DOI:
10.1039/D0QM00621A
(Research Article)
Mater. Chem. Front., 2021,
5, 268-283
Understanding the lipid production mechanism in Euglena gracilis with a fast-response AIEgen bioprobe, DPAS†
Received
24th August 2020
, Accepted 2nd October 2020
First published on 9th October 2020
Abstract
Lipid bodies are lipid-rich organelles that can regulate the storage of neutral lipids as energy sources in organisms. Visualisation of lipid droplets is an effective approach to understand lipid dynamics in microalgae. This study explores the required environmental conditions to yield lipid in a microalgal species Euglena gracilis as the biofunctional component using a lipid-specific aggregation-induced emission fluorogen, DPAS (C20H16N2O), and compares it to the commercial lipid staining probe BODIPY for visualising the lipid production in vivo. Five treatments are investigated for lipid production: (1) modified Cramer–Myers medium (MCM), (2) MCM without nitrogen (−), (3) MCM without nitrogen (−) and calcium (−), (4) MCM without nitrogen (−) and calcium (−), but with glucose (+), and (5) MCM without nitrogen (−) and calcium (−), but with glucose (+). Illumination was continuous at a rate of 70 mmol photons per m−2 s−1 in all treatments except with no light for treatment of 5. Distinct lipid droplets are labelled with DPAS and detected by confocal microscopy and flow cytometry to clarify the understanding of the lipid enrichment mechanism under various conditions. Treatment 1 indicates low lipid production in E. gracilis under an autotrophic condition. DPAS benefits from a very low background signal, and therefore, it is more sensitive than BODIPY for semiquantitative in vivo fluorescence measurements. Co-staining in the presence of BODIPY and chlorophyll also indicates that DPAS is suitable for multicolour imaging with red and green fluorophores. The present study demonstrates that DPAS is a highly effective biocompatible and photostable fluorophore for rapid and sensitive visualisation of lipid droplets. This novel staining method could be used to screen microalgae that have a potential to produce lipid droplets as a health supplement for humans.
1. Introduction
With the challenges of the population growth projection over 10.9 billion by the end of 21st century,1 extensive research on microalgae has been significantly focused due to their inherent renewable, eco-friendly, and biofunctional attributes. The valuable metabolites obtained from microalgae are important for a number of nutraceutical and pharmaceutical purposes, and the market for these products is expanding rapidly. Owing to the advantages of fast growth rates and higher oil yielding capabilities, these organisms have the potential to serve the world not only as the alternative sources of third-generation biofuel,2,3 but also for the procurement of high-valued balanced dietary constituents of antioxidants, vitamins, PUFAs and other health beneficiary bioactive compounds.4 The dietary incorporation of n-3 fatty acids has long been significant, since people habituated with more LC-PUFA intake was being studied as having significantly lower risk factors associated with coronary heart disease and stroke than more C18 fatty acid consumption.5 Currently, most of the human ω-3 LC-PUFA requirements are sourced by marine fish, which is under the threat of dwindling supplies because of environmental factors and pollutant contaminations.6 Therefore, there are ongoing efforts to enrich oilseed plants with LC-PUFAs via metabolic engineering,7 whereas microalgae are more useful due to their superior growth and abilities to utilize the wastewater and non-arable lands.
Lipid biosynthesis in algae depends on various factors such as nutrient supply, availability of essential carbon sources, light, and temperature. Under nutrient-replete favourable conditions, the carbon flux is often directed towards the synthesis of macromolecules and other cellular constituents.8,9 Conversely, under nitrogen-starved conditions, cellular carbon mostly becomes involved in the synthesis of non-N-containing compounds, especially lipids, which is a common practice used to increase lipid production in different microalgae.10–12 Starved conditions with other nutrients such as phosphorus and sulphur have also been found to induce lipid production in Scenedesmus sp. and Chlamydomonas reinhardtii, respectively.13,14 Reflective rises in lipid contents in calcium-starved cultures have also been observed in Chlorella vulgaris and Scenedesmus obliquus,15 while inhibition of neutral lipid synthesis during nitrogen starvation has been observed due to the exposure of voltage-gated and ligand-gated Ca2+ channel blockers in Chlorella sp. C2.16
Due to the photosynthetic properties of microalgae, autotrophic growth is the most common practice for algae culture where natural light energy and atmospheric CO2 can be utilised as the carbon source. Some limiting factors, however, such as low light penetrability in intensive cultures, difficulties in the establishment of the desired single species culture, maintenance of environmental growth parameters, and laborious and poor feasibility in the production of the anticipated biofunctional components have persuaded researchers to seek mixotrophic and heterotrophic conditions in closed and controlled environments.17 As an alternative carbon source to support the growth under these conditions, glucose, glycerol and acetate are frequently used, which are also species-specific.18,19
Euglena gracilis is one of the foregoing studied eukaryotic microalgae in cell biology and biochemistry.20 This unicellular, photosynthetic protist, often found in freshwater habitats, is able to harness multiple bioactive compounds such as proteins, vitamins, lipids, and unique β-1,3-glucan paramylon. Among these, paramylon has already been commercialised as an immunostimulatory, anti-tumor, and anti-HIV agent in nutraceuticals.21–23Euglena can also utilise CO2 and water for the production of macromolecular organic matter in the form of lipids, the composition of which also varies depending on the culture condition.24,25 When aerobically grown E. gracilis cells are transferred into anaerobic conditions, they can degrade paramylon to synthesise and accumulate wax esters that consist of medium-chain fatty acids and alcohols with 14:0 carbon chains as the major constituent.26–29 Generally, in the mitochondria, even-numbered fatty acid synthesis starts from acetyl-CoA, whereas during wax ester fermentation under anaerobic conditions, odd-numbered fatty acid synthesis involves propionyl-CoA under the reaction of fumarate reductase and cofactor rhodoquinone.30 Because of its lower freezing point and good cetane number (66.2), myristic acid (C14:0) has been found to be more suitable as a drop-in jet fuel than other algae-produced medium-length fatty acids such as palmitic acid (C16:0) and stearic acid (C18:0).31 Apart from this, while E. gracilis cells can be allowed to grow under photoautotrophic, photomixotrophic and heterotrophic conditions, the membrane-lipid compositions, especially thylakoid glycolipids monogalactosyldiacylglycerol (MGDG), digalactosyldiacylglycerol (DGDG) and sulfoquinovosyldiacylglycerol (SQDG) are also different.32 It is evident, therefore, that the production of various types of lipids in this species depends greatly on culture conditions and the implementation technologies.
To date, however, despite our knowledge of different cultivation techniques of microalgae, lipid-inducing conditions have been unable to achieve their full potential, due to the limited research into the broader physiological roles of lipid drops in living cells. Even the size of these unique organelles can be related to specific biological functions. Similarly, there is limited understanding of the roles of smaller droplets in mobilising lipid droplet-associated enzymes by changing the surface area ratio of triacylglycerols (TAGs).33 Investigation of the different physiological roles of lipid droplets in microalgae and their interfaces with other cellular organelles, using reliable imaging tools, is critical, and could expand opportunities for algal lipid production by elucidating the structural change mechanisms associated with different environmental stimuli.34 However, research into algal lipids has not been fully exploited because of the limitation of the visualisation tools that could allow rapid and non-invasive evaluation of lipid conditions in vivo.
Currently, lipid compositions are determined and quantified by multidisciplinary approaches such as thin-layer chromatography (TLC),35 gas chromatography (GC),36 liquid chromatography (LC),37 enzyme-linked immunosorbent assay (ELISA),38 nuclear magnetic resonance (NMR),39 flow cytometry,40 and mass spectrometry (MS).41 Among the non-destructive methods, electron microscopy, immunofluorescence microscopy,42 Raman microscopy,43,44 coherent anti-Stokes Raman scattering microscopy,45 and direct organelle mass spectrometry46 have also been used for lipid determination.47 Recent developments of virtual-freezing48 and label-free multicolor chemical imaging techniques by high-speed stimulated Raman scattering49 have also shown promises of improved imaging by flow cytometry in lipidomics.
For cost-effective rapid imaging and semiquantitative analysis of neutral lipids in microalgae, different lipid-specific dyes have been used by researchers.34,50 One of the commonest problems associated with the use of these dyes is the adjustment of the dye acquisition and penetration techniques in microalgae. Severe aggregation-caused quenching (ACQ) at higher concentrations and a self-decomposition phenomenon in a dilute stage can reduce photostability, most often making their usage difficult for fluorescence imaging.51,52 Among lipid-specific fluorescent dyes, nile red (9-diethylamino-5H-benzo[a]phenoxazine-5-one),53 BODIPY 493/503 (4,4-difluoro-1,3,5,7,8-pentamethyl-4-bora-3a,4a-diaza-s-indacene)54 and BODIPY 505/515 (4,4-difluoro-1,3,5,7-tetramethyl-4-bora-3a,4a-diaza-s-indacene)55 are the most frequently used fluorophores in fluorescent microscopy. Despite having some advantages, nile red has several limitations that make its frequent use tricky. The broad absorption and emission spectra of nile red often produce overlapping signals between the yellow and red spectra, while the higher sensitivity to the environmental polarity sometimes results in a blueshift of peak emission during lower surrounding polarity.56 Penetration of this dye in microalgae also requires pre-treatment that affects the cellular viability,57 whereas interactions with other low-density proteins and cellular structures with hydrophobic domains have also been reported.58,59 Furthermore, limitations in long-term preservation, as well as the aggregation of the excess dye and relative interference with the red channel of chlorophyll autofluorescence, makes application of this dye challenging in algal research.60 In comparison to nile red, BODIPY is more lipid droplet-specific, relatively insensitive to the surrounding polarity and more cell permeable, though BODIPY 505/515 is more photostable than its close analogue BODIPY 493/503.57,61 However, the non-fluorogenic nature of BODIPY can produce a strong background signal, while a weak correlation between lipid content and BODIPY fluorescence intensity has also been reported.62,63
To address the ACQ and self-decomposition phenomena of the traditional dyes, fluorophores with opposite characteristics could be exploited to facilitate optimum research outputs.64 Recently, several aggregation-induced emission (AIE)-based nanoprobes have successfully been implemented in different studies for human welfare.65–67 Among different lipid-specific dyes with AIE properties,34 DPAS (C20H16N2O) is a new class of AIE luminogen (AIEgen), has been successfully used in recent research to label lipid droplets at significantly higher concentrations than previously and can produce brighter images, features of which are very promising to overcome the ACQ problems in fluorescence microscopy.68 However, as the dye was used in animal cells, analysis of its penetrability and lipid labelling efficacy is required across multiple cell types to ensure its universality with different cellular compositions.
In the present study, we investigated algal growth and lipid production under different conditions such as mixotrophic, heterotrophic, and nitrogen and calcium limitations and aimed to identify the environmental conditions to maximise health beneficiary lipid biosynthesis in E. gracilis, which is a facultative organism with both plant and animal characteristics.69 Glucose was used as a source of organic carbon to facilitate lipid production in E. gracilis. More importantly, we developed a novel method to visualise lipid droplets in the complex cell structure of microalgae using the lipid-specific AIEgen to understand the enriching mechanism of lipid droplets under a specified condition.
2. Experiment
2.1. Establishment of Euglena gracilis culture and lipid induction
From E. gracilis specimens collected from the College of Science and Engineering, Flinders University, Australia, pure culture of E. gracilis (length: 55.6 ± 4.63 μm, diameter: 8–10 μm) was established in 1 L medium containing 30 wheat grains, 25 rice grains and 5 g skim milk powder. After autoclaving at 121 °C for 5 min, the culture medium was stored at 4 °C. E. gracilis was inoculated into the culture medium at 10% (v/v) and cultured in 250 mL Erlenmeyer flasks at 24 °C in a temperature-controlled room under continuous light (70 mmol photons per m−2 s−1). The flask was stirred manually twice a day to prevent algal settlement.
For the study under controlled conditions, cultures of the E. gracilis were established in culture flasks with each working volume of 20 mL under 24 h cycle illumination (130–150 μmol m−2 s−1) at 25 °C. For all examinations, the stock culture of E. gracilis was grown in a modified Cramer–Myers medium (1.0 g L−1 of (NH4)2HPO4, 1.0 g L−1 of KH2PO4, 0.2 g L−1 of MgSO4·7H2O, 0.02 g L−1 of CaCl2·2H2O, 3 mg L−1 of FeSO4·7H2O, 1.8 mg L−1 of MnCl2·4H2O, 1.5 mg L−1 of CoSO4·7H2O, 0.4 mg L−1 of ZnSO4·7H2O, 0.2 mg L−1 of Na2MoO4·2H2O, 0.02 mg L−1 of CuSO4·5H2O, and 0.1 mg L−1 of thiamine hydrochloride (vitamin B1), and cyanocobalamin (vitamin B12) at pH 3.5).
The E. gracilis cells were counted using a haemocytometer (Improved Marienfeld Neubauer, Germany) to determine the cell density. The growth of E. gracilis was also measured at 780 nm using a spectrophotometer (LKB Biochrom 4050 Ultrospec II UV/vis). Because a linear co-relationship between OD780 and cell density has been reported (Supplementary S1, ESI†), OD780 was used to measure the growth in this work. For induction of lipid accumulation, E. gracilis cells were taken from the exponential growth phase and subsequently cultured for 10 days in five (5) different treatments.
To find the optimal conditions for stress-induced lipid biosynthesis in E. gracilis cells, five treatments were used to manipulate the availabilities of nutrients and light: (1) modified Cramer–Myers medium (MCM) (24 h, 70 mmol photons per m−2 s−1); (2) MCM, nitrogen (−) (24 h, 70 mmol photons per m−2 s−1); (3) MCM, nitrogen (−), calcium (−) (24 h, 70 mmol photons per m−2 s−1); (4) MCM, nitrogen (−), calcium (−), glucose (+) (24 h, 70 mmol photons per m−2 s−1), and (5) MCM, nitrogen (−), calcium (−), glucose (+) (darkness). Culture of the MCM was considered nutrient sufficient, whereas cells in other treatments were considered to be under stressed conditions.
2.2. Determination of autofluorescence properties of Euglena gracilis
Since photosynthetic organisms can biosynthesise various molecules with autofluorescence properties (e.g. different chlorophyll and accessory pigments), the autofluorescence properties of E. gracilis were determined at different excitation–emission wavelengths using a fluorescence spectrophotometer (Cary Eclipse, MY17180002) and a Zeiss LSM 880 Airyscan confocal microscope at Flinders Microscopy and Biomedical Services, Flinders University (Supplementary S2 and Supplementary Movie M1, ESI†). Autofluorescence of chlorophyll from different treatments and from heat-killed E. gracilis cells was detected upon excitation at 488 nm using a spectrophotometer. To ensure lethal results, microalgae were collected by centrifugation (2000g, 60 s) and re-suspended in a centrifuge tube with deionized water. Subsequently, the tube was set in a beaker, filled in with water, and placed in a heater. The samples were then kept at 100 °C for 2 min, and the solution containing the microalgae was allowed to cool to room temperature. The cells were then examined once every 2 h up to 24 h using a light microscope for any movement to confirm them to be dead.70
2.3. Lipid extraction and analysis of fatty acids
The total lipid was extracted using an adapted method of Bligh and Dyer.71 Briefly, from the growing E. gracilis culture, a sample volume was taken to provide approximately 500 mg of dry algal biomass. The algal culture was then centrifuged at 10
000 × g and 4 °C for 10 min. The supernatant was discarded and the pellet was washed 3 times with an equal volume of potassium phosphate buffer adjusted to the same pH as the culture medium. Subsequently, the pellet was re-suspended in deionized water and then transferred and weighed in a weighing dish. After that, the samples were dried at 60 °C for 48 h and stored at −20 °C for further use. Subsequently, approximately 50 mg of dried algal biomass was measured and was transferred to a mortar pre-washed with hexane. The weighing dish was also washed with a small amount (1 mL) of hexane using a Pasteur pipette in the fume hood in order to completely transfer the biomass to the mortar. Subsequently, the algal biomass was ground for 5 min into a fine, smooth paste using a pestle. In the case of evaporation of hexane during grinding, hexane was added to the mortar and the resulting slurry was mixed with the pestle until it was homogenised. After that, the hexane-cell mass mixture was centrifuged at 10
000 × g and 4 °C for 20 min, and the supernatant was isolated in a pre-weighed vial. This step was followed by the addition of 3 mL of hexane to the pellet and vigorous vortexing for 1 min and centrifuged at 4 °C for 30 min at 10
000 × g to ensure that all cell debris was fully settled. The extracted oil mass was then determined gravimetrically after complete evaporation of the hexane. All lipid extracts were then stored at −20 °C for further analyses. Quantitative analysis of fatty acids was undertaken using a PerkinElmer GC-MS (Clarus 500 and 560S) with internal standards. Crude lipids were extracted from the sample using solvents. The lipids were transmethylated and the profiles of different fatty acids were obtained by gas chromatography. The results were expressed as percentage of fatty acid methyl esters.72
2.4. Determination of fluorescent properties of DPAS in DMSO/water mixtures
DPAS was obtained from Hong Kong University of Science and Technology, China.68 The absorption and photoluminescence (PL) spectra of DPAS in DMSO/water mixtures were recorded using a fluorescence spectrophotometer (Cary Eclipse, MY17180002) with quartz cuvettes of 1 cm pathlength. The fluorescence spectra of 10 μM DPAS in DMSO (Sigma-Aldrich) and 40–90% water fractions (fw) were recorded (Supplementary S3, ESI†). An image of the aggregated DPAS in 2% commercial sunflower oil was taken using a Zeiss LSM 880 Airyscan confocal microscope at Flinders Microscopy and Biomedical Services with an excitation filter of 405 nm (2% laser power) and an emission filter of 560 nm at room temperature (Supplementary S3 and Supplementary Movie M2, ESI†).
2.5. Determination of algal growth in different DPAS concentrations
E. gracilis at a density of 6.0 ± 1.0 (×103) cells per mL (Abs780: 0.0145 ± 0.0033) were introduced to different concentrations (10, 50, and 100 μM) of DPAS in the CM medium and cultured under the previously described condition. The growth rate was determined by measuring the absorbance at 780 nm using a spectrometer at regular intervals up to 18 days. Algal cells treated with 100 μM of DPAS were observed using an optical microscope (Leica, USA) at different time intervals (0, 30, and 60 min).
2.6. Preparation of E. gracilis samples for BODIPY and DPAS staining
A stock solution of 1.0 mM BODIPY™ 505/515 (Thermo Fisher Scientific Inc.) was prepared in DMSO and stored in the darkness at −20 °C. Prior to use, the stock solution was diluted to 10 μM in DI water. For each sample, the final concentration of the DMSO was adjusted to 0.1%. Fluorometric quantification of the lipids was done according to the modified method of Cooper et al.73 Briefly, algal cells were cultured as noted earlier and adjusted to a density of 106 cells per mL. The cells were then centrifuged at 2000 × g for 60 s, and re-suspended in 200 μL DI water. Then, 200 μL of the prepared 10 μM BODIPY™ 505/515 solution was gently mixed with the cell suspension and incubated for 5
min in the darkness. Subsequently, the cells were washed three times with DI water by centrifugation at 2000 × g for 60
s and re-suspended in water. The stained cells were protected from light until further use.
For the study with DPAS, a stock solution of 1.0 mM DPAS in DMSO was prepared and stored in the darkness at 4 °C. The fluorescence intensities at different time intervals (10 min, 30 min, 60 min, and 120 min) were determined using a fluorescence spectrophotometer (Cary Eclipse, MY17180002) with quartz cuvettes of 1 cm pathlength. E. gracilis cells (105) under Treatment 5 were stained with 10 μM DPAS. For the fluorometric quantification of lipids in E. gracilis cells by confocal microscopy, the cells from different treatments were adjusted to a density of 106 cell per mL. Then, 10 μM DPAS was added and the samples were vortexed at 100 rpm for 30 s and then stored in the darkness for 30 min. The final concentration of the DMSO was adjusted to 0.1% for all the studies.
2.7. Imaging of Euglena gracilis using a confocal microscope
The E. gracilis cells were imaged using a Zeiss LSM 880 Airyscan confocal microscope and the ZEN 2.6 software (Carl Zeiss). The excitation wavelength for BODIPY and DPAS was 488 nm, and the emission wavelengths for BODIPY™ 505/515 and DPAS were 490–561 nm and 570–650 nm, respectively. Autofluorescence of the chlorophyll was detected with the excitation wavelength at 488 nm and emission wavelength of 685–758 nm.
2.8. Flow cytometric analysis of lipid content
Cytometric analysis was performed using a flow cytometer (model CytoFLEX Flow Cytometer, Beckman Coulter, Inc. USA). Euglena cells from different treatments were taken in three replicates and stained with 5.0 μM BODIPY™ 505/515 and 10 μM DPAS in accordance with the previously described methods. FITC channel (488 nm laser) was used to detect the fluorescence from BODIPY™ 505/515 and chlorophyll, and a Violet 610 (405 nm laser) was used to detect the fluorescence from the DPAS. The mean fluorescence intensity from a minimum of 10
000 cells per sample was acquired. Relative fluorescence was determined using the population cell percentile, with Treatment 1 serving as the control.
2.9. Data analysis
Data from the confocal microscopy was analysed using ImageJ 1.52a.74 The raw image was exported as “tiff” format in ImageJ, and the background of image from the fluorescence channel was calculated and subtracted using “Sliding paraboloid”. Subsequently, “Area”, “Mean grey value”, “Integrated density” and “Area fraction” were measured highlighting the image. Fluorescence intensity per cell from the total cells was then determined by subtracting the background IntDen value from the IntDen value of samples.
The flow cytometry data were analysed using the CytExpert v2.4 software and presented as histogram overlays. To detect BODIPY™ 505/515 labelled cells and chlorophyll, samples were gated on FITC-A vs. SSC-A and for DPAS, samples were gated on Violet610-A vs. SSC-A. The data of confocal microscopy and flow cytometry were exported to prepare graphs using Excel 2010. The means and medians of different treatments were compared by the relative fluorescence intensity of the gated population, with Treatment 1 set as the control group. The SPSS statistics software (version 23) was used to calculate the differences between Treatment 1 and other treatments at P < 0.05 level by the paired sample t-test.
3. Results
3.1. Screening algal growth conditions for different treatments
It was clear that the N and calcium availability regulated the growth of E. gracilis. Slower growth rates in all the treatments were observed except in the CM medium (Fig. 1a and b). After 10 days of the initial culture, cells in the CM medium grew rapidly, but showed slow growth under all stressed conditions from Treatment 2 to Treatment 5 with the slowest growth in Treatment 2, where nitrogen was deficient (Fig. 1a). Therefore, considering that the nitrogen could be completely utilised by the growing cells after 7 days,75 cells were cultured in the CM medium and transferred to the different treatment groups from the exponential phase, where almost identical growth patterns were observed (Fig. 1b). Except for the CM medium, after 7 days, algal growth in all the treatments showed a negative trend, with the dark condition in Treatment 5 showing the lowest value of OD780: 0.317 of algal growth after 10 days of continuous culture compared to other treatments. The oxygen concentration was lowest (5.07 ± 0.5 mg L−1) under the dark condition of Treatment 5 and it was highest (8.19 ± 0.12 mg L−1) in Treatment 1 after 10 days of consecutive culture. Over the culture time period, the oxygen concentration in the treatments with light conditions remained almost unchanged, which was due to the photosynthetic activities of algae (Fig. 1c).
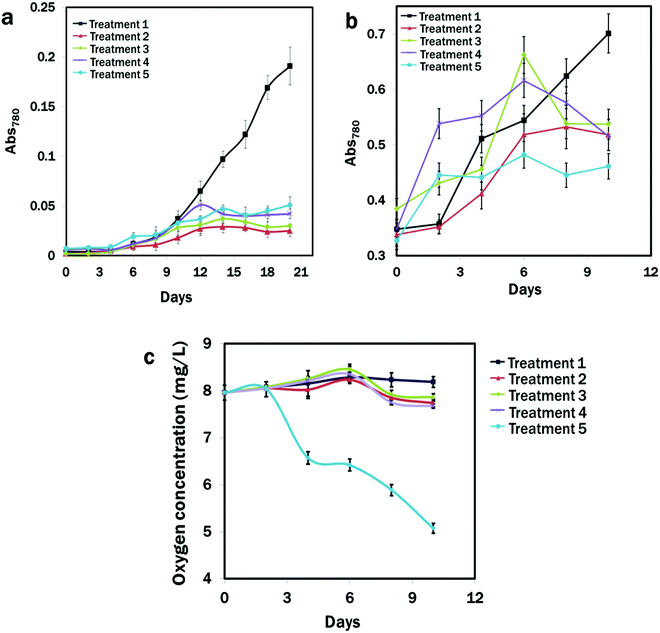 |
| Fig. 1 Growth of Euglena gracilis and changes in oxygen concentration in the medium at different time intervals. (a) Initial culture started at the Abs780 measure of 0.004 ± 0.002. (b) Culture for lipid induction began at the Abs780 measure of 0.349 ± 0.021. (c) Changes in oxygen concentration in different treatments. Treatment 1: modified Cramer–Myers medium (MCM); Treatment 2: MCM, (−) N2; Treatment 3: MCM, (−) N2, (−) Ca2+; Treatment 4: MCM, (−) N2, (−) Ca2+, (+) glucose (24 h light); and Treatment 5: MCM, (−) N2, (−) Ca2+, (+) glucose (24 h darkness) conditions. | |
3.2. Effects of DPAS on E. gracilis growth
We used lipid-specific DPAS to check its efficacy in algae with different cell membrane structure from that of the animal cell model.68 Prior to the introduction to the cells for lipid analysis, the effects of DPAS on growth were monitored. The conditions of the cells were also confirmed under a light microscope immediately after exposure to DPAS. In comparisons with the control group, no difference was observed in the growth pattern of algae even after exposure to the significantly higher concentration of 100 μM DPAS (Fig. 2a). Images from the light microscope (Fig. 2b–d) revealed that, immediately after exposure, movements of the cells reduced and the size decreased by 45% (Fig. 2b), but within 30 min, the cells had completely recuperated (Fig. 2d) and were fully functional. The movement of E. gracilis after the incorporation of DPAS molecules into the lipid drops also confirmed that cells were alive and functional (Movie M3, ESI†), suggesting that DPAS was not toxic for the algal cells.
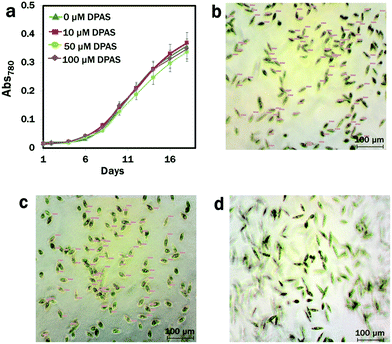 |
| Fig. 2 Effects of DPAS on E. gracilis. Growth of E. gracilis exposed to different concentrations (0–100 μM) of DPAS (a). Changes in E. gracilis size and movement at different time intervals due to the exposure to 100 μM DPAS: (b) 26.32 ± 4.74 μm (0 min after incubation); (c) 31.13 ± 4.60 μm (10 min after incubation); and (d) fully recovered and motile, 48.1 ± 6.27 μm (30 min after incubation). | |
3.3. Labelling of stress-induced lipid droplets by DPAS in E. gracilis
Nutrient starvation is a well-known condition with lipid induction in microalgae.17,76 In this experiment, the effects of nitrogen and calcium starvation on lipid accumulation in E. gracilis cells were studied. In addition to nitrogen and calcium starvation, glucose-supplemented light and dark conditions were also analysed. As an approach to rapid scanning for lipid accumulation, a simple staining procedure with minimal sample preparation was implemented on E. gracilis cells with DPAS directly added to the growing media in different nutrient treatment groups (S4, ESI†). As the control, the lipophilic green fluorescent dye BODIPY™ 505/515 was used to co-stain the cells for lipid detection in microalgae.50,77 From the images of different treatments, lipid accumulation was visible from the nutrient-starved conditions (Fig. 3B–E). However, cells from Treatment 5 (MCM, (−) N2, (−) Ca2+, (+) glucose (24 h darkness) conditions) showed the highest lipid accumulation (Fig. 3E and F), followed by those in Treatment 4 (MCM, −N2, −Ca2+, +glucose, Fig. 3D and F) and Treatment 2 (MCM, −N2, Fig. 3B and F). The lowest level of fluorescence occurred in the control group (Treatment 1: MCM medium in 24 h light condition). Autofluorescence from the chlorophyll detected was the lowest in Treatment 5 (Fig. 3E and F). It was clear that DPAS and BODIPY™ 505/515 signals were co-localised completely, and no interference from the red channel for chlorophyll was observed in confocal microscopy. ImageJ analysis of relative fluorescence per cell showed an almost identical pattern to DPAS and BODIPY™ 505/515 for lipid labelling (Fig. 3F).
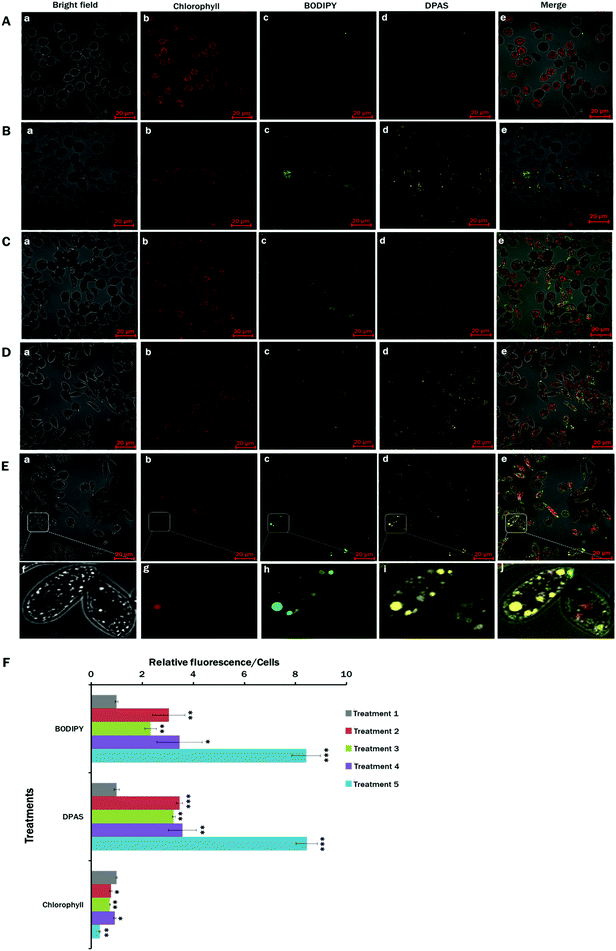 |
| Fig. 3 Images of E. gracilis cells incubated with lipid-specific AIE nanoprobe, DPAS (C20H16N2O) and commercial lipid-specific fluorescent probe, BODIPY. Cells were cultured in (A) Treatment 1: modified Cramer–Myers medium (MCM); (B) Treatment 2: MCM, (−) N2; (C) Treatment 3: MCM, (−) N2, (−) Ca2+; (D) Treatment 4: MCM, (−) N2, (−) Ca2+, (+) glucose (24 h light); (E) Treatment 5: MCM, (−) N2, (−) Ca2+, (+) glucose (24 h darkness) conditions. (Bright-field image: (a) fluorescence images – chlorophyll: (b) BODIPY: (c) and DPAS: (d) merged images: (e) and the enlarged regions of E ((f–j) for bright-field, reduced chlorophyll, BODIPY™ 505/515, DPAS stained cells and merged image, respectively). (F) Relative fluorescence intensity/cell for different treatments. Values are relative to the control condition (Treatment 1: modified Cramer–Myers medium (MCM)). Averages shown as mean ± SE; *P < 0.05; **P < 0.01. Images were taken using a Zeiss LSM 880 Airyscan confocal microscope. | |
The results of the present study showed that DPAS had good lipid specificity and penetration abilities to E. gracilis with higher sensitivity, which were very similar to those of the commercially available BODIPY™ 505/515 dye. Moreover, due to the photostable properties of AIE molecules, wash free78 and easy sample preparation techniques (Supplementary S4, ESI†), DPAS had good potential for rapid visualisation and quick screening of lipid content in the cells from different treatments.
3.4. Flow cytometric analysis of lipid contents
The plots of the flow cytometry experiments reveal that BODIPY™ 505/515 (Fig. 4a–e) and DPAS (Fig. 4f–j) could successfully label the lipid in E. gracilis cells. In comparison to the MCM medium under 24 h light condition (Treatment 1) (Fig. 4a and f), cytograms of BODIPY fluorescence vs. side scatter (Fig. 4b–e) and DPAS fluorescence vs. side scatter (Fig. 4g–j) showed more lipid accumulation in cells cultured in the nutrient starved cells. Maximum lipid accumulation is observed in Treatment 5 (MCM, (−) N2, (−) Ca2+, (+) glucose (24 h darkness) conditions) (Fig. 4e and j), followed by Treatment 4 (MCM, (−) N2, (−) Ca2+, (+) glucose) (Fig. 4d and i).
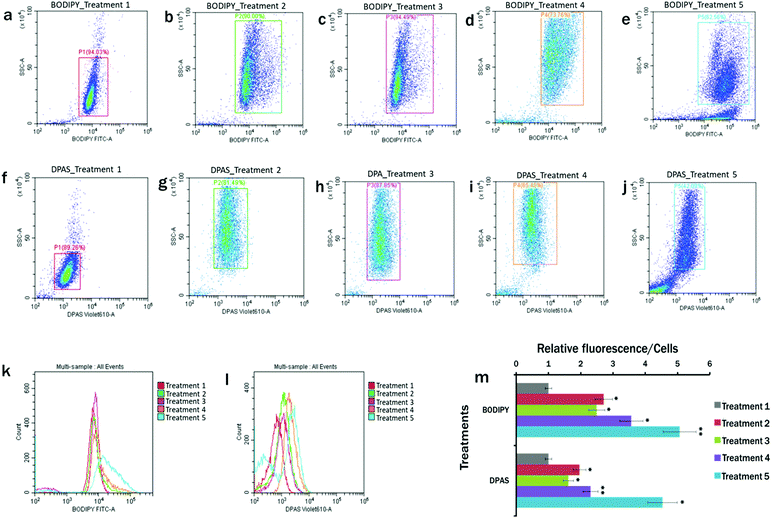 |
| Fig. 4 Flow cytometry measurements for lipid in Euglena gracilis cells in different treatments labelled with BODIPY™ 505/515 and AIE probe, DPAS. (a–e) Flow cytogram of FITC-A vs. SSC-A for BODIPY fluorescence in different treatments; (f–j) flow cytogram of Violet610-A vs. SSC-A for DPAS fluorescence. Cells were cultured in Treatment 1: modified Cramer–Myers medium (MCM); Treatment 2: MCM, (−) N2; Treatment 3: MCM, (−) N2, (−) Ca2+; Treatment 4: MCM, (−) N2, (−) Ca2+, (+) glucose (24 h light); and Treatment 5: MCM, (−) N2, (−) Ca2+, (+) glucose (24 h darkness) conditions. (k) Histogram of BODIPY fluorescence, and (l) histogram of BODIPY™ 505/515 fluorescence for cells. (m) Relative fluorescence of BODIPY™ 505/515 and DPAS/cell for different treatments. Values are relative to the control condition (Treatment 1: modified Cramer–Myers medium (MCM)). Averages shown as mean ± SE; *P < 0.05; **P < 0.01. All plots are in logarithmic scale for both axes. | |
Moreover, as lipid accumulation in algae has been reported to produce obese phenotypes,79,80 cellular events in nutrient starved population with greater side scatter in this experiment were proportional to the cellular obesity and accumulation of probes within the lipid droplets. Therefore, histograms with increased fluorescence from BODIPY™ 505/515 and DPAS were consistent with obese cell types having higher relative lipid fluorescence per cell (Fig. 4k–m).
3.5. Fatty acid analysis
The maximum amount of TFA (∼19.03% of DW) content was found from E. gracilis cells cultured in Treatment 5 (MCM, (−) N2, (−) Ca2+, (+) glucose (24 h darkness) conditions). TFA contents in Treatment 2 (MCM, (−) N2) and Treatment 4 (MCM, (−) N2, (−) Ca2+, (+) glucose) were almost identical: ∼14.06% and ∼14.5% of DW, respectively, which was also significantly (P < 0.05) higher than in the control group (Treatment 1: MCM medium) that contained ∼7.1% TFA of DW. However, the difference between Treatment 3 (MCM, (−) N2, (−) Ca2+) and Treatment 1 was not significant (Fig. 5).
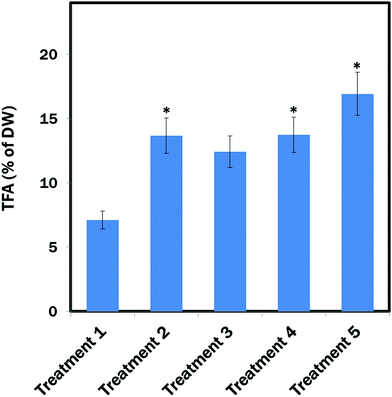 |
| Fig. 5 TFA contents in different treatments. Treatment 1: modified Cramer–Myers medium (MCM); Treatment 2: MCM, (−) N2; Treatment 3: MCM, (−) N2, (−) Ca2+; Treatment 4: MCM, (−) N2, (−) Ca2+, (+) glucose (24 h light); and Treatment 5: MCM, (−) N2, (−) Ca2+, (+) glucose (24 h darkness) conditions. Data are presented as mean ± SE, n = 3, * statistically significant (P < 0.05). | |
The percentage FA of FAMEs (Table 1) revealed a high content of long-chain polyunsaturated fatty acids (LC-PUFAs: C20–C22) of ∼30.66% in Treatment 5, which was followed by the Treatment 4 with ∼23.22% of LC-PUFAs. The lowest amount of LC-PUFAs of ∼16.30% was found in Treatment 1. Monounsaturated fatty acid (MUFA: C14:1–C20:1) content was found to be almost identical in all the treatments. Among the polyunsaturated fatty acids (PUFAs), α-linolenic acid (ALA) and linoleic acid (LA) were the most abundant in Treatments 1, 2, 3 and 4, whereas supplementation of glucose under a dark condition reduced their biosynthesis compared to other treatments. Among the MUFAs and SAFAs, the most abundant in all the treatments were palmitoleic acid (16:1n-7) and palmitic acid (C16:0), which are the precursors to longer fatty acids. The maximum amount of omega-3 fatty acids eicosapentaenoic acid (EPA) and docosahexaenoic acid (DHA) occurred in Treatment 5.
Table 1 Percentage of FAMEs in Euglena gracilis cells cultured under different conditions
Percentage of FAMEs |
Treatments |
Treatment 1 |
Treatment 2 |
Treatment 3 |
Treatment 4 |
Treatment 5 |
None detectable.
|
C12:0 |
0.19 ± 0.05 |
0.27 ± 0.01 |
0.24 ± 0.01 |
0.59 ± 0.39 |
1.28 ± 1.15 |
C13:0 |
0.28 ± 0.01 |
0.37 ± 0.03 |
0.36 ± 0.08 |
1.36 ± 1.19 |
3.63 ± 2.78 |
C14:0 |
1.85 ± 0.12 |
2.41 ± 0.18 |
2.31 ± 0.14 |
5.10 ± 3.30 |
4.93 ± 2.95 |
C15:0 |
0.44 ± 0.26 |
0.64 ± 0.41 |
0.63 ± 0.31 |
1.07 ± 0.97 |
1.27 ± 0.93 |
C16:0 |
10.87 ± 0.64 |
9.80 ± 0.14 |
10.15 ± 0.66 |
12.28 ± 4.13 |
13.51 ± 1.76 |
C16:1 N-7 |
15.18 ± 2.33 |
13.94 ± 0.54 |
13.87 ± 1.06 |
11.19 ± 0.82 |
12.85 ± 0.38 |
C17:0 |
0.105 ± 0.05 |
0.22 ± 0.09 |
0.23 ± 0.07 |
0.28 ± 0.12 |
0.82 ± 0.35 |
C17:1 N-7 |
4.38 ± 0.24 |
4.03 ± 0.58 |
4.01 ± 0.60 |
3.33 ± 1.11 |
4.15 ± 3.06 |
C18:0 |
1.12 ± 0.44 |
1.08 ± 0.03 |
1.09 ± 0.01 |
1.53 ± 0.12 |
2.52 ± 0.09 |
C18:1T N-9 |
1.71 ± 0.15 |
1.83 ± 0.01 |
1.82 ± 0.07 |
2.46 ± 0.66 |
3.14 ± 0.25 |
C18:2 TT N-6,9 |
22.48 ± 1.55 |
23.18 ± 4.06 |
22.52 ± 3.97 |
20.63 ± 7.51 |
13.68 ± 7.59 |
C18:3 N-3,6,9 (ALA) |
25.06 ± 3.53 |
23.54 ± 0.81 |
23.14 ± 0.07 |
16.91 ± 7.39 |
10.99 ± 7.16 |
C20:2 N-6,9 |
1.92 ± 1.20 |
2.76 ± 0.56 |
2.89 ± 0.55 |
3.92 ± 1.80 |
4.26 ± 1.92 |
C20:3 N-6,9,12 |
0.62 ± 0.88 |
—a |
1.08 ± 0.01 |
0.93 ± 0.14 |
0.45 ± 0.18 |
C20:4 N-6,9,12,15 |
3.0 ± 0.76 |
3.31 ± 0.17 |
3.37 ± 0.23 |
3.85 ± 0.37 |
4.71 ± 0.45 |
C20:3 N-3,6,9 (ETE) |
1.38 ± 0.10 |
1.92 ± 0.02 |
1.9 ± 0.08 |
2.23 ± 0.21 |
3.59 ± 2.93 |
C20:5 N-3,6,9,12,15 (EPA) |
5.95 ± 0.73 |
5.85 ± 1.52 |
5.81 ± 1.52 |
6.94 ± 2.54 |
10.18 ± 5.67 |
C22:1 N-9 |
0.65 ± 0.30 |
1.58 ± 0.66 |
0.91 ± 0.15 |
1.41 ± 0.25 |
2.01 ± 0.71 |
C22:5 N-6,9,12,15,18 |
0.99 ± 0.81 |
0.99 ± 0.71 |
1.36 ± 0.29 |
1.49 ± 0.18 |
2.18 ± 0.34 |
C22:6 N-3,6,9,12,15,18 (DHA) |
1.77 ± 0.23 |
2.25 ± 0.95 |
2.22 ± 0.84 |
2.45 ± 1.12 |
3.26 ± 1.83 |
SAFAs |
14.88 ± 3.91 |
14.80 ± 3.47 |
15.02 ± 3.60 |
22.22 ± 4.32 |
27.98 ± 4.44 |
MUFAs |
21.27 ± 7.13 |
19.80 ± 6.45 |
19.70 ± 6.42 |
16.98 ± 4.81 |
20.14 ± 5.34 |
PUFAs |
47.54 ± 1.82 |
46.72 ± 0.25 |
45.66 ± 0.43 |
37.55 ± 2.63 |
24.67 ± 1.89 |
LC-PUFAs |
16.30 ± 1.76 |
18.68 ± 1.75 |
19.56 ± 1.61 |
23.22 ± 1.96 |
30.66 ± 2.90 |
4. Discussion
To date, stressed conditions of deficiency of different nutrients, notably nitrogen, phosphorus, sulphur, potassium, and iron have been studied for lipid production in different microalgal species.81–83 In this study, alongside the nitrogen stress, we investigated the impact of the combination of nitrogen and calcium deprivation on lipid production. Moreover, as a supplementary carbon source we used glucose under 24 h light and 24 h darkness conditions. Interestingly, the maximum lipid production was observed in the nitrogen- and calcium-deprived and the glucose-supplemented dark condition.
During nitrogen starvation, biosynthesis of lipid droplets occurred due to the increased TAG formation from acyl-CoA, recycling of acyl moieties from degraded membrane lipid products, and increased carbon flux towards the source compounds (e.g. glycerol-3-phosphate and acyl-CoA) for fatty acid synthesis.80,84–86 Supplementation of organic carbon such as glucose to the nitrogen-starved growing media could alter the carbon fluxes towards the biosynthesis of necessary precursors of lipid droplets, which are generally synthesised during photosynthesis using atmospheric carbon sources (CO2).87 The block of starch biosynthesis could be an effective way to enhance TAG synthesis by diverting carbon flux.88,89 In the present study, reduction of chlorophyll under the dark condition reduced the starch biosynthesis in the chloroplast, and glucose supplement supported the biosynthesis of glycerate-3-phosphate and glyceraldehyde-3-phosphate as the precursor molecules of pyruvate and acetyl-CoA through altered carbon flux, resulting in an increase in lipid production. Under the nutrient-starved light conditions, blockage of the starch biosynthesis in the chlorophyll might possibly not occur, and under the glucose-supplemented light condition, the availability of additional organic carbon might favour the redirection of the carbon flux, but still starch biosynthesis occurred in the chloroplasts. Therefore, despite the accumulation of lipids, it did not reach the same level as under the dark condition.
Furthermore, a recent report of the model of photosynthetic organisms suggests that in the presence of light, chloroplasts take up Ca2+ from the cytosol and store it, while transitioning to the dark condition induces the chlorophyll to release the stored Ca2+ back into the cytosol.90 Additionally, during nitrogen starvation, carbon from the photosynthesis system was channelled into storage molecules such as starch or lipids,25 which is supposed to require excess energy and adequate signalling functions. Generally, two photosystems, photosystem-I (PS-I) and photosystem-II (PS-II), are involved to achieve the linear electron flow (LEF) and cyclic electron flow (CEF) that create transmembrane proton (H+) gradient and reduce power (NADPH), and consequently, generate ATP.91,92 These ATPs are utilised in the Krebs cycle, glyoxylate cycle and in the mitochondrion for respiratory oxidative phosphorylation. During the process of lipid biosynthesis under N deficiency, excess ATPs are also required by the system, whereby ATP production through CEF has been reported to be elevated to compensate for the reduction of ATP from PS-II through LEF due to the limited CO2 fixation activities.93–95 However, CEF is a Ca2+-dependent process, responding appropriately through the combined actions of Ca2+ sensor calmodulin (CaM) and chloroplast-localized Ca2+ sensor (CAS) protein.96–98 The chlorophyll reduction observed in the present study under a dark condition could be the result of the phosphorylation of the photosynthetic and thylakoid protein complexes that could alter the proton (H+) gradient and Ca2+-buffering mechanism in the chloroplast,99–101 consequently increasing the Ca2+ concentration in the cytosol of E. gracilis. As a result, the generated Ca2+ level under the dark condition was conceivably adequate to successfully run the cellular signalling for ATP production through CEF, which was definitely not the case for the calcium-starved and light conditions.
However, despite the reported positive correlations between carbon flux redirection and lipid biosynthesis, substantial differences have been found in lipid accumulation under the same condition even for the same species, suggesting the complexities of controlling lipid biosynthesis mechanisms.102,103 Therefore, a reliable and convenient tool is critical for easy detection and visualisation of lipid droplets in algae during culture.
In this study, we used a novel and easy strategy to visualise lipid droplets in E. gracilis by adopting a lipid-specific AIE fluorophore, DPAS. The emission of DPAS increased rapidly with the addition of water from 80% to 90% in DMSO, which was due to the insoluble properties of AIE molecules and formation of nanoaggregates in the aqueous media.104 Fluorescent intensity was found to be almost identical and stable for the samples after 2 h of staining, as supported by the results of a previous study, where DPAS was stable in continuous scanning with 2% laser power energy.68
The results of the confocal microscopy and flow cytometry in the present study suggest a good working condition of DPAS for lipid labelling in the Euglena cells, which are similar to the commercially available BODIPY™ 505/515 dye. However, given the priority of BODIPY in visualisation of lipid droplets over other tractional lipid-specific probes,105 some studies suggest that inappropriate outcomes might result from disproportionate binding, unselective labelling of the mitochondria, and background signals in plasma and nuclear membranes.54,67,106
The hydrophobic nature of lipid droplets and the absence of strong interactions with other organelles allow hydrophobic probes to accumulate in lipid droplets. The specific structure with the partially negatively charged oxygen atoms attached to the electron acceptor in the carbonyl group can possibly make lipid-specific AIE probes hydrophobic.107 Additionally, the cheap raw materials of DPAS synthesis fluorenone, benzophenone and salicylaldehyde are also hydrophobic, which might cause the hydrophobicity of DPAS and allow this nanofluorophore to strongly target and interact with intercellular hydrophobic lipid droplets.68
The E. gracilis has a unique complex cell membrane structure that is composed of glucose, galactose, mannose, fucose, xylose, and rhamnose. Therefore, it appears that penetration of the AIE fluorophore DPAS is not hindered by the cellular structure of this algal species. Once DPAS has been internalised by the cells, the restriction of the intramolecular motions and activation of the excited state intramolecular proton transfer process can probably turn the fluorescence on this AIE probe and allow the lipid droplets to become visible.108 Furthermore, because the DPAS did not interfere with the red channel of chlorophyll autofluorescence or the green channel of BODIPY dye, it is possible that this lipid-specific AIE fluorophore might be an appropriate multicolour visualising tool for microalgae.
This study also suggests a relatively easy application of DPAS compared to the BODIPY dye, since a much higher concentration of 100 μM DPAS showed no negative impact on the growth or behaviour of Euglena cells or on the fluorescence intensity. The sample preparation was also relatively easy, with fewer steps involved (Supplementary S4, ESI†), which is time-efficient and less stressful for the living organism. This might allow study of other physiological factors within the cell in a natural condition. In contrast, the use of commercial lipid-specific probes, regardless of BODIPY and Nile Red, requires extreme caution for dye adjustment, since lower concentrations can cause photobleaching problems, and a slightly higher concentration or lower-level competencies during washing steps might result in marked background signals and ineffective lipid studies. Due to the AIE properties of DPAS, this dye is free from those limitations, and upon aggregation, strong fluorescence of this probe clearly distinguish lipid droplets from the background. Moreover, from a previous study,68 it has already been proved that within 5 min of irradiation with 2% laser power energy under a confocal microscope, BODIPY could lose 90% of the fluorescence signals, supposedly through self-decomposition, whereas DPAS showed almost no change in fluorescence intensity even after 60 min of continuous scanning with 2% laser power energy, which is far superior to any other commercially available lipid-specific fluorophores. Additionally, DPAS could label the lipid within 10 min in the organism, indicating that the flexible molecular structure of this probe might enable it to quickly adjust its conformation as per the penetration requirement of the Euglena cell membrane or transport receptor structures.
The fatty acid content and composition in algae depend entirely on the species and cultivation conditions. E. gracilis is a known producer of different biofunctional components with several health benefits.109–113 However, since the composition of FAMEs in microalgae can be affected by many factors, analysis of time point and culture condition is crucial to obtain desired biofunctional compounds from this organism.114,115 In this study, the fatty acids profile revealed much high contents of LC-PUFAs in Treatments 4 and 5 compared to that of the Treatment 1, while MUFAs content was almost identical in all the treatments. LC-PUFAs, specifically DHA and EPA, could have significant physiological and therapeutic functions for human well-being. Desaturase and elongase enzymes that biosynthesize EPA and DHA from LA and ALA are scarce in human, and so the conversion rate, which also starts to deteriorate with age and under certain disease conditions.6,116–119 Therefore, dietary supplementation of LC-PUFAs could aid human by cutting down the risk-factors of cancer, and cardiovascular and neurodegenerative diseases through balancing the healthy ω-6/ω-3 ratio.116,120–122 ARA (C20:4) and EPA as the building blocks of distinguished prostaglandins, leukotrienes, tromboxanes, and lipoxins play essential roles in different bio-physiological processes and can be utilised to treat conditions like atopic diseases and asthma.123–125 Moreover, inverse correlations with obesity, diabetes and hepatosteatosis with consumption of palmitoleic acid have also been reported in some studies,126,127 while dietary supplementation of polyunsaturated omega-6 fatty acid and linoleic acid can also prevent hair loss and assist with wound healing properties.128,129 Additionally, the necessity of α-linolenic acid for dietary supplementation is crucial since it could improve the biosynthesis of beneficiary long-chain PUFAs as humans are unable to de novo synthesize α-linolenic acid from stearic acid due to the absence of the 12- and 15-desaturase enzymes.130,131 DHA can also support multiplexed cell membrane and cell signalling, particularly in the brain and retina, adequate supplementation of which boosts visual activities and immunity and improves cognitive functions in humans.132–135 Since all these fatty acids were available in large proportions in the treatments, and DHA and EPA in Treatment 5, the results of the present study also suggest that promising biofunctional compounds might be available from culturing E. gracilis under applied conditions.
5. Conclusion
In this study, we identified the optimal environmental condition for lipid production in Euglena gracilis as a source of health beneficiary metabolites. The LC-PUFA content was found at the highest level in E. gracilis under nitrogen and calcium deprivation and glucose supplementation in darkness. E. gracilis could be exploited to produce healthy food supplement for humans. We also developed a novel protocol by using an AIE bioprobe for the rapid and easy detection of lipid drops in E. gracilis, which is quick and convenient for high contrast visualisation under an in situ condition. The probe was biocompatible and could easily penetrate the Euglena cells, suggesting the possibility of using the AIE bioprobe to detect oil drops in other species of microalgae. The AIE probe DPAS is able to localize lipid droplets accurately, demonstrating the DPAS probe having a better performance than the traditional lipid-specific dye BODIPY. As the DPAS probe is free of the drawback of aggregation-caused quenching, the novel AIE probe can remove the interference of background noise during confocal microscopy imaging. Therefore, the DPAS probe is recommend for screening the capacity of lipid droplet production in algal strains that have the potential as a source to produce healthy food for human.
Conflicts of interest
The authors declare that they have no conflict of interest.
Acknowledgements
AHM Mohsinul Reza is grateful for the financial support of Australian Government Research Training Program Scholarship (AGRTPS) (International) for his PhD study at Flinders University. AHM Mohsinul Reza is also thankful to Mr Philip Michelsen, College of Science and Engineering, Flinders University, for his technical support during algae culture. The authors acknowledge the expertise, equipment, and support provided by Microscopy Australia and the Australian National Fabrication Facility at the South Australian nodes under the National Collaborative Research Infrastructure Strategy for their great support.
References
- World Population Prospects 2019, https://population.un.org/wpp/Graphs/Probabilistic/POP/TOT/900, (accessed August 2020).
- C. S. Jones and S. P. Mayfield, Algae biofuels: versatility for the future of bioenergy, Curr. Opin. Biotechnol, 2012, 23, 346–351 CrossRef CAS.
- A. P. Singh and S. I. Olsen, A critical review of biochemical conversion, sustainability and life cycle assessment of algal biofuels, Appl. Energy, 2011, 88, 3548–3555 CrossRef CAS.
- C. Galasso, A. Gentile, I. Orefice, A. Ianora, A. Bruno, D. M. Noonan, C. Sansone, A. Albini and C. Brunet, Microalgal Derivatives as Potential Nutraceutical and Food Supplements for Human Health: A Focus on Cancer Prevention and Interception, Nutrients, 2019, 11, 1226 CrossRef CAS.
- E. B. Rimm, L. J. Appel, S. E. Chiuve, L. Djoussé, M. B. Engler, P. M. Kris-Etherton, D. Mozaffarian, D. S. Siscovick, A. H. Lichtenstein and American Heart Association Nutrition Committee of the Council on Lifestyle and Cardiometabolic Health, Council on Epidemiology and Prevention, Council on Cardiovascular Disease in the Young, Council on Cardiovascular and Stroke Nursing; and Council on Clinical Cardiology, Seafood Long-Chain n-3 Polyunsaturated Fatty Acids and Cardiovascular Disease: A Science Advisory From the American Heart Association, Circulation, 2018, 138, e35–e47 CrossRef CAS.
- I. Khozin-Goldberg, U. Iskandarov and Z. Cohen, LC-PUFA from photosynthetic microalgae: occurrence, biosynthesis, and prospects in biotechnology, Appl. Microbiol. Biotechnol., 2011, 91, 905–915 CrossRef CAS.
- M. Venegas-Calerón, O. Sayanova and J. A. Napier, An alternative to fish oils: Metabolic engineering of oil-seed crops to produce omega-3 long chain polyunsaturated fatty acids, Prog. Lipid Res., 2010, 49, 108–119 CrossRef.
- M. J. Szul, S. P. Dearth, S. R. Campagna and E. R. Zinser, Carbon Fate and Flux in Prochlorococcus under Nitrogen Limitation, mSystems, 2019, 4, e00254 CAS.
- J. Kim, C. M. Brown, M. K. Kim, E. H. Burrows, S. Bach, D. S. Lun and P. G. Falkowski, Effect of cell cycle arrest on intermediate metabolism in the marine diatom Phaeodactylum tricornutum, Proc. Natl. Acad. Sci. U. S. A., 2017, 114, E8007–E8016 CrossRef CAS.
- X. Wang, H. K. Fosse, K. Li, M. S. Chauton, O. Vadstein and K. I. Reitan, Influence of nitrogen limitation on lipid accumulation and EPA and DHA content in four marine microalgae for possible use in aquafeed, Front. Mar. Sci., 2019, 6, 95 CrossRef.
- J. H. Janssen, R. H. Wijffels and M. J. Barbosa, Lipid production in Nannochloropsis gaditana during nitrogen starvation, Biology, 2019, 8, 5 CrossRef CAS.
- E. C. Goncalves, J. Koh, N. Zhu, M. J. Yoo, S. Chen, T. Matsuo, J. V. Johnson and B. Rathinasabapathi, Nitrogen starvation-induced accumulation of triacylglycerol in the green algae: evidence for a role for ROC40, a transcription factor involved in circadian rhythm, Plant J., 2016, 85, 743–757 CrossRef CAS.
- F. Yang, W. Xiang, T. Li and L. Long, Transcriptome analysis for phosphorus starvation-induced lipid accumulation in Scenedesmus sp, Sci. Rep., 2018, 8, 16420 CrossRef.
- A. Sato, R. Matsumura, N. Hoshino, M. Tsuzuki and N. Sato, Responsibility of regulatory gene expression and repressed protein synthesis for triacylglycerol accumulation on sulfur-starvation in Chlamydomonas reinhardtii, Front. Plant Sci., 2014, 5, 444 Search PubMed.
- P. C. Gorain, S. K. Bagchi and N. Mallick, Effects of calcium, magnesium and sodium chloride in enhancing lipid accumulation in two green microalgae, Environ. Technol., 2013, 34, 1887–1894 CrossRef CAS.
- H. Chen, Y. Zhang, C. He and Q. Wang, Ca2+ signal transduction related to neutral lipid synthesis in an oil-producing green alga Chlorella sp. C2, Plant Cell Physiol., 2014, 55, 634–644 CrossRef CAS.
- X. M. Sun, L. J. Ren, Q. Y. Zhao, X. J. Ji and H. Huang, Microalgae for the production of lipid and carotenoids: a review with focus on stress regulation and adaptation, Biotechnol. Biofuels, 2018, 11, 272 CrossRef CAS.
- M. V. Rohit and S. Venkata Mohan, Quantum yield and fatty acid profile variations with nutritional mode during microalgae cultivation, Front. Bioeng. Biotechnol., 2018, 6, 111 CrossRef CAS.
- O. Perez-Garcia, F. M. Escalante, L. E. de-Bashan and Y. Bashan, Heterotrophic cultures of microalgae: metabolism and potential products, Water Res., 2011, 45, 11–36 CrossRef CAS.
- J. Krajčovič, M. Vesteg and S. D. Schwartzbach, Euglenoid flagellates: a multifaceted biotechnology platform, J. Biotechnol., 2015, 202, 135–145 CrossRef.
- J. S. Rodríguez-Zavala, M. A. Ortiz-Cruz, G. Mendoza-Hernández and R. Moreno-Sánchez, Increased synthesis of α-tocopherol, paramylon and tyrosine by Euglena gracilis under conditions of high biomass production, J. Appl. Microbiol., 2010, 109, 2160–2172 CrossRef.
- X. Luo, P. Su and W. Zhang, Advances in microalgae-derived phytosterols for functional food and pharmaceutical applications, Mar. Drugs, 2015, 13, 4231–4254 CrossRef CAS.
- P. Grimm, J. M. Risse, D. Cholewa, J. M. Müller, U. Beshay, K. Friehs and E. Flaschel, Applicability of Euglena gracilis for biorefineries demonstrated by the production of α-tocopherol and paramylon followed by anaerobic digestion, J. Biotechnol., 2015, 215, 72–79 CrossRef CAS.
- Q. Hu, M. Sommerfeld, E. Jarvis, M. Ghirardi, M. Posewitz, M. Seibert and A. Darzins, Microalgal triacylglycerols as feedstocks for biofuel production: perspectives and advances, Plant J., 2008, 54, 621–639 CrossRef CAS.
- S. A. Scott, M. P. Davey, J. S. Dennis, I. Horst, C. J. Howe, D. J. Lea-Smith and A. G. Smith, Biodiesel from algae: challenges and prospects, Curr. Opin. Biotechnol, 2010, 21, 277–286 CrossRef CAS.
- T. Tomiyama, K. Goto, Y. Tanaka, T. Maruta, T. Ogawa, Y. Sawa, T. Ito and T. Ishikawa, A major isoform of mitochondrial trans-2-enoyl-CoA reductase is dispensable for wax ester production in Euglena gracilis under anaerobic conditions, PLoS One, 2019, 14, e0210755 CrossRef CAS.
- T. Tomiyama, K. Kurihara, T. Ogawa, T. Maruta, T. Ogawa, D. Ohta, Y. Sawa and T. Ishikawa, Wax Ester Synthase/Diacylglycerol Acyltransferase Isoenzymes Play a Pivotal Role in Wax Ester Biosynthesis in Euglena gracilis, Sci. Rep., 2017, 7, 13504 CrossRef.
- Y. Yoshida, T. Tomiyama, T. Maruta, M. Tomita, T. Ishikawa and K. Arakawa,
De novo assembly and comparative transcriptome analysis of Euglena gracilis in response to anaerobic conditions, BMC Genomics, 2016, 17, 182 CrossRef.
- T. Furuhashi, T. Ogawa, R. Nakai, M. Nakazawa, A. Okazawa, A. Padermschoke, K. Nishio, M. Hirai, M. Arita and D. Ohta, Wax ester and lipophilic compound profiling of Euglena gracilis by gas chromatography-mass spectrometry: toward understanding of wax ester fermentation under hypoxia, Metabolomics, 2014, 11, 175–183 CrossRef.
- M. Hoffmeister, A. van der Klei, C. Rotte, K. W. van Grinsven, J. J. van Hellemond, K. Henze, A. G. Tielens and W. Martin,
Euglena gracilis rhodoquinone:ubiquinone ratio and mitochondrial proteome differ under aerobic and anaerobic conditions, J. Biol. Chem., 2004, 279, 22422–22429 CrossRef CAS.
-
J. Yanowitz, M. A. Ratcliff, R. L. McCormick, J. D. Taylor and M. J. Murphy, in Tech. Rep., National Renewable Energy Laboratory, 2014, http://www.nrel.gov/docs/fy14osti/61693.pdf, (accessed July, 2020).
- S. Shibata, S. I. Arimura, T. Ishikawa and K. Awai, Alterations of membrane lipid content correlated with chloroplasts and mitochondria development in Euglena gracilis, Front. Plant Sci., 2018, 9, 370 CrossRef.
- K. D. Chapman, J. M. Dyer and R. T. Mullen, Biogenesis and functions of lipid droplets in plants: Thematic Review Series: Lipid droplet synthesis and metabolism: from Yeast to Man, J. Lipid Res., 2012, 53, 215–226 CrossRef CAS.
- A. H. M. M. Reza, J. Tavakoli, Y. Zhou, J. Qin and Y. Tang, Synthetic fluorescent probes to apprehend calcium signalling in lipid droplet accumulation in microalgae—an updated review, Sci. China: Chem., 2020, 63 Search PubMed.
- B. Fuchs, R. Süβ, K. Teubera, M. Eibisch and J. Schiller, Lipid analysis by thin-layer chromatography-a review of the current state, J. Chromatogr. A, 2011, 1218, 2754–2774 CrossRef CAS.
- P. Volin, Analysis of steroidal lipids by gas and liquid chromatography, J. Chromatogr. A, 2001, 935, 125–140 CrossRef CAS.
- H. Nygren, T. Seppänen-Laakso and H. Rischer, Liquid chromatography-mass spectrometry (LC-MS)-based analysis of molecular lipids in algae samples, Methods Mol. Biol., 2020, 1980, 215–222 CrossRef CAS.
- M. Ishihara, T. Kujiraoka, T. Iwasaki, M. Nagano, M. Takano, J. Ishii, M. Tsuji, H. Ide, I. P. Miller, N. E. Miller and H. Hattori, A sandwich enzyme-linked immunosorbent assay for human plasma apolipoprotein A-V concentration, J. Lipid Res., 2005, 46, 2015–2022 CrossRef CAS.
- J. Li, T. Vosegaard and Z. Guo, Applications of nuclear magnetic resonance in lipid analyses: An emerging powerful tool for lipidomics studies, Prog. Lipid Res., 2017, 68, 37–56 CrossRef CAS.
- L. Peng, S. Xu, X. Zheng, X. Cheng, R. Zhang, J. Liu, B. Liu and A. Tong, Rational design of a red-emissive fluorophore with AIE and ESIPT characteristics and its application in light-up sensing of esterase, Anal. Chem., 2017, 89, 3162–3168 CrossRef CAS.
- L. Li, J. Han, Z. Wang, J. Liu, J. Wei, S. Xiong and Z. Zhao, Mass spectrometry methodology in lipid analysis, Int. J. Mol. Sci., 2014, 15, 10492–10507 CrossRef.
- A. Fujita, J. Cheng and T. Fujimoto, Quantitative electron microscopy for the nanoscale analysis of membrane lipid distribution, Nat. Protoc., 2010, 5, 661–669 CrossRef CAS.
- N. Nitta, T. Iino, A. Isozaki, M. Yamagishi, Y. Kitahama, S. Sakuma, Y. Suzuki, H. Tezuka, M. Oikawa, F. Arai, T. Asai, D. Deng, H. Fukuzawa, M. Hase, T. Hasunuma, T. Hayakawa, K. Hiraki, K. Hiramatsu, Y. Hoshino, M. Inaba, Y. Inoue, T. Ito, M. Kajikawa, H. Karakawa, Y. Kasai, Y. Kato, H. Kobayashi, C. Lei, S. Matsusaka, H. Mikami, A. Nakagawa, K. Numata, T. Ota, T. Sekiya, K. Shiba, Y. Shirasaki, N. Suzuki, S. Tanaka, S. Ueno, H. Watarai, T. Yamano, M. Yazawa, Y. Yonamine, D. Di Carlo, Y. Hosokawa, S. Uemura, T. Sugimura, Y. Ozeki and K. Goda, Raman image-activated cell sorting, Nat. Commun., 2020, 11, 3452 CrossRef CAS.
- Y. Wakisaka, Y. Suzuki, O. Iwata, A. Nakashima, T. Ito, M. Hirose, R. Domon, M. Sugawara, N. Tsumura, H. Watarai, T. Shimobaba, K. Suzuki, K. Goda and Y. Ozeki, Probing the metabolic heterogeneity of live Euglena gracilis with stimulated Raman scattering microscopy, Nat. Microbiol., 2016, 1, 16124 CrossRef CAS.
- D. Jaeger, C. Pilger, H. Hachmeister, E. Oberländer, R. Wördenweber, J. Wichmann, J. H. Mussgnug, T. Huser and O. Kruse, Label-free in vivo analysis of intracellular lipid droplets in the oleaginous microalga Monoraphidium neglectum by coherent Raman scattering microscopy, Sci. Rep., 2016, 6, 35340 CrossRef CAS.
- P. J. Horn, N. R. Ledbetter, C. N. James, W. D. Hoffman, C. R. Case, G. F. Verbeck and K. D. Chapman, Visualization of lipid droplet composition by direct organelle mass spectrometry,
J. Biol. Chem., 2011, 286, 3298–3306 CrossRef CAS.
- A. Patel, I. Antonopoulou, J. Enman, U. Rova, P. Christakopoulos and L. Matsakas, Lipids detection and quantification in oleaginous microorganisms: an overview of the current state of the art, BMC Chem. Eng., 2019, 1, 13 CrossRef.
- H. Mikami, M. Kawaguchi, C. J. Huang, H. Matsumura, T. Sugimura, K. Huang, C. Lei, S. Ueno, T. Miura, T. Ito, K. Nagasawa, T. Maeno, H. Watarai, M. Yamagishi, S. Uemura, S. Ohnuki, Y. Ohya, H. Kurokawa, S. Matsusaka, C. W. Sun, Y. Ozeki and K. Goda, Virtual-freezing fluorescence imaging flow cytometry, Nat. Commun., 2020, 11, 1162 CrossRef CAS.
- Y. Suzuki, K. Kobayashi, Y. Wakisaka, D. Deng, S. Tanaka, C. Huang, C. Lei, C. Sun, H. Liu, Y. Fujiwaki, S. Lee, A. Isozaki, Y. Kasai, T. Hayakawa, S. Sakuma, F. Arai, K. Koizumi, H. Tezuka, M. Inaba, K. Hiraki, T. Ito, M. Hase, S. Matsusaka, K. Shiba, K. Suga, M. Nishikawa, M. Jona, Y. Yatomi, Y. Yalikun, Y. Tanaka, T. Sugimura, N. Nitta, K. Goda and Y. Ozeki, Label-free chemical imaging flow cytometry by high-speed multicolor stimulated Raman scattering, Proc. Natl. Acad. Sci. U. S. A., 2019, 116, 15842–15848 CrossRef CAS.
- J. Rumin, H. Bonnefond, B. Saint-Jean, C. Rouxel, A. Sciandra, O. Bernard, J. P. Cadoret and G. Bougaran, The use of fluorescent Nile red and BODIPY for lipid measurement in microalgae, Biotechnol. Biofuels, 2015, 8, 42 CrossRef.
- S. J. Yu, M. W. Kang, H. C. Chang, K. M. Chen and Y. C. Yu, Bright fluorescent nanodiamonds:
No photobleaching and low cytotoxicity, J. Am. Chem. Soc., 2005, 127, 17604–17605 CrossRef CAS.
- M. Collot, T. K. Fam, P. Ashokkumar, O. Faklaris, T. Galli, L. Danglot and A. S. Klymchenko, Ultrabright and fluorogenic probes for multicolor imaging and tracking of lipid droplets in cells and tissues, J. Am. Chem. Soc., 2018, 140, 5401–5411 CrossRef CAS.
- P. Greenspan, E. P. Mayer and S. D. Fowler, Nile red: a selective fluorescent stain for intracellular lipid droplets, J. Cell Biol., 1985, 100, 965–973 CrossRef CAS.
- Thermo Fisher Scientific BODIPY 493/503, https://www.thermofisher.com/order/catalog/product/D3922, (accessed July 2019).
- Thermo Fisher Scientific BODIPY 505/515, https://www.thermofisher.com/order/catalog/product/D3921, (accessed July 2019).
- D. Elsey, D. Jameson, B. Raleigh and M. J. Cooney, Fluorescent measurement of microalgal neutral lipids, J. Microbiol. Methods, 2007, 68, 639–642 CrossRef CAS.
- T. Mutanda, D. Ramesh, S. Karthikeyan, S. Kumari, A. Anandraj and F. Bux, Bioprospecting for hyper-lipid producing microalgal strains for sustainable biofuel production, Bioresour. Technol., 2011, 102, 57–70 CrossRef CAS.
- M. B. Brown, J. N. Miller and N. J. Seare, An investigation of the use of Nile red as a long-wavelength fluorescent probe for the study of alpha 1-acid glycoprotein-drug interactions, J. Pharm. Biomed. Anal., 1995, 13, 1011–1017 CrossRef CAS.
- W. J. Brown, T. R. Sullivan and P. Greenspan, Nile red staining of lysosomal phospholipid inclusions, Histochemistry, 1992, 97, 349 CAS.
- J. Rumin, H. Bonnefond, B. Saint-Jean, C. Rouxel, A. Sciandra, O. Bernard, J. P. Cadoret and G. Bougaran, The use of fluorescent Nile red and BODIPY for lipid measurement in microalgae, Biotechnol. Biofuels, 2015, 8, 42 CrossRef.
- J. Koreivienė, Microalgae lipid staining with fluorescent BODIPY dye, Methods Mol. Biol., 2020, 1980, 47–53 CrossRef.
- H. De la Hoz Sieglera, W. Ayidzoea, A. Ben-Zvia, R. E. Burrellab and W. C. McCaffrey, Improving the reliability of fluorescence-based neutral lipid content measurements in microalgal cultures, Algal Res., 2012, 1, 176–184 CrossRef.
- S. Daemen, M. A. M. J. van Zandvoort, S. H. Parekh and M. K. C. Hesselink, Microscopy tools for the investigation of intracellular lipid storage and dynamics, Mol. Metab., 2015, 5, 153–163 CrossRef.
- B. Liu, A. Pucci and T. Baumgartner, Aggregation induced emission: a land of opportunities, Mater. Chem. Front., 2017, 1, 1689–1690 RSC.
- X. Zhang, B. Yao, Q. Hu, Y. Hong, A. Wallace, K. Reynolds, C. Ramsey, A. Maeder, R. Reeda and Y. Tang, Detection of biomarkers in body fluids using bioprobes based on aggregation-induced emission fluorogens, Mater. Chem. Front., 2020, 4, 2548–2570 RSC.
- K. C. Chong, F. Hu and B. Liu, AIEgen bioconjugates for specific detection of disease-related protein biomarkers, Mater. Chem. Front., 2019, 3, 12–24 RSC.
- Y. J. Li, H. T. Zhang, X. Y. Chen, P. F. Gao and C. H. Hu, A multifunctional AIEgen with high cell-penetrating ability for intracellular fluorescence assays, imaging and drug delivery, Mater. Chem. Front., 2019, 3, 1151–1158 RSC.
- Z. Wang, C. Gui, E. Zhao, J. Wang, X. Li, A. Qin, Z. Zhao, Z. Yu and B. Z. Tang, Specific fluorescence probes for lipid droplets based on simple AIEgens, ACS Appl. Mater. Interfaces, 2016, 8, 10193–10200 CrossRef CAS.
- E. C. O'Neill, S. Kuhaudomlarp, M. Rejzek, J. U. Fangel, K. Alagesan, D. Kolarich, W. G. T. Willats and R. A. Field, Exploring the glycans of Euglena gracilis, Biology, 2017, 6, E45 CrossRef.
- J. Wang, J. Sun, Y. Song, Y. Xu, X. Pan, Y. Sun and D. Li, A label-free microfluidic biosensor for activity detection of single microalgae cells based on chlorophyll fluorescence, Sensors, 2013, 13, 16075–16089 CrossRef CAS.
- E. G. Bligh and W. J. Dyer, A rapid method of total lipid extraction and purification, Can. J. Biochem. Physiol., 1959, 37, 911–917 CrossRef CAS.
- W. W. Christie, Preparation of ester derivatives of fatty acids for chromatographic analysis, Adv. Lipid Methodol., 1993, 2, e111 Search PubMed.
- M. S. Cooper, W. R. Hardin, T. W. Petersen and R. A. Cattolico, Visualizing “green oil” in live algal cells, J. Biosci. Bioeng., 2010, 109, 198–201 CrossRef CAS.
- C. A. Schneider, W. S. Rasband and K. W. Eliceiri, NIH Image to ImageJ: 25 years of image analysis, Nat. Methods, 2012, 9, 671–675 CrossRef CAS.
- M. Tossavainen, U. Ilyass, V. Ollilainen, K. Valkonen, A. Ojala and M. Romantschuk, Influence of long term nitrogen limitation on lipid, protein and pigment production of Euglena gracilis in photoheterotrophic cultures, PeerJ, 2019, 7, e6624 CrossRef.
- F. Ren, P. Liu, Y. Gao, J. Shi, B. Tong, Z. Cai and Y. Dong, Real time bioimaging for mitochondria by taking the aggregation process of aggregation-induced emission near-infrared dyes with wash-free staining, Mater. Chem. Front., 2019, 3, 57–63 RSC.
- H. Alishah Aratboni, N. Rafiei, R. Garcia-Granados, A. Alemzadeh and J. R. Morones-Ramírez, Biomass and lipid induction strategies in microalgae for biofuel production and other applications, Microb. Cell Fact., 2019, 18, 178 CrossRef.
- S. Wu, B. Zhang, A. Huang, L. Huan, L. He, A. Lin, J. Niu and G. Wang, Detection of intracellular neutral lipid content in the marine microalgae Prorocentrum micans and Phaeodactylum tricornutum using Nile red and BODIPY 505/515, J. Appl. Psychol., 2014, 26, 1659–1668 CAS.
- U. Goodenough, I. Blaby, D. Casero, S. D. Gallaher, C. Goodson, S. Johnson, J. H. Lee, S. S. Merchant, M. Pellegrini, R. Roth, J. Rusch, M. Singh, J. G. Umen, T. L. Weiss and T. Wulan, The path to triacylglyceride obesity in the sta6 strain of Chlamydomonas reinhardtii, Eukaryotic Cell, 2014, 13, 591–613 CrossRef.
- E. C. Goncalves, A. C. Wilkie, M. Kirst and B. Rathinasabapathi, Metabolic regulation of triacylglycerol accumulation in the green algae: identification of potential targets for engineering to improve oil yield, Plant Biotechnol. J., 2016, 14, 1649–1660 CrossRef CAS.
- L. D. Zhu, Z. H. Li and E. Hiltunen, Strategies for lipid production improvement in microalgae as a biodiesel feedstock, BioMed Res. Int., 2016, 8792548 CAS.
- H. Wang, Y. Zhang, W. Zhou, L. Noppol and T. Liu, Mechanism and enhancement of lipid accumulation in filamentous oleaginous microalgae Tribonema minus under heterotrophic condition, Biotechnol. Biofuels, 2018, 11, 328 CrossRef CAS.
- R. Praveenkumar, K. Shameera, G. Mahalakshmi, M. A. Akbarsha and N. Thajuddin, Influence of nutrient deprivations on lipid accumulation in a dominant indigenous microalga Chlorella sp., BUM11008: evaluation for biodiesel production, Biomass Bioenergy, 2012, 37, 60–66 CrossRef CAS.
- R. Miller, G. Wu, R. R. Deshpande, A. Vieler, K. Gärtner, X. Li, E. R. Moellering, S. Zäuner, A. J. Cornish, B. Liu, B. Bullard, B. B. Sears, M. H. Kuo, E. L. Hegg, Y. Shachar-Hill, S. H. Shiu and C. Benning, Changes in transcript abundance in Chlamydomonas reinhardtii following nitrogen deprivation predict diversion of metabolism, Plant Physiol., 2010, 154, 1737–1752 CrossRef CAS.
- J. Fan, C. Yan, C. Andre, J. Shanklin, J. Schwender and C. Xu, Oil accumulation is controlled by carbon precursor supply for fatty acid synthesis in Chlamydomonas reinhardtii, Plant Cell Physiol., 2012, 53, 1380–1390 CrossRef CAS.
- J. Fan, C. Yan and C. Xu, Phospholipid:diacylglycerol acyltransferase-mediated triacylglycerol biosynthesis is crucial for protection against fatty acid-induced cell death in growing tissues of Arabidopsis, Plant J., 2013, 76, 930–942 CrossRef CAS.
- X. Deng, J. Cai and X. Fei, Effect of the expression and knockdown of citrate synthase gene on carbon flux during triacylglycerol biosynthesis by green algae Chlamydomonas reinhardtii, BMC Biochem., 2013, 14, 38 CrossRef.
- V. H. Work, R. Radakovits, R. E. Jinkerson, J. E. Meuser, L. G. Elliott, D. J. Vinyard, L. M. Laurens, G. C. Dismukes and M. C. Posewitz, Increased lipid accumulation in the Chlamydomonas reinhardtii sta7-10 starchless isoamylase mutant and increased carbohydrate synthesis in complemented strains, Eukaryotic Cell, 2010, 9, 1251–1261 CrossRef CAS.
- Y. Li, D. Han, G. Hu, D. Dauvillee, M. Sommerfeld, S. Ball and Q. Hu,
Chlamydomonas starchless mutant defective in ADP-glucose pyrophosphorylase hyper-accumulates triacylglycerol, Metab. Eng., 2010, 12, 387–391 CrossRef CAS.
- S. Sello, R. Moscatiello, N. Mehlmer, M. Leonardelli, L. Carraretto, E. Cortese, F. G. Zanella, B. Baldan, I. Szabò, U. C. Vothknecht and L. Navazio, Chloroplast Ca2+ fluxes into and across thylakoids revealed by thylakoid-targeted aequorin probes, Plant Physiol., 2018, 177, 38–51 CrossRef CAS.
- H. Takahashi, S. Clowez, F. A. Wollman, O. Vallon and F. Rappaport, Cyclic electron flow is redox-controlled but independent of state transition, Nat. Commun., 2013, 4, 1954 CrossRef.
- J. Alric, J. Lavergne and F. Rappaport, Redox and ATP control of photosynthetic cyclic electron flow in Chlamydomonas reinhardtii (I) aerobic conditions, Biochim. Biophys. Acta, 2010, 1797, 44–51 CrossRef CAS.
- Y. M. Zhang, H. Chen, C. L. He and Q. Wang, Nitrogen Starvation Induced Oxidative Stress in an Oil-Producing Green Alga Chlorella sorokiniana C3, PLoS One, 2013, 8, e69225 CrossRef CAS.
- T. Shikanai, Cyclic electron transport around photosystem I: genetic approaches, Annu. Rev. Plant Biol., 2007, 58, 199–217 CrossRef CAS.
- S. Schmollinger, T. Mühlhaus, N. R. Boyle, I. K. Blaby, D. Casero, T. Mettler, J. L. Moseley, J. Kropat, F. Sommer, D. Strenkert, D. Hemme, M. Pellegrini, A. R. Grossman, M. Stitt, M. Schroda and S. S. Merchant, Nitrogen-sparing mechanisms in Chlamydomonas affect the transcriptome, the proteome, and photosynthetic metabolism, Plant Cell, 2014, 26, 1410–1435 CrossRef CAS.
- M. Terashima, D. Petroutsos, M. Hüdig, I. Tolstygina, K. Trompelt, P. Gäbelein, C. Fufezan, J. Kudla, S. Weinl, G. Finazzi and M. Hippler, Calcium-dependent regulation of cyclic photosynthetic electron transfer by a CAS, ANR1, and PGRL1 complex, Proc. Natl. Acad. Sci. U. S. A., 2012, 109, 17717–17722 CrossRef CAS.
- H. Chen, J. Hu, Y. Qiao, W. Chen, J. Rong, Y. Zhang, C. He and Q. Wang, Ca2+-regulated cyclic electron flow supplies ATP for nitrogen starvation-induced lipid biosynthesis in green alga, Sci. Rep., 2015, 5, 15117 CrossRef CAS.
- Z. Chen, M. K. Yang, C. Y. Li, Y. Wang, J. Zhang, D. B. Wang, X. E. Zhang and F. Ge, Phosphoproteomic analysis provides novel insights into stress responses in Phaeodactylum tricornutum, a model diatom, J. Proteome Res., 2014, 13, 2511–2523 CrossRef CAS.
- S. Stael, B. Wurzinger, A. Mair, N. Mehlmer, U. C. Vothknecht and M. Teige, Plant organellar calcium signalling: an emerging field, J. Exp. Bot., 2012, 63, 1525–1542 CrossRef CAS.
- R. Fristedt, P. Granath and A. V. Vener, A protein phosphorylation threshold for functional stacking of plant photosynthetic membranes, PLoS One, 2010, 5, e10963 CrossRef.
- R. Fristedt, A. Willig, P. Granath, M. Crèvecoeur, J. D. Rochaix and A. V. Vener, Phosphorylation of photosystem II controls functional macroscopic folding of photosynthetic membranes in arabidopsis, Plant Cell, 2009, 21, 3950–3964 CrossRef CAS.
- M. Siaut, S. Cuiné, C. Cagnon, B. Fessler, M. Nguyen, P. Carrier, A. Beyly, F. Beisson, C. Triantaphylidès, Y. Li-Beisson and G. Peltier, Oil accumulation in the model green alga Chlamydomonas reinhardtii: characterization, variability between common laboratory strains and relationship with starch reserves, BMC Biotechnol., 2011, 11, 7 CrossRef CAS.
- I. K. Blaby, A. G. Glaesener, T. Mettler, S. T. Fitz-Gibbon, S. D. Gallaher, B. Liu, N. R. Boyle, J. Kropat, M. Stitt, S. Johnson, C. Benning, M. Pellegrini, D. Casero and S. S. Merchant, Systems-level analysis of nitrogen starvation-induced modifications of carbon metabolism in a Chlamydomonas reinhardtii starchless mutant, Plant Cell, 2013, 25, 4305–4323 CrossRef CAS.
- Y. Hong, J. W. Lam and B. Z. Tang, Aggregation-induced emission: phenomenon, mechanism and applications, Chem. Commun., 2009, 4332–4353 RSC.
- T. Govender, L. Ramanna, I. Rawat and F. Bux, BODIPY staining, an alternative to the Nile Red fluorescence method for the evaluation of intracellular lipids in microalgae, Bioresour. Technol., 2012, 114, 507–511 CrossRef CAS.
- H. D. Siegler, W. Ayidzoe, A. Ben-Zvi, R. E. Burrell and W. C. McCaffrey, Improving the reliability of fluorescence-based neutral lipid content measurements in microalgal cultures, Algal Res., 2012, 1, 176–184 CrossRef.
- E. Wang, E. Zhao, Y. Hong, J. Lam and B. Z. Tang, A highly selective AIE fluorogen for lipid droplet imaging in live cells and green algae, J. Mater. Chem. B, 2014, 2, 2013–2019 RSC.
- H. Peng, L. He and V. S. Haritos, Flow-cytometry-based physiological characterisation and transcriptome analyses reveal a mechanism for reduced cell viability in yeast engineered for increased lipid content, Biotechnol. Biofuels, 2019, 12, 98 CrossRef.
- K. Iwasaki, A. Kaneko, Y. Tanaka, T. Ishikawa, H. Noothalapati and T. Yamamoto, Visualizing wax ester fermentation in single Euglena gracilis cells by Raman microspectroscopy and multivariate curve resolution analysis, Biotechnol. Biofuels, 2019, 12, 128 CrossRef.
- S. Takaichi, Carotenoids in algae: distributions, biosyntheses and functions, Mar. Drugs, 2011, 9, 1101–1118 CrossRef CAS.
- J. P. Schwarzhans, D. Cholewa, P. Grimm, U. Beshay, J. M. Risse, K. Friehs and E. Flaschel, Dependency of the fatty acid composition of Euglena gracilis on growth phase and culture conditions, J. Appl. Phycol., 2015, 27, 1389–1399 CrossRef CAS.
- B. Santek, M. Felski, K. Friehs, M. Lotz and E. Flaschel, Production of paramylon, a β-1,3-glucan, by heterotrophic cultivation of Euglena gracilis on a synthetic medium, Eng. Life Sci., 2009, 9, 23–28 CrossRef CAS.
- E. W. Becker, Micro-algae as a source of protein, Biotechnol. Adv., 2007, 25, 207–210 CrossRef CAS.
- Y. Liang, N. Sarkany and Y. Cui, Biomass and lipid productivities of Chlorella vulgaris under autotrophic, heterotrophic and mixotrophic growth conditions, Biotechnol. Lett., 2009, 31, 1043–1049 CrossRef CAS.
- T. Heredia-Arroyo, W. Wei and B. Hu, Oil accumulation via heterotrophic/mixotrophic Chlorella protothecoides, Appl. Biochem. Biotechnol., 2010, 162, 1978–1995 CrossRef CAS.
- E. Sokoła-Wysoczańska, T. Wysoczański, J. Wagner, K. Czyż, R. Bodkowski, S. Lochyński and B. Patkowska-Sokoła, Polyunsaturated fatty acids and their potential therapeutic role in cardiovascular system disorders-a review, Nutrients, 2018, 10, 1561 CrossRef.
- A. Molfino, G. Gioia, F. Rossi Fanelli and M. Muscaritoli, The role for dietary omega-3 fatty acids supplementation in older adults, Nutrients, 2014, 6, 4058–4073 CrossRef CAS.
- W. J. Lukiw and N. G. Bazan, Docosahexaenoic acid and the aging brain, J. Nutr., 2008, 138, 2510–2514 CrossRef CAS.
- S. C. Dyall, Long-chain omega-3 fatty acids and the brain: a review of the independent and shared effects of EPA, DPA and DHA, Front. Aging Neurosci., 2015, 7, 52 Search PubMed.
- I. B. Asztalos, J. A. Gleason, S. Sever, R. Gedik, B. F. Asztalos, K. V. Horvath, M. L. Dansinger, S. Lamon-Fava and E. J. Schaefer, Effects of eicosapentaenoic acid and docosahexaenoic acid on cardiovascular disease risk factors: a randomized clinical trial, Metab., Clin. Exp., 2016, 65, 1636–1645 CrossRef CAS.
- R. D. S. Freitas and M. M. Campos, Protective effects of omega-3 fatty acids in cancer-related complications, Nutrients, 2019, 11, 945 CrossRef CAS.
- R. Avallone, G. Vitale and M. Bertolotti, Omega-3 fatty acids and neurodegenerative diseases: new evidence in clinical trials, Int. J. Mol. Sci., 2019, 20, 4256 CrossRef CAS.
- T. Koto, N. Nagai, H. Mochimaru, T. Kurihara, K. Izumi-Nagai, S. Satofuka, H. Shinoda, K. Noda, Y. Ozawa, M. Inoue, K. Tsubota, Y. Oike and S. Ishida, Eicosapentaenoic acid is anti-inflammatory in preventing choroidal neovascularization in mice, Invest. Ophthalmol. Visual Sci., 2007, 48, 4328–4334 CrossRef.
- A. Balić, D. Vlašić, K. Žužul, B. Marinović and Z. Bukvić Mokos, Omega-3 versus omega-6 polyunsaturated fatty acids in the prevention and treatment of inflammatory skin diseases, Int. J. Mol. Sci., 2020, 21, 741 CrossRef.
- D. Fussbroich, R. A. Colas, O. Eickmeier, J. Trischler, S. P. Jerkic, K. Zimmermann, A. Göpel, T. Schwenger, A. Schaible, D. Henrich, P. Baer, S. Zielen, J. Dalli, C. Beermann and R. Schubert, A combination of LCPUFA ameliorates airway inflammation in asthmatic mice by promoting pro-resolving effects and reducing adverse effects of EPA, Mucosal Immunol., 2020, 13, 481–492 CrossRef CAS.
- D. Tricò, A. Mengozzi, L. Nesti, M. Hatunic, R. Gabriel Sanchez, T. Konrad, K. Lalić, N. M. Lalić, A. Mari, A. Natali and EGIR-RISC Study Group, Circulating palmitoleic acid is an independent determinant of insulin sensitivity, beta cell function and glucose tolerance in non-diabetic individuals: a longitudinal analysis, Diabetologia, 2020, 63, 206–218 CrossRef.
- M. E. Frigolet and R. Gutiérrez-Aguilar, The role of the novel lipokine palmitoleic acid in health and disease, Adv. Nutr., 2017, 8, 173S–181S CrossRef CAS.
- J. R. Silva, B. Burger, C. Kühl, T. Candreva, M. Dos Anjos and H. G. Rodrigues, Wound healing and omega-6 fatty acids: from inflammation to repair, Mediators Inflammation, 2018, 2018, 2503950 Search PubMed.
- E. L. Guo and R. Katta, Diet and hair loss: effects of nutrient deficiency and supplement use, Dermatol. Pract. Concept., 2017, 7, 1–10 CAS.
- G. C. Burdge and P. C. Calder, Conversion of alpha-linolenic acid to longer-chain polyunsaturated fatty acids in human adults, Reprod., Nutr., Dev., 2005, 45, 581–597 CrossRef CAS.
- B. M. Anderson and D. W. Ma, Are all n-3 polyunsaturated fatty acids created equal?, Lipids Health Dis., 2009, 8, 33 CrossRef.
- G. Y. Sun, A. Simonyi, K. L. Fritsche, D. Y. Chuang, M. Hannink, Z. Gu, C. M. Greenlief, J. K. Yao, J. C. Lee and D. Q. Beversdorf, Docosahexaenoic acid (DHA): An essential nutrient and a nutraceutical for brain health and diseases, Prostaglandins, Leukotrienes Essent. Fatty Acids, 2018, 136, 3–13 CrossRef CAS.
- P. Guesnet and J. M. Alessandri, Docosahexaenoic acid (DHA) and the developing central nervous system (CNS) - Implications for dietary recommendations, Biochimie, 2011, 93, 7–12 CrossRef CAS.
- S. Gutiérrez, S. L. Svahn and M. E. Johansson, Effects of omega-3 fatty acids on immune cells, Int. J. Mol. Sci., 2019, 20, 5028 CrossRef.
- M. J. Weiser, C. M. Butt and M. H. Mohajeri, Docosahexaenoic acid and cognition throughout the lifespan, Nutrients, 2016, 8, 99 CrossRef.
Footnote |
† Electronic supplementary information (ESI) available. See DOI: 10.1039/d0qm00621a |
|
This journal is © the Partner Organisations 2021 |