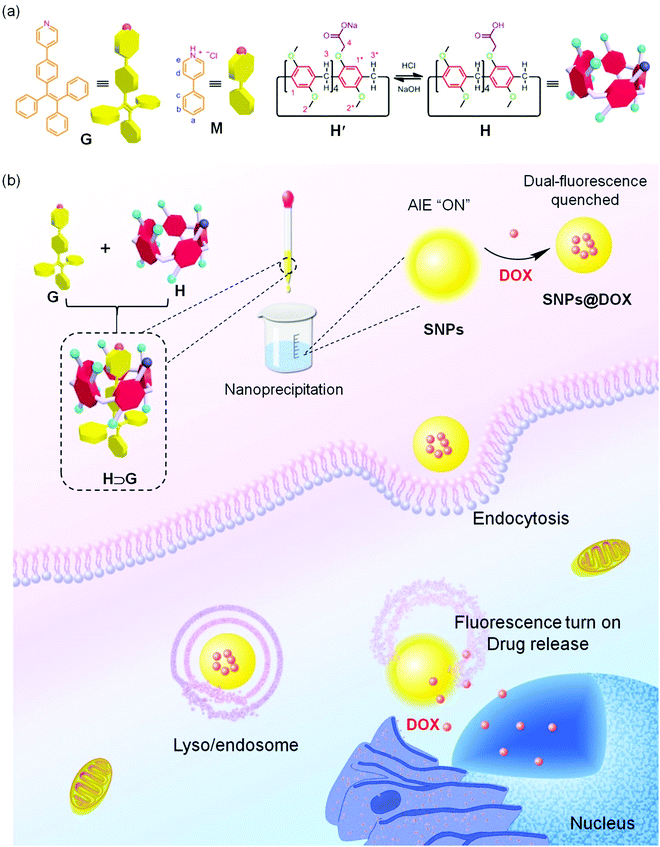 |
| Scheme 1 (a) Chemical structures and cartoon representations of G, M, and H. (b) The fabrication of SNPs@DOX using SNPs as drug nanocarriers and cartoon illustration of imaging-guided drug delivery. SNPs are prepared from the host–guest complex H⊃G through a nanoprecipitation method. After cellular endocytosis, the dual-fluorescence quenched nanoparticles SNPs@DOX are transported into the lyso/endosome, where the loaded drug releases from SNPs due to the protonation of DOX in an acidic microenvironment, thus recovering the fluorescence. | |
Results and discussion
1H NMR spectroscopy was utilized to study the host–guest recognition between H and G (Fig. 1). In order to eliminate the influence on the chemical shifts caused by the protonation and deprotonation during host–guest complexation, we utilized M and deprotonated pillar[5]arene (H′) as model compounds so that the non-covalent interactions could be monitored by detecting the chemical shift changes before and after complexation. Compared with the proton signals of free M, apparent changes in chemical shifts related to the protons on M were observed upon the addition of H′ (Fig. 1a and b). The signals of protons He and Hd displayed an upfield shift after host–guest complexation (Δδ = 0.283 and 0.061 ppm for He and Hd, respectively), indicating that the guest deeply penetrated into the cavity of H′ and these protons were shielded by the electron-rich cyclic structure upon the formation of an inclusion complex.62 In addition, a broadening effect was observed for these peaks caused by the complexation dynamics (Fig. 1b). In addition, slight chemical shift changes were also observed for the peaks related to the protons H1 and H1* (Δδ = 0.008 and 0.014 ppm for H1 and H1*, respectively), which was caused by the host–guest complexation. 2D NOESY NMR spectroscopy was used to reveal the topological structure and relative position of the building blocks in the host–guest complex H′⊃M (Fig. 1d). Intensive nuclear Overhauser effect correlations between the peaks of protons (Ha–e) and protons (H1 and H1*) were observed, which further indicated that M penetrated into the cavity of the cyclic pillar[5]arene host through host–guest interactions.
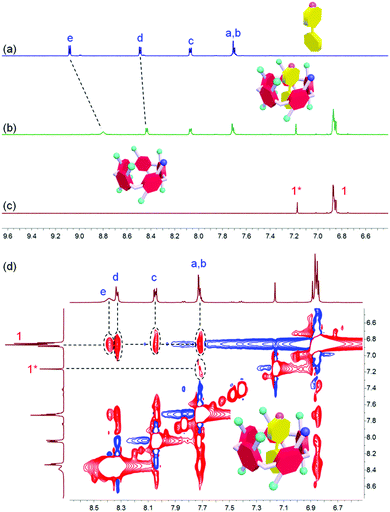 |
| Fig. 1 Partial 1H NMR spectra (400 MHz, acetone-d6/D2O = 1 : 1, room temperature) of (a) M (1.00 mM), (b) H′ (1.00 mM) and M (1.00 mM), and (c) H′ (1.00 mM). (d) Partial NOESY NMR spectrum (400 MHz, acetone-d6/D2O = 1 : 1, room temperature) of H′⊃M (5.00 mM). | |
In order to reveal the complexation thermodynamic behaviors and binding affinity of H⊃G, an isothermal titration calorimetry (ITC) experiment was conducted (Fig. 2). The association constant was measured to be (1.27 ± 0.10) × 104 M−1 with a 1
:
1 complexation stoichiometry. Moreover, the changes in enthalpy and entropy were also calculated from ITC measurement to be −14.1 kJ mol−1 and 31.2 J mol−1 deg−1, respectively, which indicated that the host–guest recognition was driven by advantageous entropy changes with the assistance of enthalpy.63 Direct evidence for the formation of H⊃G came from electrospray ionization mass spectrometry, in which a peak at m/z 1204.4 was detected corresponding to [H⊃G + H]+, which confirmed the 1
:
1 complexation between H and G (Fig. S7, ESI†). For H and G, the proton from the carboxylic acid group on H could transfer to the pyridine unit on G in the principle of undergoing an acid–base reaction, thus forming stable electrostatic interaction between H and G that mainly drove the formation of a host–guest complex.
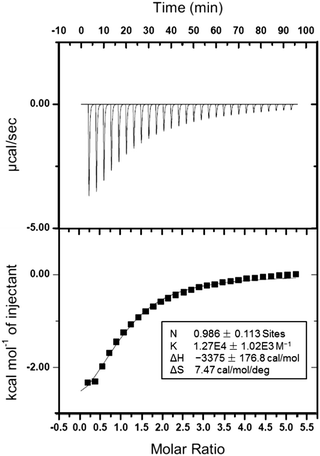 |
| Fig. 2 ITC data of the host–guest complexation between H′ and M at 298.15 K. | |
After establishment of the host–guest complexation, the photophysical properties of free G and H⊃G were studied. The AIEgen-based guest G displayed a classical AIE effect. The fluorescence intensity of G was extremely weak in acetone where it was well dissolved. It should be noted that the emission of G in acetone significantly improved in the presence of H attributed to the host–guest interactions (Fig. 3a and b). Under a UV lamp at 365 nm, the yellow emission of the solution G lighted up in the presence of H (Fig. 3b), further supporting the proposed mechanism shown in Fig. 3a. Gradually enhancing the volume fraction of water (fw) in the mixture of acetone and H2O from 0 to 80 vol%, the fluorescence intensity of G at 550 nm increased slowly. A dramatic enhancement of the fluorescence intensity was monitored by further improving the fw value from 80 to 98 vol% (Fig. 3e). This phenomenon was consistent with other classical AIE dyes. Excitingly, the AIE property was maintained by the formation of H⊃G and a complexation-triggered enhancement of the AIE effect was achieved (Fig. 3c and Fig. S8, ESI†). As shown in Fig. 3e, the fluorescence intensity of H⊃G was always much higher than that of G at the same fw values. The emission intensity of H⊃G was around 2.8 times that of free G when fw reached 98% (Fig. 3c). The mechanism behind the complexation-enhanced AIE effect was that the intramolecular rotation of G was significantly restricted by the formation of a [2]psedorotaxane-type structure (Fig. 3a). The nonradiative decay channels of the AIEgen-based guest were effectively blocked, thus bursting the fluorescent emission. In addition, the hydrophobicity of the AIE-active fluorogen was remarkably increased by incorporating hydrophobic H into this supramolecular complex, which was also favourable to promote the AIE effect by improving the aggregation of H⊃G in aqueous solution.
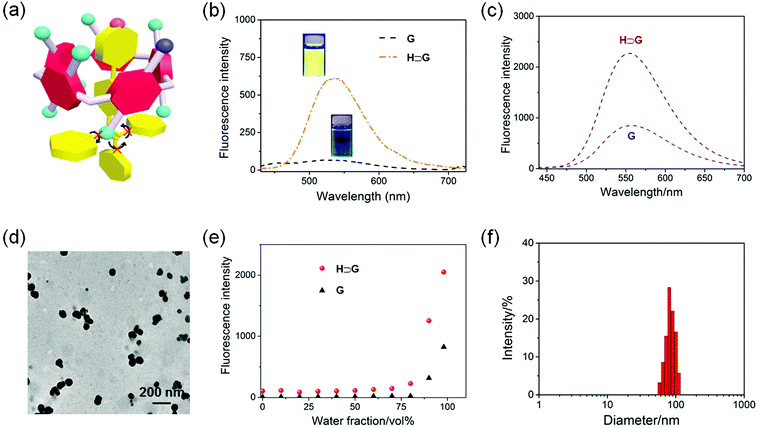 |
| Fig. 3 (a) Cartoon representation of complexation-induced AIE enhancement by restricting the intramolecular rotation of G. (b) Fluorescence spectra of G (100 μM) and H⊃G (100 μM) in acetone. Inset: The photos of the corresponding solution under UV lamp. (c) Fluorescence spectra of G (5.00 μM) and H⊃G (5.00 μM) in aqueous solution. (d) TEM image of SNPs prepared from H⊃G. (e) Plots of the emission intensity at 550 nm versus fw of the aqueous mixtures containing G (5.00 μM) and H⊃G (5.00 μM), respectively. (f) DLS result of the solution containing SNPs. | |
In order to improve the stability of the host–guest complex for biomedical applications, SNPs were prepared through a nanoprecipitation method by slowly adding the acetone solution of H⊃G into water under sonication. As shown in the transmission electron microscopy (TEM) image, spherical nanoparticles with a diameter ranging from 50 to 110 nm were observed (Fig. 3d). The average diameter of these nanoparticles was determined by dynamic light scattering (DLS) to be 87.3 ± 7.9 nm (Fig. 3f), in good agreement with the result obtained from TEM. The stability of the SNPs in the physiological environment was evaluated by DLS measurements, and negligible changes in mean diameter were detected in PBS within 24 h (Fig. S9, ESI†), ensuring the biomedical applications of SNPs.
The internalization pathways of the SNPs were investigated by using various endocytosis inhibitors. Cellular uptake of SNPs by U87MG cells was significantly blocked at 4 °C, demonstrating that the cellular uptake was energy-dependent. Additionally, the endocytosis of SNPs was greatly inhibited in the presence of amiloride-HCl (AMD), chlorpromazine (CPZ) or genistein (Gen). Pre-treatment of the cells with AMD, CPZ or Gen resulted in 23.7%, 51.1% or 22.9% reduction in the cellular internalization (Fig. 4a), respectively, which indicated that the SNPs were internalized mainly through the clathrin-mediated endocytic pathway with the assistance of macropinocytosis- and caveolae-mediated uptake.64,65 These internalization pathways allowed SNPs to undergo an endo/lysosomal transportation for intracellular delivery. A 3-(4′,5′-dimethyl-2′-thiazolyl)-2,5-diphenyltetrazolium bromide (MTT) assay was utilized to assess the cytotoxicity of SNPs. Incubation with H, G, or SNPs for 24 h led to ignorable influence on relative cell viability against both cancerous U87MG and normal HEK293 cells (Fig. S10 and S11, ESI†), a convincing indicator of excellent biocompatibility of these building blocks and the supramolecular complex for biomedical use.
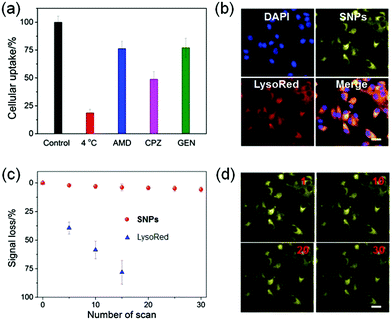 |
| Fig. 4 (a) Cellular internalization of SNPs in the absence and presence of various inhibitors. (b) CLSM images of U87MG cells after 4 h incubation with SNPs. (c) Signal loss of fluorescent emission of SNPs and LysoTracker Red with increasing number of scans. (d) CLSM images of U87MG cells stained with SNPs with increasing number of scans (the number of scans is shown in the upper right corner). | |
Confocal laser scanning microscopy (CLSM) was utilized to reveal the cellular internalization of SNPs and trace their intracellular location. As shown in Fig. 4b, yellow fluorescence was observed in the cytoplasm after 4 h incubation with SNPs, and the signal was well-overlapped with the red fluorescence from LysoTracker Red (LysoRed), suggesting that SNPs mainly accumulated in lyso/endosomes after endocytosis. More intriguingly, the SNPs displayed superior photostability under laser excitation during cell imaging. CLSM images indicated that the fluorescence of SNPs was greatly maintained after 30 scans, and only 5.8% signal loss was determined (Fig. 4c and d). In sharp comparison, the fluorescence signal of LysoRed almost vanished after 15 scans with a signal loss as high as 78.4% (Fig. 4c and Fig. S12, ESI†), because LysoRed was easily quenched at low working concentration by laser excitation. For SNPs, although their outermost layer was possibly photobleached upon exposure to a laser, the nanoparticle structure helped to avoid photo-oxidization due to the resistance of oxygen diffusion into the cores, thus significantly improving the photostability of SNPs.
Considering the hydrophobicity of this binary supramolecular system, SNPs could be further utilized as nanocarriers to encapsulate anticancer drugs driven by π–π stacking and hydrophobic interactions between the drug and H⊃G. Indeed, DOX could be encapsulated into SNPs to form a ternary delivery system (SNPs@DOX) with a drug loading content of 26.7%. Negligible changes in morphology were observed after loading DOX (Fig. S13, ESI†), and only a slight increase in the mean diameter was detected for SNPs@DOX with an average diameter of 104 nm (Fig. S14, ESI†). The successful encapsulation was further confirmed by zeta potential measurements, in which the zeta value increased from −7.8 mV for SNPs to 23.2 mV for SNPs@DOX (Fig. S15 and S16, ESI†).
Interestingly, a huge overlap was detected between the absorption of DOX and the emission of SNPs (Fig. 5a), suggesting that a FRET process could take place in SNPs@DOX, in which the AIEgen G worked as a fluorescent donor and anticancer drug DOX acted as an acceptor. As shown in Fig. 5b, the emission intensity at around 550 nm corresponding to the characteristic fluorescence of SNPs weakened upon the gradual addition of DOX. The disappearance of the AIE behavior in the presence of DOX arose from the FRET from TPE-based fluorogens to DOX,66 because the intermolecular distance between G and DOX was close in the hydrophobic core of SNPs@DOX. Additionally, the fluorescence of DOX self-quenched due to the ACQ effect, which was fundamentally caused by the π–π stacking of DOX molecules with a rigid planar structure. Therefore, a dual-fluorescence-quenched drug delivery system was constructed on the basis of the ETR effect, which was mediated by the FRET and ACQ effects.
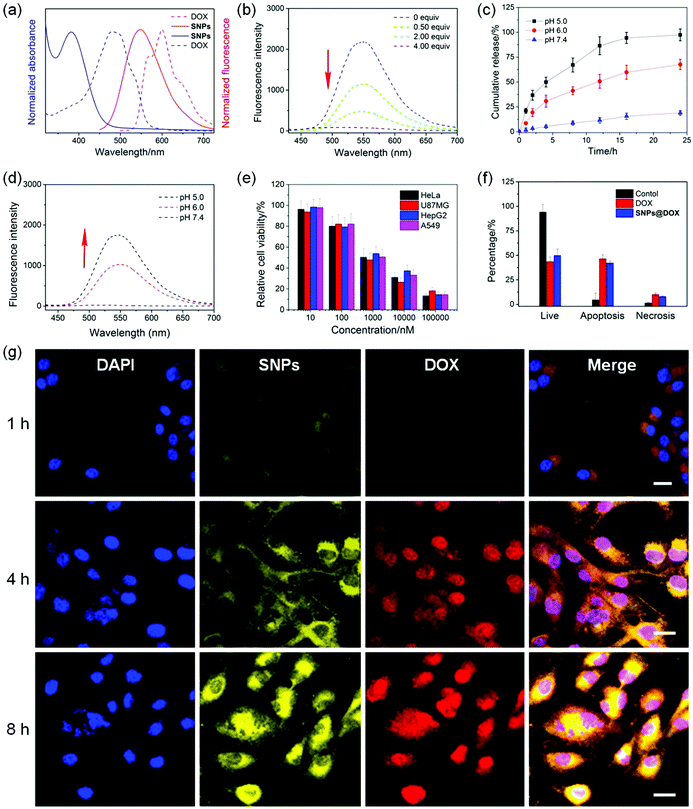 |
| Fig. 5 (a) Absorption and fluorescence emission spectra of SNPs and DOX. (b) Fluorescence spectra of SNPs in the presence of different amounts of DOX. (c) Drug release curves of SNPs@DOX under different pH values. (d) Fluorescence recovery of SNPs@DOX after 8 h incubation under different pH values. (e) Relative cell viability of SNPs@DOX against various cell lines at different concentrations after 24 h incubation. (f) Apoptosis analysis of the U87MG cells treated with different formulations. (g) CLSM images of the U87MG cells incubated with SNPs@DOX for 1 h, 4 h and 8 h, respectively. Scale bars = 25 μm. | |
The neutral DOX in SNPs@DOX could be protonated in an acidic intracellular environment, facilitating its release from the nanoformulation to activate its anticancer capability. In order to mimic the pH gradient from the bloodstream to the intracellular endo/lysosome, the release behaviors of DOX from SNPs@DOX were measured at pH 7.4, 6.0, and 5.0, respectively. Fig. 5c indicated that the release profile was pH-dependent. The ternary system was quite stable at pH 7.4, and only 18.2% of the loaded DOX was released within 24 h incubation. The release rate and amount greatly accelerated in an acidic environment, and 67.5% of the loaded DOX was released from SNPs@DOX at pH 6.0 and 95.4% at pH 5.0 within the same period. More importantly, the ETR effect between SNPs and DOX was interrupted accompanied by the release of DOX, thus recovering the fluorescence of the SNPs (Fig. 5d). Therefore, the drug release process could be traced in real-time by detecting the magnitude and location of the energy transfer-dependent fluorescent variations, allowing imaging-guided drug delivery.
CLSM was used to study the internalization and in situ drug release behaviour of SNPs@DOX. After 1 h of incubation with SNPs@DOX, yellow fluorescence from SNPs and red fluorescence from DOX was found in the cytoplasm of the U87MG cells (Fig. 5g). The fluorescence signals became much more intensive by extending the incubation time to 4 h, which demonstrated that the loaded DOX successfully escaped from SNPs@DOX. Attributed to the nucleus-targeting ability of DOX, the released anticancer drug entered into the cell nucleus, as evidenced by the colocolization of blue fluorescence from DAPI and red fluorescence from DOX. The fluorescence intensity of SNPs and DOX further increased after 8 h incubation, and most of the drug translocated into the nucleus where its anticancer activity took place. After being internalized by the cancer cells, SNPs@DOX was transported into the endo/lysosomes with a relatively low pH, where the neutral DOX could be protonated into the cationic state, leading to the release of loaded DOX from SNPs@DOX. As a result, the silenced fluorescence “woke up” by interrupting the ETR effect between the SNPs and DOX.
The MTT assay was employed to evaluate the anticancer efficacy of SNPs@DOX, and free DOX was chosen as a control. Significant growth inhibition was observed for various cancer cell lines treated with SNPs@DOX at a high DOX concentration, including HeLa, U87MG, HepG2, and A549 cells. According to the MTT assay, the half maximal inhibitory concentrations (IC50) of SNPs@DOX were calculated to be 2.14 ± 0.17, 1.80 ± 0.11, 2.88 ± 0.20, and 1.96 ± 0.14 μM against HeLa, U87MG, HepG2, and A549 cells, respectively. The anticancer efficacy of SNPs@DOX was comparable to that of free DOX, whose IC50 values were 3.25 ± 0.28, 1.77 ± 0.14, 2.63 ± 0.18, and 1.53 ± 0.10 μM against HeLa, U87MG, HepG2, and A549 cells, respectively (Fig. S18, ESI†). The quantities of living cells, apoptosis cells, and necrosis cells were determined after the U87MG cells were administrated with free DOX and SNPs@DOX. The corresponding apoptotic and necrotic cell counts in these two therapeutic groups were very close, in good agreement with the results obtained from the MTT assay. Similarly, SNPs could be used as feasible nanocarriers to load other hydrophobic anticancer drugs, such as camptothecin and paclitaxel. Compared with the free drugs, the potency of these supramolecular drug delivery systems was greatly maintained benefiting from the non-covalent interactions (Fig. S19–S26, ESI†). These studies demonstrated that the supramolecular system possessing AIE properties showed promising potential in imaging-guided drug delivery.67–69
Conclusions
In summary, we established a new host–guest recognition between a carboxylate-modified pillar[5]arene H and a TPE-based guest G. The intramolecular rotation of the aromatic rings of G was effectively restricted after the formation of H⊃G, in which the pyridine head deeply penetrated into the cavity of H driven by the electrostatic interaction. Benefiting from the complexation-enhanced AIE effect, highly emissive SNPs were prepared, which could be used for living cell imaging with excellent photostability. More interestingly, SNPs were able to load DOX to afford a self-imaging drug delivery system. Mediated by FRET and ACQ effects, the fluorescence from SNPs and DOX were both extinguished arising from the ETR effect. The release of loaded DOX from SNPs@DOX woke up the silenced fluorescence triggered in an acidic microenvironment, which allowed the in situ detection of drug release in cells. In vitro studies verified that the anticancer capability of the drugs in the nanoformulations was effectively preserved using this supramolecular nanomaterial. The present study provides a novel supramolecular method for the construction of imaging-guided drug delivery systems, which have great potential applications in the field of cancer treatment.
Author contributions
D. Liu, J. Wang, M. Liu, D. Wu, and J. Shen conceived and designed the research. D. Liu, S. Qi, M. Li, and D. Wu synthesized the compounds and investigated the host–guest complexation. D. Liu, X. Du, X. Wang, B. Ren, M. Liu, and J. Shen conducted the in vitro studies. D. Liu, J. Wang, M. Liu, D. Wu, and J. Shen co-wrote the paper.
Conflicts of interest
The authors declare no competing financial interest.
Acknowledgements
This work was supported by the Key Laboratory Construction Project of Jilin Province (No. 20190901002JC), Innovation Building Projects of Jilin Province (No. 2019C007), Zhejiang Provincial Natural Science Foundation of China (LQ20B040001) and the National Natural Science Foundation of China (51603184).
Notes and references
- R. Weissleder and M. J. Pittet, Imaging in the era of molecular oncology, Nature, 2008, 452, 580–589 CrossRef CAS PubMed.
- E. Meijering, A. E. Carpenter, H. Peng, F. A. Hamprecht and J.-C. Olivo-Marin, Imagining the future of bioimage analysis, Nat. Biotechnol., 2016, 34, 1250–1255 CrossRef CAS PubMed.
- J. R. W. Conway, N. O. Carragher and P. Timpson, Developments in preclinical cancer imaging: innovating the discovery of therapeutics, Nat. Rev. Cancer, 2014, 14, 314–328 CrossRef CAS PubMed.
- A. L. Antaris, H. Chen, K. Cheng, Y. Sun, G. Hong, C. Qu, S. Diao, Z. Deng, X. Hu, B. Zhang, X. Zhang, O. K. Yaghi, Z. R. Alamparambil, X. Hong, Z. Cheng and H. Dai, A small-molecule dye for NIR-II imaging, Nat. Mater., 2016, 15, 235–242 CrossRef CAS PubMed.
- G. Hong, A. Antaris and H. Dai, Near-infrared fluorophores for biomedical imaging, Nat. Biomed. Eng., 2017, 1, 0010 CrossRef CAS.
- M. Gao, F. Yu, C. Lv, J. Choo and L. Chen, Fluorescent chemical probes for accurate tumor diagnosis and targeting therapy, Chem. Soc. Rev., 2017, 46, 2237–2271 RSC.
- D. Wang and B. Z. Tang, Aggregation-induced emission luminogens for activity-based sensing, Acc. Chem. Res., 2019, 52, 2559–2570 CrossRef CAS.
- J. Mei, N. L. C. Leung, R. T. K. Kwok, J. W. Y. Lam and B. Z. Tang, Aggregation-induced emission: together we shine, united we soar!, Chem. Rev., 2015, 115, 11718–11940 CrossRef CAS PubMed.
- S. Wang, Y. Fan, D. Li, C. Sun, Z. Lei, L. Lu, T. Wang and F. Zhang, Nat. Commun., 2019, 10, 1058 CrossRef PubMed.
- H.-B. Cheng, Y. Li, B. Z. Tang and J. Yoon, Chem. Soc. Rev., 2020, 49, 21–31 RSC.
- Y. Li, S. Liu, T. Han, H. Zhang, C. Chuah, R. T. K. Kwok, J. W. Y. Lam and B. Z. Tang, Sparks fly when AIE meets with polymers, Mater. Chem. Front., 2019, 3, 2207–2220 RSC.
- J. Mei, Y. Hong, J. W. Y. Lam, A. Qin, Y. Tang and B. Z. Tang, Aggregation-induced emission: the whole is more brilliant than the parts, Adv. Mater., 2014, 26, 5429–5479 CrossRef CAS.
- P. Gao, W. Pan, N. Li and B. Tang, Fluorescent probes for organelle-targeted bioactive species imaging, Chem. Sci., 2019, 10, 6035–6071 RSC.
- G. Feng and B. Liu, Aggregation-induced emission (AIE) dots: emerging theranostic nanolights, Acc. Chem. Res., 2018, 51(6), 1404–1414 CrossRef CAS.
- Z. Zhang, Z. Zhao, L. Wu, S. Lu, S. Ling, G. Li, L. Xu, L. Ma, Y. Hou, X. Wang, X. Li, G. He, K. Wang, B. Zou and M. Zhang, Emissive platinum(II) cages with reverse fluorescence resonance energy transfer for multiple sensing, J. Am. Chem. Soc., 2020, 142, 2592–2600 CrossRef CAS PubMed.
- G. Yu, R. Zhao, D. Wu, F. Zhang, L. Shao, J. Zhou, J. Yang, G. Tang, X. Chen and F. Huang, Pillar[5]arene-based amphiphilic supramolecular brush copolymers: fabrication, controllable self-assembly and application in self-imaging targeted drug delivery, Polym. Chem., 2016, 7, 6178–6188 RSC.
- P. Zhang, X. Nie, M. Gao, F. Zeng, A. Qin, S. Wu and B. Z. Tang, A highly selective fluorescent nanoprobe based on AIE and ESIPT for imaging hydrogen sulfide in live cells and zebrafish, Mater. Chem. Front., 2017, 1, 838–845 RSC.
- X. Gao, G. Feng, P. N. Manghnani, F. Hu, N. Jiang, J. Liu, B. Liu, J. Z. Sun and B. Z. Tang, A two-channel responsive fluorescent probe with AIE characteristics and its application for selective imaging of superoxide anions in living cells, Chem. Commun., 2017, 53, 1653–1656 RSC.
- M. Li, Y. Gao, Y. Yuan, Y. Wu, Z. Song, B. Z. Tang, B. Liu and Q. C. Zheng, One-step formulation of targeted aggregation-induced emission dots for image-guided photodynamic therapy of cholangiocarcinoma, ACS Nano, 2017, 11, 3922–3932 CrossRef CAS PubMed.
- D. Wang and B. Z. Tang, Aggregation-induced emission luminogens for activity-based sensing, Acc. Chem. Res., 2019, 52, 2559–2570 CrossRef CAS PubMed.
- G. Jin, G. Feng, W. Qin, B. Z. Tang, B. Liu and K. Li, Multifunctional organic nanoparticles with aggregation-induced emission (AIE) characteristics for targeted photodynamic therapy and RNA interference therapy, Chem. Commun., 2016, 52, 2752–2755 RSC.
- D. Ding, K. Li, B. Liu and B. Z. Tang, Bioprobes based on AIE fluorogens, Acc. Chem. Res., 2013, 46, 2441–2453 CrossRef CAS PubMed.
- G. Yu, M. Zhang, M. L. Saha, Z. Mao, J. Chen, Y. Yao, Z. Zhou, Y. Liu, C. Gao, F. Huang, X. Chen and P. J. Stang, Antitumor activity of a unique polymer that incorporates a fluorescent self-assembled metallacycle, J. Am. Chem. Soc., 2017, 139, 15940–15949 CrossRef CAS PubMed.
- X. Yan, P. Wei, Y. Liu, M. Wang, C. Chen, J. Zhao, G. Li, M. L. Saha, Z. Zhou, Z. An, X. Li and P. J. Stang, Endo- and exo-functionalized tetraphenylethylene M12L24 nanospheres: fluorescence emission inside a confined space, J. Am. Chem. Soc., 2019, 141, 9673–9679 CrossRef CAS PubMed.
- R. Zhang, G. Niu, Q. Lu, X. Huang, J. H. C. Chau, R. T. K. Kwok, X. Yu, M.-H. Li, J. W. Y. Lam and B. Z. Tang, Cancer cell discrimination and dynamic viability monitoring through wash-free bioimaging using AIEgens, Chem. Sci., 2020, 11, 7676–7684 RSC.
- Y. Gao, H. Zhang, Z. He, F. Fang, C. Wang, K. Zeng, S. Gao, F. Meng, L. Luo and B. Z. Tang, Multicationic AIEgens for unimolecular photodynamic theranostics and two-photon fluorescence bioimaging, Mater. Chem. Front., 2020, 4, 1623–1633 RSC.
- G. Yu, G. Tang and F. Huang, Supramolecular enhancement of aggregation-induced emission and its application in cancer cell imaging, J. Mater. Chem. C, 2014, 2, 6609–6617 RSC.
- D. Wu, Y. Li, J. Yang, J. Shen, J. Zhou, Q. Hu, G. Yu, G. Tang and X. Chen, Supramolecular nanomedicine constructed from cucurbit[8]uril-based amphiphilic brush copolymer for cancer therapy, ACS Appl. Mater. Interfaces, 2017, 9(51), 44392–44401 CrossRef CAS PubMed.
- Y. Liao, B. Li, Z. Zhao, Y. Fu, Q. Tan, X. Li, W. Wang, J. Yin, H. Shan, B. Z. Tang and X. Huang, Targeted theranostics for tuberculosis: a rifampicin-loaded aggregation-induced emission carrier for granulomas
tracking and anti-infection, ACS Nano, 2020, 14, 8046–8058 CrossRef CAS PubMed.
- X. Yan, M. Wang, T. R. Cook, M. Zhang, M. L. Saha, Z. Zhou, X. Li, F. Huang and P. J. Stang, Light-emitting superstructures with anion effect: coordination-driven self-assembly of pure tetraphenylethylene metallacycles and metallacages, J. Am. Chem. Soc., 2016, 138, 4580–4588 CrossRef CAS PubMed.
- G. Yu, D. Wu, Y. Li, Z. Zhang, L. Shao, J. Zhou, Q. Hu, G. Tang and F. Huang, A pillar[5]arene-based [2]rotaxane lights up mitochondria, Chem. Sci., 2016, 7, 3017–3024 RSC.
- J. Li, K. Shi, M. Drechsler, B. Z. Tang, J. Huang and Y. Yan, A supramolecular fluorescent vesicle based on a coordinating aggregation induced emission amphiphile: insight into the role of electrical charge in cancer cell division, Chem. Commun., 2016, 52, 12466–12469 RSC.
- J. Shi, Y. Li, Q. Li and Z. Li, Enzyme-responsive bioprobes based on the mechanism of aggregation-induced emission, ACS Appl. Mater. Interfaces, 2018, 10, 12278–12294 CrossRef CAS.
- D. Wu, L. Shao, Y. Li, Q. Hu, F. Huang, G. Yu and G. Tang, A boron difluoride dye showing the aggregation-induced emission feature and high sensitivity to intra- and extra-cellular pH changes, Chem. Commun., 2016, 52, 541–544 RSC.
- G. Yu, T. R. Cook, Y. Li, X. Yan, D. Wu, L. Shao, J. Shen, G. Tang, F. Huang, X. Chen and P. J. Stang, A tetraphenylethene-based highly emissive metallacage as a component of theranostic supramolecular nanoparticles, Proc. Natl. Acad. Sci. U. S. A., 2016, 113, 13720–13725 CrossRef CAS PubMed.
- J. Qi, X. Duan, Y. Cai, S. Jia, C. Chen, Z. Zhao, Y. Li, H.-Q. Peng, R. T. K. Kwok, J. W. Y. Lam, D. Ding and B. Z. Tang, Simultaneously boosting the conjugation, brightness and solubility of organic fluorophores by using AIEgens, Chem. Sci., 2020, 11, 8438–8447 RSC.
- L. Yang, W. Fang, Y. Ye, Z. Wang, Q. Hu and B. Z. Tang, Redox-responsive fluorescent AIE bioconjugate with aggregation enhanced retention features for targeted imaging reinforcement and selective suppression of cancer cells, Mater. Chem. Front., 2019, 3, 1335–1340 RSC.
- W. Qin, N. Alifu, Y. Cai, J. W. Y. Lam, X. He, H. Su, P. Zhang, J. Qian and B. Z. Tang, Synthesis of an efficient far-red/near-infrared luminogen with AIE characteristics for in vivo bioimaging applications, Chem. Commun., 2019, 55, 5615–5618 RSC.
- C. Zhang, S. Jin, S. Li, X. Xue, J. Liu, Y. Huang, Y. Jiang, W.-Q. Chen, G. Zou and X.-J. Liang, Imaging intracellular anticancer drug delivery by self-assembly micelles with aggregation-induced emission (AIE micelles), ACS Appl. Mater. Interfaces, 2014, 6, 5212–5220 CrossRef CAS PubMed.
- X. Ni, X. Zhang, X. Duan, H.-L. Zheng, X.-S. Xue and D. Ding, Near-infrared afterglow luminescent aggregation-induced emission dots with ultrahigh tumor-to-liver signal ratio for promoted image-guided cancer surgery, Nano Lett., 2019, 19, 318–330 CrossRef CAS PubMed.
- S. J. Barrow, S. Kasera, M. J. Rowland, J. Barrio and O. A. Scherman, Cucurbituril-based molecular recognition, Chem. Rev., 2015, 115, 12320–12406 CrossRef CAS PubMed.
- G. Yu, K. Jie and F. Huang, Supramolecular amphiphiles based on host–guest molecular recognition motifs, Chem. Rev., 2015, 115, 7240–7303 CrossRef CAS PubMed.
- T. Ogoshi, T. Yamagishi and Y. Nakamoto, Pillar-shaped macrocyclic hosts pillar[n]arenes: new key players for supramolecular chemistry, Chem. Rev., 2016, 116, 7937–8002 CrossRef CAS PubMed.
- M. Xue, Y. Yang, X. Chi, Z. Zhang and F. Huang, Pillararenes, a new class of macrocycles for supramolecular chemistry, Acc. Chem. Res., 2012, 45, 1294–1308 CrossRef CAS PubMed.
- X. Ji, M. Ahmed, L. Long, N. M. Khashab, F. Huang and J. L. Sessler, Adhesive supramolecular polymeric materials constructed from macrocycle-based host–guest interactions, Chem. Soc. Rev., 2019, 48, 2682–2697 RSC.
- A. Harada, Cyclodextrin-based molecular machines, Acc. Chem. Res., 2001, 34, 456–464 CrossRef CAS PubMed.
- J. Zhou, G. Yu and F. Huang, Supramolecular chemotherapy based on host–guest molecular recognition: a novel strategy in the battle against cancer with a bright future, Chem. Soc. Rev., 2017, 46, 7021–7053 RSC.
- D.-S. Guo and Y. Liu, Supramolecular chemistry of p-Sulfonatocalix[n]arenes and its biological applications, Acc. Chem. Res., 2014, 47, 1925–1934 CrossRef CAS PubMed.
- D. Xia, P. Wang, X. Ji, N. M. Khashab, J. L. Sessler and F. Huang, Functional supramolecular polymeric networks: the marriage of covalent polymers and macrocycle-based host–guest interactions, Chem. Rev., 2020, 120, 6070–6123 CrossRef CAS PubMed.
- N. L. Strutt, H. Zhang, S. T. Schneebeli and J. F. Stoddart, Functionalizing pillar[n]arenes, Acc. Chem. Res., 2014, 47, 2631–2642 CrossRef CAS PubMed.
- T. Kakuta, T. Yamagishi and T. Ogoshi, Stimuli-responsive supramolecular assemblies constructed from pillar[n]arenes, Acc. Chem. Res., 2018, 51, 1656–1666 CrossRef CAS PubMed.
- H. Zhu, L. Shangguan, B. Shi, G. Yu and F. Huang, Recent progress in macrocyclic amphiphiles and macrocyclic host-based supra-amphiphiles, Mater. Chem. Front., 2018, 2, 2152–2174 RSC.
- Y. Wang, G. Ping and C. Li, Efficient complexation between pillar[5]arenes and neutral guests: from host–guest chemistry to functional materials, Chem. Commun., 2016, 52, 9858–9872 RSC.
- J.-D. Ding, W.-J. Jin, Z. Pei and Y. Pei, Morphology transformation of pillararene-based supramolecular nanostructures, Chem. Commun., 2020, 56, 10113–10126 RSC.
- G. Yu, W. Yu, L. Shao, Z. Zhang, X. Chi, Z. Mao, C. Gao and F. Huang, A pillar[5]arene-based supramolecular diblock copolymeric amphiphile: fabrication and application in Targeted drug delivery, Adv. Funct. Mater., 2016, 26, 8999–9008 CrossRef CAS.
- J.-Y. Chen and J.-L. Hou, Controllable synthetic ion channels, Org. Chem. Front., 2018, 5, 1728–1736 RSC.
- H. Li, D.-X. Chen, Y.-L. Sun, Y. B. Zheng, L.-L. Tan, P. S. Weiss and Y.-W. Yang, Viologen-mediated assembly of and sensing with carboxylatopillar[5]arene-modified gold nanoparticles, J. Am. Chem. Soc., 2013, 135, 1570–1576 CrossRef CAS PubMed.
- J. Chen, Y. Zhang, Z. Meng, L. Guo, X. Yuan, Y. Zhang, Y. Chai, J. L. Sessler, Q. Meng and C. Li, Supramolecular combination chemotherapy: a pH-responsive co-encapsulation drug delivery system, Chem. Sci., 2020, 11, 6275–6282 RSC.
- G. Yu, J. Yang, X. Fu, Z. Wang, L. Shao, Z. Mao, Y. Liu, Z. Yang, F. Zhang, W. Fan, J. Song, Z. Zhou, C. Gao, F. Huang and X. Chen, Supramolecular hybrid material constructed
from graphene oxide and pillar[6]arene-based host–guest complex as a ultrasound and photoacoustic signals nanoamplifier, Mater. Horiz., 2018, 5, 429–435 RSC.
- H. Liang, B. Hua, F. Xu, L.-S. Gan, L. Shao and F. Huang, Acid/base-tunable unimolecular chirality switching of a pillar[5]azacrown pseudo[1]catenane, J. Am. Chem. Soc., 2020, 142, 19772–19778 CrossRef CAS PubMed.
- N. L. Strutt, R. S. Forgan, J. M. Spruell, Y. Y. Botros and J. F. Stoddart, Monofunctionalized Pillar[5]arene as a Host for Alkanediamines, J. Am. Chem. Soc., 2011, 133, 5668–5671 CrossRef CAS.
- G. Yu, B. Hua and C. Han, Proton transfer in host–guest complexation between a difunctional pillar[5]arene and alkyldiamines, Org. Lett., 2014, 16, 2486–2489 CrossRef CAS.
- P. Wei, X. Yan, J. Li, Y. Ma and F. Huang, Two 2:3 copillar[5]arene constitutional isomers: syntheses, crystal structures and host–guest complexation ofderivatives with dicarboxylic acid sodium salts in water, Chem. Commun., 2013, 49, 1070–1072 RSC.
- X. Hu, J. Hu, J. Tian, Z. Ge, G. Zhang, K. Luo and S. Liu, Cell-penetrating hyperbranched polyprodrug amphiphiles for synergistic reductive milieu-triggered drug release and enhanced magnetic resonance signals, J. Am. Chem. Soc., 2013, 135, 17617–17629 CrossRef CAS PubMed.
- V. Z. Sun, Z. Li, T. J. Deming and D. T. Kamei, Intracellular fates of cell-penetrating block copolypeptide vesicles, Biomacromolecules, 2011, 12, 10–13 CrossRef CAS PubMed.
- X. Xue, S. Jin, C. Zhang, K. Yang, S. Huo, F. Chen, G. Zou and X.-J. Liang, Probe-inspired nano-prodrug with dual-color fluorogenic property reveals spatiotemporal drug release in living cells, ACS Nano, 2015, 9, 2729–2739 CrossRef CAS PubMed.
- T. Xiao, L. Qi, W. Zhong, C. Lin, R. Wang and L. Wang, Stimuli-responsive nanocarriers constructed from pillar[n]arene-based supra-amphiphiles, Mater. Chem. Front., 2019, 3, 1973–1993 RSC.
- G. Yu and X. Chen, Host–guest chemistry in supramolecular theranostics, Theranostics, 2019, 9, 3041–3074 CrossRef CAS PubMed.
- M. J. Webber and R. Langer, Drug delivery by supramolecular design, Chem. Soc. Rev., 2017, 46, 6600–6620 RSC.
Footnote |
† Electronic supplementary information (ESI) available: Details of synthesis procedures, NMR spectra and other supplementary information. See DOI: 10.1039/d0qm00974a |
|
This journal is © the Partner Organisations 2021 |
Click here to see how this site uses Cookies. View our privacy policy here.