DOI:
10.1039/D0QI01037E
(Research Article)
Inorg. Chem. Front., 2021,
8, 109-121
FeCo nanoalloys embedded in nitrogen-doped carbon nanosheets/bamboo-like carbon nanotubes for the oxygen reduction reaction†
Received
26th August 2020
, Accepted 21st October 2020
First published on 22nd October 2020
Abstract
Herein, FeCo bimetallic organic frameworks (MOFs) with different compositions were fabricated by controlling the initial molar ratio of Fe3+/Co2+ ions. Through a one-step carbonization treatment of FeCo MOFs and the melamine mixture, a series of FeCo nanoalloys encapsulated in bamboo-like N-doped carbon nanotubes (CNTs), entangled with N-doped carbon nanosheet nanocomposites (FexCoy–N–C), were prepared. The FexCoy–N–C materials presented an excellent ORR performance in alkaline medium. X-ray photoelectron spectroscopy (XPS) studies revealed the existence of ORR-active centers, such as Co–Nx, pyridinic-N and graphitic-N, which were responsible for the enhanced ORR activity. Besides, the synergistic coupling between FeCo alloy and N-doped carbon nanotubes favors the enhancement of the electrocatalytic performance. Among FexCoy–N–C electrocatalysts, the optimized Fe1Co3–N–C and Fe3Co1–N–C catalysts showed higher ORR performance. In addition, both Fe1Co3–N–C and Fe3Co1–N–C showed superior stability compared to the benchmark commercial Pt/C catalyst.
1. Introduction
With population growth and rapid economic development, more and more fossil fuels are being used, leading to increased global warming and environmental pollution. Fuel cells (FCs) have been considered as an alternative to achieve the utilization of clean energy due to their high energy conversion efficiency and “zero” pollutant emission.1–4 One technical limitation requiring efficient electrocatalysts is the sluggish kinetically controlled ORR at the cathode.5–7 To date, Pt-based materials have been employed as commercial catalysts for the ORR.8,9 However, noble metal-based catalysts suffer from high cost, inferior stability, and intolerance to the fuel crossover phenomenon, which hinder the large-scale commercialization of fuel cells.10,11 Therefore, intensive efforts have been devoted to exploring highly efficient and stable ORR electrocatalysts based on non-precious metal centers.12–14 In particular, metal/N-doped carbon materials, e.g., M–N–C, M = Fe, Co, are recognized as one of the most promising non-precious catalysts owing to their low cost and desirable catalytic activity.15,16 It was reported that Fe–N–C materials possess superior ORR activity compared to rather stable Co–N–C catalysts.17,18 With respect to activity and stability, the synergistic effect between the Fe and Co centers favors better ORR performance than their monometallic counterparts.18–27 The enhancement of the electrocatalytic ORR performance is induced by various types of active sites present in the metallic Fe–Co–N–C catalysts. These active sites are metal–nitrogen moieties embedded in carbon substrates (labeled as Fe–Nx and Co–Nx),17,28–33 bimetallic alloy species encapsulated in a nitrogen doped carbon matrix (labeled as FeCo@C),6,34–46 and nitrogen–carbon moieties (labeled as NxCy).47 With regard to the interfacial electrocatalytic reactions, an efficient transport of reactants and electrons to the active sites is another crucial factor for fabricating high-performing electrocatalysts. For example, various 3D-structural electrocatalysts consisting of 0D nanoalloys, 1D nanotubes and 2D nanosheets, such as Fe/Fe3C@NGL–NCNTs,48 Fe@C–NG/NCNTs,49 CoFe/N–GCT,41 FeNi@N–CNT/NCSs,50 and CoFe/Co@NCNT/NG,26 have been prepared with an increased number of active sites and large surface areas to improve the ORR electrocatalytic performance.
Metal–organic frameworks (MOFs), with well-tunable physico-chemical properties, such as a large surface area, functional modification, and adjustable porosity, have served as self-sacrificing templates to prepare transition metal nanoparticles/carbon composites, especially Fe(Co)–N/C materials.3 Those from the carbonization of MOFs possibly display the following qualities: (i) high distribution of M–N active moieties; (ii) various types of nitrogen species; (iii) large surface area and improved accessibility of active sites; (iv) porous structure; and (v) enhanced electrical conductivity as a result of graphitized carbon. Recently, Chen et al.51 reported a host–guest strategy using FemIm nanocluster (NC) in a zeolite imidazole framework-8 (ZIF-8) to obtain well-configured Fe–N/C electrocatalysts. Due to the host–guest effect, the Fe–N/C catalysts produced various types of Fe–Nx models, including N–Fe–N4, Fe–N4, and Fe–N2, as a result of which 5% Fe–N/C catalyst with abundant N–Fe–N4 sites showed an outstanding ORR activity in acidic media. Zhong et al.52 reported cobalt nanoparticles interacting with N-doped carbon nanotubes (Co/CoNx/N–CNT/C) produced by in situ pyrolysis of Co-mela-BDC (Co MOF, composed of Co ions, 1,4-dicarboxybenzene ligands (BDC) and melamine) at 800 °C under a N2 atmosphere. The Co/CoNx/N–CNT/C showed high density of NCNTs and N content resulting in excellent ORR activity (Eonset = 0.90 V, E1/2 = 0.80 V vs. RHE), close to Pt/C values (Eonset = 0.93 V, E1/2 = 0.80 V) in alkaline media.
Inspired by these facts, a series of FeCo nanoalloys embedded in bamboo-like N-doped carbon nanotubes (CNTs), entangled with N-doped carbon nanosheet (FexCoy–N–C) nanocomposites with varied ratios of Fe/Co, were successfully prepared by pyrolysis of FeCo bimetallic MOFs and melamine precursors (x and y refer to the feeding molar ratio of Fe3+/Co2+). Interestingly, the initial molar ratio of Fe3+/Co2+ for the FexCoy–N–C nanocomposite plays a key role in the ORR electrochemical performance. In particular, the Fe1Co3–N–C and Fe3Co1–N–C catalysts showed higher ORR activity and stability than Fe1Co2–N–C, Fe1Co1–N–C and Fe2Co1–N–C.
2. Experimental section
2.1 Synthesis of the bimetallic FexCoy MOFs
Typically, 3 mmol FeCl3·6H2O, 1 mmol Co(OOCCH3)2·4H2O and 6.3 mmol 1,4-dicarboxybenzene (BDC) were dissolved in mixed solvents of N,N-dimethylformamide (DMF, 40 mL) and absolute ethanol (20 mL). The resulting mixture was treated by ultrasound for 30 min to obtain a homogeneous solution and then sealed in a Teflon-lined stainless steel autoclave at 150 °C for 12 h. After cooling down, the precipitate was collected by centrifugation and washed thoroughly with DMF, and dried at 60 °C overnight. The product was denoted as the Fe3Co1 MOF with a Fe3+/Co2+ molar ratio of 3
:
1.
2.2 Synthesis of FexCoy–N–C catalysts
A mixture with a ratio of FexCoy MOFs to melamine powder of 4/3 was mechanically mixed by grinding. The mixture was subjected to heat treatment under a N2 atmosphere at 250 °C with a ramp rate of 5 °C min−1 for 2 h, and then the temperature was increased up to 800 °C with the same heating rate for another 4 h. After calcination, the black powder was immersed in 10 wt% HNO3 for 3 h, washed with ultra-pure water until the pH value ≈7 was attained, and then dried at 60 °C under air. The pyrolyzed products were labeled as FexCoy–N–C (i/y = 1
:
3, 1
:
2, 1
:
1, 2
:
1, and 3
:
1), respectively.
2.3 Physicochemical characterization
The crystalline phases of products were characterized by X-ray diffraction (XRD) analysis using a Rigaku UItima III diffractometer with a scanning rate of 10° (2θ) min−1 using Cu-Kα (λ = 0.15406 nm) radiation at 40 kV and 40 mA. The SEM and HRTEM images were individually examined using a ZEISS Supra 55 scanning electron microscope (SEM) and high-resolution transmission electron microscope (HRTEM) on a Tecnai G2 F20 field emission transmission electron microscope (FEI) operated at 200 kV. X-ray photoelectron spectroscopy (XPS) was performed using an Axis Supra X-ray photoelectron spectrometer equipped with an Al/Mg double anode. Low-temperature nitrogen adsorption–desorption experiments were performed using a Gemini VII 2390 series surface area analyzer. The specific surface area was calculated according to the Brunauer–Emmett–Teller (BET) method based on the adsorption isotherm. The pore volume and pore size distribution were calculated using the Barrett–Joyner–Halenda (BJH) method. Raman spectra were recorded using a LabRAM Aramis Raman spectrometer with a visible laser beam of 532 nm.
2.4 Electrochemical measurements
The electrochemical measurements were conducted in a standard three-electrode glass cell with 0.1 M KOH as the electrolyte at 25 °C. A carbon plate and a saturated calomel electrode (SCE) were used as the counter and reference electrodes, respectively. All measured potentials vs. SCE were converted with respect to the RHE based on the formula: E(RHE) = E(SCE) + 1.009 V. A glassy carbon disk electrode with a geometric area of 0.07 cm2 was used as the working electrode, which was polished with γ-alumina powder and successively ultrasonicated in water and ethanol for 1 min prior to any measurement. The catalyst ink was prepared by dispersing 4.0 mg of catalyst in 700 μL deionized water, 250 μL isopropanol and 50 μL Nafion® mixed by ultrasound for 2 h. 4.0 μL of the ink was dropped on the working electrode surface, and dried in air at 300 rpm. The mass loading was 0.23 mg cm−2 for the FexCoy–N–C catalyst. The cyclic voltammograms (CVs) were recorded at a scan rate of 50 mV s−1 in an Ar- or O2-saturated electrolyte. In an O2-saturated electrolyte, linear sweep voltammetry (LSV) curves were recorded at a scan rate of 5 mV s−1 with different rotating speeds from 625 to 2500 rpm. The ORR kinetics was analyzed using the Koutecky–Levich (K–L) equation. | 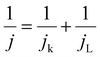 | (1) |
| 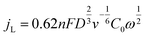 | (2) |
where j is the current density (mA cm−2); jk is the kinetic current density (mA cm−2); jL is the limiting current density (mA cm−2); n is the number of electrons transferred per oxygen molecule; F (96
485 C mol−1) is the Faraday constant; D (1.9 × 10−5 cm s−1) is the diffusion coefficient of O2 in 0.1 M KOH; ν (0.01 cm2 s−1) is the kinetic viscosity of the solution; C0 (1.2 × 10−6 mol cm−3) is the concentration of O2 in the electrolyte; and ω is the rotating rate (rad s−1).53
The number of electrons transferred (n) and the peroxide yield (H2O2%) were obtained from the rotating ring-disk electrode (RRDE) tests by using the following equations:
| 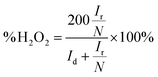 | (3) |
|  | (4) |
where
Id is the disk current (mA),
Ir is the ring current (mA), and
N, the current collection coefficient, of the Pt ring is 0.424 under our test conditions.
The electrochemically active surface area (ECSA) measurements were performed between 0.31 and 0.41 V vs. RHE at the scan rates from 20 to 120 mV s−1 in 0.1 M N2-saturated KOH. The accelerated stability test was conducted in 0.1 M O2 saturated KOH solution at a scan rate of 100 mV−1. The durability of the catalyst was tested by chronoamperometric measurement at 1600 rpm at 0.7 V in 0.1 M O2 saturated KOH solution for 9 h.
3. Results and discussion
Fig. 1a shows the X-ray diffraction (XRD) patterns of bimetallic FexCoy-MOFs with various molar ratios of Fe3+/Co2+ (x/y = 1
:
3, 1
:
2, 1
:
1, 2
:
1, 3
:
1). Their XRD patterns display several diffraction peaks centered at 9.1°, 16.7°, 18.5°, 20.6° and 25.4°/2θ, indicating a similar crystalline structure. One can observe that the diffraction peaks at around 11°/2θ are slightly shifted to a lower angle (2θ) with the molar ratio of Fe3+/Co2+, demonstrating structural differences among FexCoy-MOFs as a result of the different coordinated configurations between the Fe/Co and BDC organic ligand. The morphologies of FexCoy-MOFs were characterized using a scanning electron microscope (SEM), cf. Fig. 1b–f. The Fe1Co3, Fe1Co2 and Fe1Co1 MOFs show irregular morphological features, whereas the Fe2Co1-MOF and Fe3Co1-MOF with a higher relative amount of Fe3+ present irregular spindle-shaped nanorods. Notably, the particle size and regularity of the FexCoy-MOF are strongly associated with the concentration of Fe3+. The Fe3Co1–MOF sample displays a more uniform and well-dispersed structure with an equatorial diameter of ca. 120 nm and a length of ca. 450 nm, Fig. 1f. This phenomenon suggests that Fe3+ has an important impact on the morphology of the bimetallic FexCoy-MOF.54,55 Moreover, the morphologies of these bimetallic FexCoy-MOFs are quite different from the Fe-MOF and Co-MOF using the same BDC organic ligand as a linker,52,56 thus revealing that Fe and Co ions are involved in the formation process of bimetallic FexCoy-MOFs.
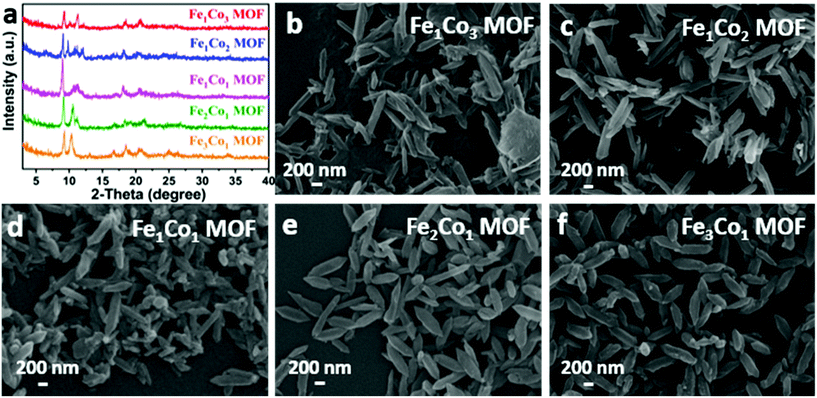 |
| Fig. 1 (a) XRD patterns of bimetallic FexCoy MOFs and SEM images of (b) Fe1Co3 MOF, (c) Fe1Co2 MOF, (d) Fe1Co1 MOF, (e) Fe2Co1 MOF and (f) Fe3Co1 MOF. | |
The bimetallic FexCoy MOFs with different compositions were further pyrolyzed at 800 °C under a N2 atmosphere for 4 h, and then subjected to acid etching to produce Fe1Co3–N–C, Fe1Co2–N–C, Fe1Co1–N–C, Fe2Co1–N–C and Fe3Co1–N–C samples. ICP-AES results clarify that the Fe content substantially increases from 1.6 to 6.1 wt% with the Fe3+/Co2+ molar ratio from 1/3 to 3/1, while Co content gradually decreases from 5.0 to 1.9 wt%, Fig. S1, ESI.†Fig. 2a depicts the XRD patterns of all FexCoy–N–C in the interval of 20–80°/2θ. The relatively strong diffraction peak at 26.3°/2θ corresponds to the (002) plane of graphitic carbon (JCPDS No. 41-1487), while the two peaks at 44.6° and 64.9°/2θ match well with the (110) and (200) planes of the FeCo alloy (JCPDS No. 48-1816), respectively. One notices that the diffraction peak position of the (110) plane gradually shifts to a lower angle with increasing Fe/Co molar ratio of the FeCo alloy, attributable to the lattice contraction in FeCo.57 In addition, along with increasing the initial Fe/Co molar ratio, the diffraction density of the FeCo alloy becomes stronger, suggesting a larger crystal size and higher crystallinity. The SEM images of FexCoy–N–C samples show the appearance of carbon nanotubes after the calcination of the FeCo MOFs and melamine mixture, suggesting that Co and Fe metals could facilitate the formation and growth of carbon nanotubes, Fig. 2b–f. Interestingly, the abundant interconnected 3D networks of NCNTs are obviously observed on the Fe1Co3–N–C and Fe3Co1–N–C samples, especially the latter, coming from the Fe3Co1 MOF precursor with a regular spindle-shape and well-dispersed structure. Here, the reaction between the FexCoy MOF template and melamine induces the following: (i) the metal nano-catalysts derived from MOFs catalyze the carbonization and formation of NCNTs; (ii) the confinement effect of the MOF matrix aids to improve the length and dispersion of NCNTs; and (iii) melamine serves as basic units of NCNTs and acts as a pore former as well as the N source.
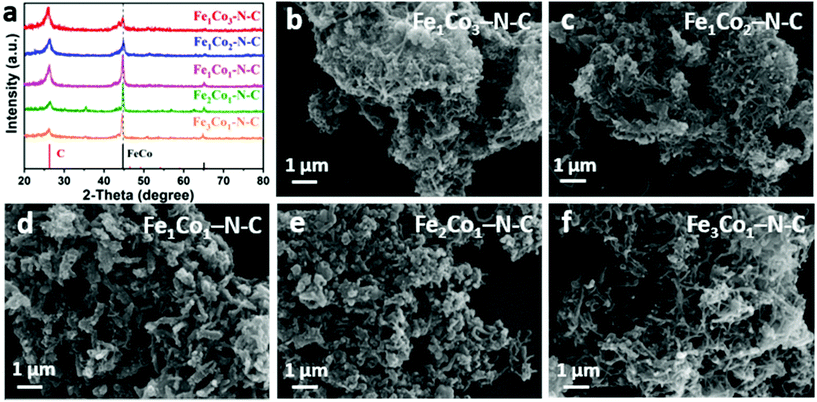 |
| Fig. 2 (a) XRD patterns of FexCoy–N–C materials and SEM images of Fe1Co3–N–C (b), Fe1Co2–N–C (c), Fe1Co1–N–C (d), Fe2Co1–N–C (e) and Fe3Co1–N–C (f). | |
In order to obtain further compositional information, Rietveld analysis (RF) was performed on all FexCoy–N–C samples, as shown in Fig. 3(a) with a blue line.33,58 One notes that the predominant phases in all samples are carbon and the FeCo alloy, whereas the presence of the Fe0.28Co0.72 phase (PDF#51-0740) is revealed in Fe1Co3–N–C (24 wt% Fe nominal). The latter was previously reported for its similarity to α-Mn,59 which agrees with the nominal mass fraction of Fe, in the Fe1Co3–N–C. On the other hand, a third phase is observed in the Fe2Co1–N–C sample, namely, CoFe2O4 (PDF#79-1744). This phenomenon is not evident when the nominal mass fraction of Fe increases from 66 wt% to 74 wt%. The structural properties of Fe–Co nanoalloys are summarized in Table 1, which shows that the crystallite size is in the range of 11 to 170.1 nm for the Fe-based samples. As discussed above, in the Fe1Co3–N–C and Fe2Co1–N–C samples, the predominant phases are Fe0.28Co0.72 and CoFe2O4, respectively. Fig. 3(b) presents the relationship of the lattice parameter (a) as a function of the nominal mass fraction of Fe present in the samples. It clearly shows that Vegard's law could be described with a mass fraction less than 20 wt% Fe, where the stable phase at 100 wt% of Co is the Co (Fm
m) phase based on the phase Fe–Co diagram.60 Sánchez-De Jesús et al.61 found that at a concentration of 10 wt% Fe, the reticular parameter is 2.897 Å. This value is very close to the calculated value of the solid solution of FeCo, cf. Table 1. In particular, at 20 wt% of Fe, the predominant phase is Fe0.28Co0.72 (I
3m) with a certain amount of FeCo phase (Pm
m).59 If each α-Mn atom in the cubic phase is substituted by Fe0.28Co0.72, leading to a conversion to the FeCo alloy as a function of the nominal percentage of Fe in the CoxFey phase. This rearrangement of the Fe0.28Co0.72 structure indicates that one Fe atom is centered in the body of the cubic structure with a cobalt-shell-nucleus-like building block. This formation tendency is congruent with the phase diagram of FeCo, when comparing the cubic structure of pure Fe (PDF#6-0696).60 Particularly, such a FeCo cobalt-shell-nucleus-like structure remains the same with the increase of the mass fraction of Fe, in (2) – (5) samples, cf. Fig. 3(b). The addition of Fe into Co-based catalysts could not only modify the electronic structures but also afford stronger affinity for the adsorption of oxygen species.62 The presence of the CoFe2O4 phase possibly means another transformation zone towards the Fe2O3 phase (Pm
m).
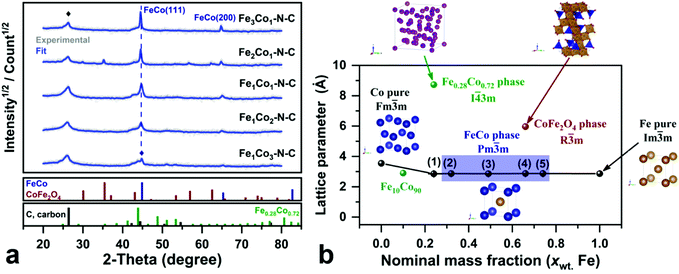 |
| Fig. 3 (a) Rietveld refinement analyses of XRD patterns of samples: FexCoy–N–C in the angle interval of 20 to 80°. The fitting parameters are on average Rwp% = 33.4, and GoF = 1.1; (b) experimental reticular parameter (a) as a function of the nominal mass fraction of Fe in FexCoy–N–C samples, (1)–(5) are Fe1Co3–N–C, Fe1Co2–N–C, Fe1Co1–N–C, Fe2Co1–N–C and Fe3Co1–N–C, respectively. Fe10Co90 is taken from a reference.61 | |
Table 1 Structural properties, mass percentage, and crystallite size of Fe-based samples
Samples |
x
wt Fe |
Phases |
Reticular parameter a/Å |
Reticular parameter c/Å |
Mass percentage phase/wt% |
Crystallite size 〈d〉/nm |
Fe1Co3–N–C |
0.24 |
FeCo |
2.8572 (0.0009) |
n. a. |
37.99 (0.0) |
34.1 (6.6) |
Fe0.28Co0.72 |
8.7329 (0.0085) |
n. a. |
62.01 (10.8) |
11.1 (1.3) |
Fe1Co2–N–C |
0.32 |
FeCo |
2.8702 (0.0007) |
n. a. |
100 |
21.1 (1.2) |
Fe1Co1–N–C |
0.49 |
FeCo |
2.8635 (0.0004) |
n. a. |
100 |
23.5 (0.7) |
Fe2Co1–N–C |
0.67 |
FeCo |
2.8752 (0.0002) |
n. a. |
77.16 (5.5) |
79.9 (3.6) |
CoFe2O4 |
5.9640 (0.0074) |
14.6097 (0.0352) |
22.84 (2.2) |
81.7 (23.4) |
Fe3Co1–N–C |
0.74 |
FeCo |
2.8758 (0.00017) |
n. a. |
100 |
170.1 (17.1) |
The microstructure and the morphology of FexCoy–N–C samples were further studied using a high-resolution transmission electron microscope (HRTEM), cf. Fig. 4 and Fig. S2–S5.† The HRTEM images evidence that the porous carbon nanosheets (NSs) entangled with bamboo-like NCNTs emerge on a Fe3Co1–N–C sample, while the black dots correspond to the FeCo alloy nanoparticles embedded in the carbon support. Fig. 4c reveals that the dark core shows a lattice fringe d-spacing of 0.20 nm ascribed to the (110) plane of FeCo nanoalloys, while CNTs composed of layered graphitic carbon with a d-spacing of 0.34 nm are observed. Moreover, the selected area electron diffraction (SAED) pattern of the Fe3Co1–N–C sample shows the rings and polycrystalline diffraction scattering dots, which are assigned to the diffraction of the graphitic carbon shell and the FeCo alloys, Fig. 4d. This fact confirms again that the FeCo nanoalloys are encapsulated in the graphitic carbon layer.
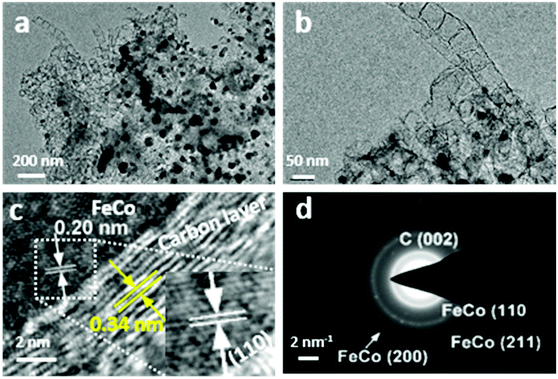 |
| Fig. 4 (a–c) HRTEM images and (d) SAED pattern of the Fe3Co1–N–C sample. | |
Fig. 5a shows the N2 adsorption–desorption isotherms of all FexCoy–N–C samples, indicating a type-IV characteristic with a H2-type hysteresis loop in the relative pressure (P/P0) range of 0.45–1.0, which is related to the interconnected and uniform channel-like mesoporous structure, the so-called “ink-bottle pores”.63 The BJH pore size distribution curves also manifest the presence of mesopores (3–4 nm) on FexCoy–N–C samples, as shown in Fig. S6.† The pore size distribution is mainly below 4 nm due to the random and loose stacking of tiny graphitic layers in the NCNTs.64 The calculated Brunauer–Emmett–Teller (BET) surface area is 282.31, 277.69, 226.83, 185.39 and 308.95 m2 g−1 for Fe1Co3–N–C, Fe1Co2–N–C, Fe1Co1–N–C, Fe2Co1–N–C and Fe3Co1–N–C samples, respectively. The mesoporous structure and large surface area of the Fe3Co1–N–C electrocatalyst favor the transport of mass/electrons certainly improving the accessibility of ORR active sites during the electrocatalytic reaction process.
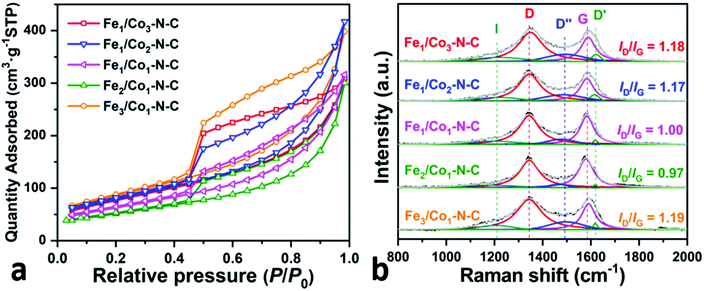 |
| Fig. 5 (a) N2 adsorption–desorption isotherms and (b) Raman spectra of the Fex/Coy–N–C samples. | |
Raman spectroscopy was carried out to investigate the chemical modifications on the CN/CNT surface. Fig. 5b contrasts the Raman spectra of all FexCoy–N–C samples at the energy interval of 800–2000 cm−1. After deconvolution, the Raman spectra display five overlapping bands, namely, I band (∼1200 cm−1), D band (∼1345 cm−1), D′′ band (∼1500 cm−1), G band (∼1585 cm−1), and D′ band (∼1617 cm−1).65 The D band is related to the symmetry breakdown of sp3 carbon atoms, structural disorders and defects, while the D′′ band is relevant to the amorphous sp2 phase. The dominant D band and the presence of D′′ band infer that all FexCoy–N–C samples have a highly disordered degree of graphitic carbon. The G band arises from the in-plane tangential stretching vibration mode of sp2 carbon atoms. The ID/IG ratio, derived from the integrated intensity of each band, is used as an indicator of the defect fraction and disordered degree in the carbonaceous materials. The ID/IG ratio of Fe1Co3–N–C and Fe3Co1–N–C samples is higher than those of the Fe1Co2–N–C, Fe1Co1–N–C and Fe2Co1–N–C, caused by the higher N-dopant content.66 An increase in the number of defects on Fe1Co3–N–C and Fe3Co1–N–C samples may result from the lattice distortion of sp2 hybrid carbon when N atoms intercalate into the carbon matrix. Besides, as summarized in Table 2, the G-band position of the Fe3Co1–N–C sample is centered at a higher wavenumber with respect to other FexCoy–N–C samples, suggesting that Fe3Co1–N–C is highly disordered.67 The relation of the in-plane crystallite size (La) and ID/IG ratio is given by eqn (5):
| 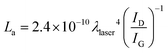 | (5) |
where
λ is the laser wavelength (532 nm). As summarized in
Table 2, it can be noticed that the in-plane crystallite size of Fe
3Co
1–N–C is the smallest one (16.13 nm), probably because of the grafting of more N-containing groups on the basal-plane surface of carbon in Fe
3Co
1–N–C.
Table 2 Pore diameter and BET surface area of all Fex/Coy–N–C samples
Samples |
Fe1/Co3–N–C |
Fe1/Co2–N–C |
Fe1/Co1–N–C |
Fe2/Co1–N–C |
Fe3/Co1–N–C |
BET surface area (m2 g−1) |
282.31 |
277.69 |
226.83 |
185.39 |
308.95 |
Pore diameter (nm) |
3.47 |
3.51 |
3.59 |
3.57 |
3.59 |
G-band position (cm−1) |
1588 |
1583 |
1583 |
1581 |
1590 |
L
a (nm) |
16.27 |
16.41 |
19.20 |
19.79 |
16.13 |
To probe the elemental composition and surface valence state of FexCoy–N–C samples, X-ray photoelectron spectroscopy (XPS) measurements were performed and are shown in Fig. 6 and S7.† The XPS survey spectra of all FexCoy–N–C samples reveal the presence of Co 2p, Fe 2p, N 1s, C 1s, and O 1s, cf. Fig. S7a.† As shown in Fig. 6a, the high-resolution Co 2p spectra present a spin–orbit coupling of Co 2p3/2 and Co 2p1/2, and their corresponding satellite peaks. After deconvolution, three signals centered at ∼778.6 eV, ∼780.5 eV and ∼783.6 eV are attributed to the metallic Co, CoOx and CoNx species, respectively.52,68 The CoNx species are possibly the result of coordination between the surrounding Co atoms, in the cubic structure with a cobalt-shell-nucleus-like building block, and N moieties. Such synergistic coupling between FeCo alloy and N-doped carbon nanotubes is proposed to enhance the electrocatalytic performance.69 One notes that the relative proportion of Co–Nx, known as highly ORR-active centers, decreases along with the increase of the Fe/Co ratio of FexCoy–N–C samples, Table 3.53 The Fe 2p3/2 spectra can be identified as Fe0 (∼707.0 eV), Fe2+ (∼708.5 eV), and Fe3+ (∼711.0 eV), respectively. Most of the Fe2+ and Fe3+ ions correspond to the Fe–Nx configuration, while a fraction of them comes from the surface oxidation of CoFe alloy nanoparticles in air.51 Fe0 originated from the FeCo alloy and metallic Fe could provide extra electrons to neighboring Fe–Nx, and increase the HOMO energy of Fe–Nx to overlap with the LUMO of triplet O2 molecules, which leads to the enhancement of ORR activity.70,71 It can be found that the Fe–Nx (Fe2+ and Fe3+) content decreases with the increasing Fe/Co ratio in the MOF precursors from 1/3 to 3/1. In contrast, the relative percentage of Fe0 increases. The deconvoluted XPS spectra of N 1s show five types of N configuration, namely, pyridinic N (398.6 eV), Co(Fe)–Nx (399.6 eV), pyrrolic N (401.1 eV), graphitic N (402.7 eV), and oxidized N (405.9 eV), respectively (Fig. 6c).38,43,68 It indicates the disruption of π conjugation on the outer layers of the carbon lattice due to nitrogen doping. Such an N-doping effect stems from the pyrolysis process of melamine at high temperatures, accompanied by the release of NH3 gas. All types of nitrogen dopants are believed to play vital roles in the improvement of ORR performance, except for the oxidized N moieties.72 Different types of N active species are reported to have different influences on the ORR performance; for example, M–Nx, pyridinic N as well as pyrrolic N contribute to improve the onset potential (Eonset), while graphitic-N could enhance the limiting diffusion current density (jL). In particular, previous literature studies reported that the enhanced ORR activity in the N-doped carbon materials containing pyridinic-type nitrogen functionalities is ascribed to the conjugation effect of the nitrogen lone pair electrons on the nitrogen and graphene π-systems.73–75 The presence of Co(Fe)–Nx species further supports that FeCo nanoparticles strongly interact with the N-doped carbon support, favoring to facilitate electron transfer from the metal atoms to CNTs, thereby accelerating the formation of the OOH species.64 Besides, in Fig. S7b,† the total content of N on Fe1Co3–N–C and Fe3Co1–N–C samples is higher than those of the Fe1Co2–N–C, Fe1Co1–N–C and Fe2Co1–N–C, in agreement with the Raman results. Such nitrogen enrichment might provide a higher density of catalytically active centers with low stereohindrance for binding redox species, followed by the complete reduction of oxygen to water via a four-electron reaction path.
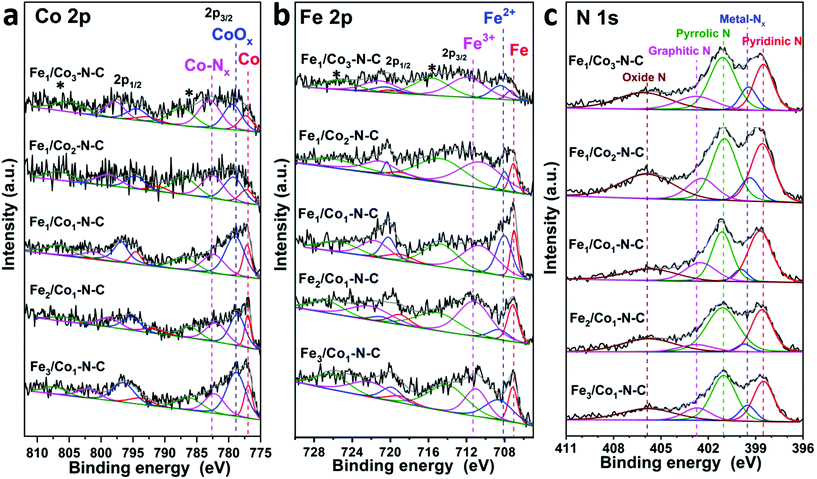 |
| Fig. 6 XPS spectra of (a) Co 2p, (b) Fe 2p, and (c) N 1s for FexCoy–N–C materials. | |
Table 3 Analysis of the Fe 2p and Co 2p photoemission lines of all Fex/Coy–N–C samples
Samples |
Fe1/Co3–N–C (at%) |
Fe1/Co2–N–C (at%) |
Fe1/Co1–N–C (at%) |
Fe2/Co1–N–C (at%) |
Fe3/Co1–N–C (at%) |
Co0 |
16.5 |
15.8 |
18.6 |
18.2 |
18.4 |
CoOx |
35.7 |
40.5 |
45.2 |
53.0 |
56.0 |
CoNx |
47.8 |
43.7 |
36.2 |
28.8 |
25.6 |
Fe0 |
8.5 |
15.1 |
17.2 |
22.4 |
21.2 |
Fe2+ |
24.6 |
11.3 |
33.2 |
12.5 |
37.4 |
Fe3+ |
66.9 |
73.6 |
49.5 |
67.1 |
41.3 |
3.1. ORR catalytic activity evaluation
The ORR electrocatalytic activity of FexCoy–N–C catalysts was evaluated in 0.1 M KOH with 20 wt% commercial Pt/C catalyst as the benchmark. Fig. 7a shows the cyclic voltammetry (CV) curves of FexCoy–N–C and Pt/C electrodes in O2 (solid line)- and Ar (dashed line)-saturated electrolytes, respectively. In contrast to Pt/C, all FexCoy–N–C catalysts display featureless quasi-rectangular voltammograms in an Ar-saturated solution, whereas the well-defined ORR cathodic peaks can be observed at ca. 0.80 V in the O2-saturated solution. The reduction peak potential (Eredox) of the Fe1Co3–N–C and Fe3Co1–N–C catalysts is 0.82 V higher than those values of Fe1Co2–N–C (0.81 V), Fe1Co1–N–C (0.79 V) and Fe2Co1–N–C (0.80 V) catalysts. The linear sweep voltammetry (LSV) curves of all FexCoy–N–C at various rotating speeds show increased diffusion current density along with increasing rotating speeds, in Fig. S8–12.† Furthermore, the Koutecky–Levich (K–L) plots at different electrode potentials display linear and parallel lines indicating an ORR first-order reaction kinetics on FexCoy–N–C electrodes, with respect to the concentration of dissolved O2.
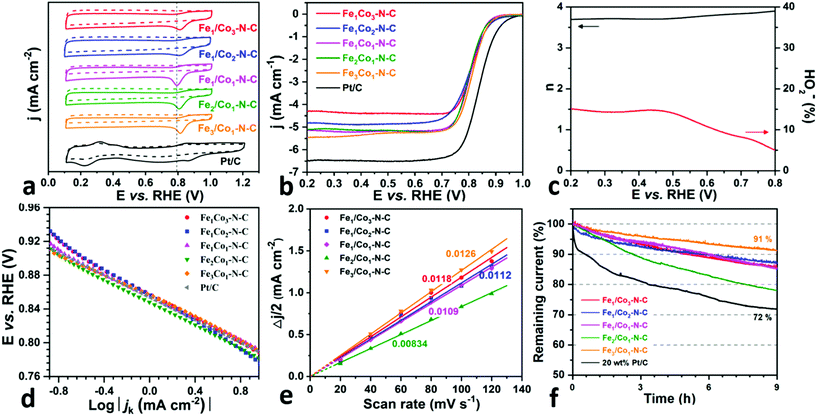 |
| Fig. 7 (a) Cyclic voltammetry curves at a scan rate of 50 mV s−1 in Ar (dashed line) and 0.1 M O2-saturated (solid line) KOH; (b) linear sweep voltammograms of electrocatalysts at 5 mV s−1 and 1600 rpm in 0.1 M O2-saturated KOH; (c) electron transfer number (n) and peroxide (H2O2) yield of Fe3Co1–N–C; (d) ORR Tafel plots derived from the K–L equation; (e) the Cdl calculation and (f) chronoamperometry (i–t) curves at 0.70 V vs. RHE of all FexCoy–N–C and Pt/C catalysts. | |
Fig. 7b depicts the linear sweep voltammetry (LSV) curves recorded at 1600 rpm of all FexCoy–N–C and Pt/C electrocatalysts. The onset potentials, Eonset, of FexCoy–N–C catalysts are nearly the same, but are negatively related to that of Pt/C. In terms of the half-wave potential (E1/2) and kinetic current density at 0.8 V, a series of FexCoy–N–C catalysts show no significant difference, cf. Table 4, which might be the result of the same cubic structure. The RRDE measurement was conducted to further analyze the electron transfer number (n) and the H2O2 yield. It is found that the H2O2 yield of Fe1/Co3–N–C and Fe3Co1–N–C catalysts is around 15% in the potential range of 0.2 to 0.7 V, lower than those of other FexCoy–N–C catalysts, Fig. 7c and S8–11.† The calculated electron transfer number (n) is >3.7, which suggests that the ORR on both the Fe1/Co3–N–C and Fe3Co1–N–C proceeds, via a nearly 4-electron pathway. Moreover, the Tafel slopes at the low overpotential region, in Fig. 7d, of Fe1/Co3–N–C and Fe3Co1–N–C catalysts display small values of 65.62 and 59.93 mV dec−1 with high ORR kinetics current density, respectively, lower than those of Fe1Co2–N–C (74.85 mV dec−1), Fe1Co1–N–C (77.62 mV dec−1), Fe2Co1–N–C (68.48 mV dec−1). It is worth noting that the ORR performance of Fe1Co3–N–C and Fe3Co1–N–C is comparable to that of some reported FeCo supported on N-doped carbon materials, for instance, CoFe/N–GCT,41 N–C–CoFe,42 Fe–Co–N–C,23 and CoFe/NC–0.2-900,45 see Table S1.†
Table 4 ORR activity parameters of all Fex/Coy–N–C and Pt/C catalysts
Samples |
E
onset/V |
E
1/2/V |
j
k@0.8 V/mA cm−2 |
Tafel slope/mV dec−1 |
Fe1/Co3–N–C |
0.87 |
0.81 |
−6.98 |
67 |
Fe1/Co2–N–C |
0.87 |
0.81 |
−6.39 |
75 |
Fe1/Co1–N–C |
0.87 |
0.80 |
−5.18 |
78 |
Fe2/Co1–N–C |
0.85 |
0.80 |
−5.06 |
68 |
Fe3/Co1–N–C |
0.87 |
0.82 |
−6.81 |
60 |
Pt/C |
0.90 |
0.84 |
−17.02 |
67 |
The electrochemically active surface area (ECSA) of the catalyst is strongly associated with the site density and the double layer capacitance (Cdl). The Cdl values of all FexCoy–N–C samples were evaluated by the double-layer charging/discharging curves at different scan rates in the non-faradaic potential range of 0.31–0.41 V, Fig. S13.† The plots of the current density Δj = (ja − jc)/2 at 0.36 V (ja and jc are the anodic and cathodic current density, respectively) against scan rates show a linear relationship and the corresponding slope value is related to Cdl, see Fig. 7e. One notes that Fe3Co1–N–C has the largest Cdl of 12.6 mF cm−1 among all FexCoy–N–C catalysts, thus confirming again that the high ORR electrochemical activity occurs on the Fe3Co1–N–C catalyst. This fact signifies that the Fe3Co1–N–C exposes more electro-active sites due to its abundant interconnected NCNT networks, which is consistent with the results of the BET surface area. Besides, in Fig. 7f, the high remaining current density over 9 h of continuous operation at 0.7 V suggests that the FexCoy–N–C catalysts display better durability for the ORR compared with the commercial Pt/C catalyst. Importantly, the Fe3Co1–N–C shows a near-90% current retention, higher than those for other FexCoy–N–C samples. Only a slight decrease (12 mV) in E1/2 is observed after 5000 cycles (Fig. S14†), which suggest a good stability of the Fe3Co1–N–C catalyst.
The improved ORR performance (activity, durability and stability) of Fe1Co3–N–C and Fe3Co1–N–C complex composites can be ascribed to the following reasons: (i) the abundance of M–Nx ORR active sites (CoNx) on the Fe1Co3–N–C catalyst is responsible for the enhanced ORR activity, and the strong interaction between the metal centers and N-doped carbon support, favoring to facilitate electron transfer from N-doped carbon to the Fermi level of FeCo alloys, and hence leading to a higher electronic density and efficient ORR kinetics; (ii) the synergistic effect between the FeCo alloy and N-doped carbon could enhance the electrocatalytic performance; (iii) the nitrogen-doped carbon not only effectively participates in the ORR process in terms of various N moieties like pyridinic-N, graphitic-N and pyrrolic-N, but also can protect the FeCo nanoalloys, preventing them from being leached out under the oxidative alkaline conditions with respect to the long-term stability/durability; and (iv) particularly, in Fe3Co1–N–C, large surface area, porous structure and abundant NCNTs greatly aid to expose more active sites and improve availability of catalytic centers.
4. Conclusion
In summary, we reported an in situ pyrolysis strategy to fabricate high-performance FeCo bimetallic MOF-derived FexCoy–N–C catalysts for the ORR in alkaline medium. The FexCoy–N–C materials with tunable morphology and chemical composition as well as pore structure mainly depend on the molar ratio of Fe3+/Co2+. Due to the confinement effect of MOFs, melamine can be effectively transformed into uniformly bamboo-like N-doped CNTs tangled with N-doped carbon nanosheets, while the obtained FeCo nanoalloys are encapsulated in the porous carbon matrix. The optimized Fe1Co3–N–C and Fe3Co1–N–C catalysts exhibit excellent ORR activity comparable to the commercial Pt/C catalyst but superior long-term stability. The formation of M–Nx (Fe–Nx and Co–Nx) species, N-doped carbon and varied N moieties (pyridinic-N, graphitic-N and pyrrolic-N), as well as the synergistic effect between FeCo alloy and N-doped carbon, is responsible for the high ORR performance. This work opens a new avenue to develop promising electrocatalysts for the ORR in alkaline medium.
Conflicts of interest
There are no conflicts to declare.
Acknowledgements
This work is supported by the National Natural Science Foundation of China, the Programme XU Guangqi (Code Projet: 45644RC), and the Fundamental Research Funds for the Central Universities (JD1716, 12060093063). H. H. Zhong specially acknowledges the financial support from the China Scholarship Council. Xiao-Wei Song thanks the University Natural Science Research Project of Anhui Province (grant number KJ2019A0549) and the talent introduction fund of Anqing Normal University (grant number 150002019) for financial support.
References
- N. S. Lewis and D. G. Nocera, Powering the Planet: Chemical Challenges in Solar Energy Utilization, Proc. Natl. Acad. Sci. U. S. A., 2006, 103, 15729–15735 CrossRef CAS.
- R. Bashyam and P. Zelenay, A Class of Non-precious Metal Composite Catalysts for Fuel Cells, Nature, 2006, 443, 63–66 CrossRef CAS.
- L. Yang, X. Zeng, W. Wang and D. Cao, Recent Progress in MOF-derived, Heteroatom-doped Porous Carbons as highly efficient electrocatalysts for oxygen reduction reaction in fuel cells, Adv. Funct. Mater., 2018, 28, 1704537–1704557 CrossRef.
- C. A. Campos-Roldán, H. Zhong, S. M. Unni, R. d. G. González-Huerta, Y. Feng and N. Alonso-Vante, Unitized Regenerative Alkaline Microfluidic Cell Based on Platinum Group Metal-Free Electrode Materials, ACS Appl. Energy Mater., 2020, 3(8), 7397–7403 CrossRef.
- Y. Qian, I. A. Khan and D. Zhao, Electrocatalysts Derived from Metal–Organic Frameworks for Oxygen Reduction and Evolution Reactions in Aqueous Media, Small, 2017, 13, 1701143 CrossRef.
- V. Nallathambi, J.-W. Lee, S. P. Kumaraguru, G. Wu and B. N. Popov, Development of High Performance Carbon Composite Catalyst for Oxygen Reduction Reaction in PEM Proton Exchange Membrane Fuel Cells, J. Power Sources, 2008, 183, 34–42 CrossRef CAS.
- Y. Feng, A. Gago, L. Timperman and N. Alonso-Vante, Chalcogenide Metal Centers for Oxygen Reduction Reaction: Activity and Tolerance, Electrochim. Acta, 2011, 56, 1009–1022 CrossRef CAS.
- M. Nesselberger, S. Ashton, J. C. Meier, I. Katsounaros, K. J. J. Mayrhofer and M. Arenz, The Particle Size Effect on the Oxygen Reduction Reaction Activity of Pt Catalysts: Influence of Electrolyte and Relation to Single Crystal Models, J. Am. Chem. Soc., 2011, 133, 17428–17433 CrossRef CAS.
- M. Shao, Q. Chang, J.-P. Dodelet and R. Chenitz, Recent Advances in Electrocatalysts for Oxygen Reduction Reaction, Chem. Rev., 2016, 116, 3594–3657 CrossRef CAS.
- B. You, N. Jiang, M. Sheng, W. S. Drisdell, J. Yano and Y. Sun, Bimetal–Organic Framework Self-Adjusted Synthesis of Support-Free Nonprecious Electrocatalysts for Efficient Oxygen Reduction, ACS Catal., 2015, 5, 7068–7076 CrossRef CAS.
- Y. Ye, F. Cai, C. Yan, Y. Li, G. Wang and X. Bao, Two-Step Pyrolysis of ZIF-8 Functionalized with Ammonium Ferric Citrate for Efficient Oxygen Reduction Reaction, J. Energy Chem., 2017, 26, 1174–1180 CrossRef.
- M. Xiao, J. Zhu, L. Ma, Z. Jin, J. Ge, X. Deng, Y. Hou, Q. He, J. Li, Q. Jia, S. Mukerjee, R. Yang, Z. Jiang, D. Su, C. Liu and W. Xing, Microporous Framework Induced Synthesis of Single-Atom Dispersed Fe-N-C Acidic ORR Catalyst and Its in Situ Reduced Fe-N4 Active Site Identification Revealed by X-ray Absorption Spectroscopy, ACS Catal., 2018, 8, 2824–2832 CrossRef CAS.
- F. Xiao, G.-L. Xu, C.-J. Sun, M. Xu, W. Wen, Q. Wang, M. Gu, S. Zhu, Y. Li, Z. Wei, X. Pan, J. Wang, K. Amine and M. Shao, Nitrogen-Coordinated Single Iron Atom Catalysts Derived from Metal Organic Frameworks for Oxygen Reduction Reaction, Nano Energy, 2019, 61, 60–68 CrossRef CAS.
- X. Xu, Z. Xia, X. Zhang, R. Sun, X. Sun, H. Li, C. Wu, J. Wang, S. Wang and G. Sun, Atomically Dispersed Fe-N-C Derived from Dual Metal-Organic Frameworks as Efficient Oxygen Reduction Electrocatalysts in Direct Methanol Fuel Cells, Appl. Catal., B, 2019, 259, 118042 CrossRef CAS.
- J.-D. Yi, R. Xu, Q. Wu, T. Zhang, K.-T. Zang, J. Luo, Y.-L. Liang, Y.-B. Huang and R. Cao, Atomically Dispersed Iron–Nitrogen Active Sites within Porphyrinic Triazine-Based Frameworks for Oxygen Reduction Reaction in Both Alkaline and Acidic Media, ACS Energy Lett., 2018, 3, 883–889 CrossRef CAS.
- J.-D. Yi, R. Xu, G.-L. Chai, T. Zhang, K. Zang, B. Nan, H. Lin, Y.-L. Liang, J. Lv, J. Luo, R. Si, Y.-B. Huang and R. Cao, Cobalt Single-atoms Anchored on Porphyrinic Triazine-based Frameworks as Bifunctional Electrocatalysts for Oxygen Reduction and Hydrogen Evolution Reactions, J. Mater. Chem. A, 2019, 7, 1252–1259 RSC.
- S. Li, L. Zhang, J. Kim, M. Pan, Z. Shi and J. Zhang, Synthesis of Carbon-Supported Binary FeCo–N Non-Noble Metal Electrocatalysts for the Oxygen Reduction Reaction, Electrochim. Acta, 2010, 55, 7346–7353 CrossRef CAS.
- G. Wu, K. L. More, C. M. Johnston and P. Zelenay, High-Performance Electrocatalysts for Oxygen Reduction Derived from Polyaniline, Iron, and Cobalt, Science, 2011, 332, 443 CrossRef CAS.
- Z. Zhang, M. Dou, H. Liu, L. Dai and F. Wang, A Facile Route to Bimetal and Nitrogen-Codoped 3D Porous Graphitic Carbon Networks for Efficient Oxygen Reduction, Small, 2016, 12, 4193–4199 CrossRef CAS.
- J. Wang, Z. Huang, W. Liu, C. Chang, H. Tang, Z. Li, W. Chen, C. Jia, T. Yao, S. Wei, Y. Wu and Y. Li, Design of N-Coordinated Dual-Metal Sites: A Stable and Active Pt-Free Catalyst for Acidic Oxygen Reduction Reaction, J. Am. Chem. Soc., 2017, 139, 17281–17284 CrossRef CAS.
- B. Y. Guan, Y. Lu, Y. Wang, M. Wu and X. W. Lou, Porous Iron–Cobalt Alloy/Nitrogen-Doped Carbon Cages Synthesized via Pyrolysis of Complex Metal–Organic Framework Hybrids for Oxygen Reduction, Adv. Funct. Mater., 2018, 28, 1706738 CrossRef.
- Y. Q. Jin, Z. Lin, R. Zhong, J. Huang, G. Liang, J. Li, Y. Jin and H. Meng, Cobalt Iron Carbonate Hydroxide Hydrate on 3D Porous Carbon as Active and Stable Bifunctional Oxygen Electrode for Zn–air Battery, J. Power Sources, 2018, 402, 388–393 CrossRef CAS.
- L. Osmieri, C. Zafferoni, L. Wang, A. H. A. M. Videla, A. Lavacchi and S. Specchia, Polypyrrole-Derived Fe−Co−N−C Catalyst for the Oxygen Reduction Reaction: Performance in Alkaline Hydrogen and Ethanol Fuel Cells, ChemElectroChem, 2018, 5, 1954–1965 CrossRef CAS.
- M. Tan, T. He, J. Liu, H. Wu, Q. Li, J. Zheng, Y. Wang, Z. Sun, S. Wang and Y. Zhang, Supramolecular Bimetallogels: A Nanofiber Network for Bimetal/Nitrogen Co-doped Carbon Electrocatalysts, J. Mater. Chem. A, 2018, 6, 8227–8232 RSC.
- M. Wu, Q. Wei, G. Zhang, J. Qiao, M. Wu, J. Zhang, Q. Gong and S. Sun, Fe/Co Double Hydroxide/Oxide Nanoparticles on N-Doped CNTs as Highly Efficient Electrocatalyst for Rechargeable Liquid and Quasi-Solid-State Zinc–Air Batteries, Adv. Energy Mater., 2018, 8, 1801836 CrossRef.
- P. Zhu, J. Gao and S. Liu, Facile in Situ Coupling CoFe/Co Nanoparticles and N-doped Carbon Nanotubes/Graphitic Nanosheets as Bifunctional Oxygen Electrocatalysts for Rechargeable Zn-air Batteries, J. Power Sources, 2020, 449, 227512 CrossRef CAS.
- Y. Wang, T. Hu, Y. Qiao and Y. Chen, Synergistic Engineering of Defects and Architecture in CoFe@NC toward Highly Efficient Oxygen Electrode Reactions, Int. J. Hydrogen Energy, 2020, 45, 8686–8694 CrossRef CAS.
- Q. Lin, X. Bu, A. Kong, C. Mao, F. Bu and P. Feng, Heterometal-Embedded Organic Conjugate Frameworks
from Alternating Monomeric Iron and Cobalt Metalloporphyrins and Their Application in Design of Porous Carbon Catalysts, Adv. Mater., 2015, 27, 3431–3436 CrossRef CAS.
- T. Palaniselvam, V. Kashyap, S. N. Bhange, J.-B. Baek and S. Kurungot, Nanoporous Graphene Enriched with Fe/Co-N Active Sites as a Promising Oxygen Reduction Electrocatalyst for Anion Exchange Membrane Fuel Cells, Adv. Funct. Mater., 2016, 26, 2150–2162 CrossRef CAS.
- S. Li, C. Cheng, X. Zhao, J. Schmidt and A. Thomas, Active Salt/Silica-Templated 2D Mesoporous FeCo-Nx-Carbon as Bifunctional Oxygen Electrodes for Zinc–Air Batteries, Angew. Chem., Int. Ed., 2018, 57, 1856–1862 CrossRef CAS.
- J. Wang, W. Liu, G. Luo, Z. Li, C. Zhao, H. Zhang, M. Zhu, Q. Xu, X. Wang, C. Zhao, Y. Qu, Z. Yang, T. Yao, Y. Li, Y. Lin, Y. Wu and Y. Li, Synergistic Effect of Well-Defined Dual Sites Boosting the Oxygen Reduction Reaction, Energy Environ. Sci., 2018, 11, 3375–3379 RSC.
- D. Zhang, W. Chen, Z. Li, Y. Chen, L. Zheng, Y. Gong, Q. Li, R. Shen, Y. Han, W.-C. Cheong, L. Gu and Y. Li, Isolated Fe and Co Dual Active Sites on Nitrogen-Doped Carbon for A Highly Efficient Oxygen Reduction Reaction, Chem. Commun., 2018, 54, 4274–4277 RSC.
- H. Zhong, L. A. Estudillo-Wong, Y. Gao, Y. Feng and N. Alonso-Vante, Cobalt-Based Multicomponent Oxygen Reduction Reaction Electrocatalysts Generated by Melamine Thermal Pyrolysis with High Performance in an Alkaline Hydrogen/Oxygen Microfuel Cell, ACS Appl. Mater. Interfaces, 2020, 12, 21605–21615 CrossRef CAS.
- S. H. Lim, Z. Li, C. K. Poh, L. Lai and J. Lin, Highly Active Non-Precious Metal Catalyst Based on Poly(vinylpyrrolidone)–Wrapped Carbon Nanotubes Complexed with Iron–Cobalt Metal Ions for Oxygen Reduction Reaction, J. Power Sources, 2012, 214, 15–20 CrossRef CAS.
- P. Cai, Y. Hong, S. Ci and Z. Wen, In Situ Integration of CoFe Alloy Nanoparticles with Nitrogen-Doped Carbon Nanotubes as Advanced Bifunctional Cathode Catalysts for Zn–air Batteries, Nanoscale, 2016, 8, 20048–20055 RSC.
- S. H. Noh, M. H. Seo, J. Kang, T. Okajima, B. Han and T. Ohsaka, Towards A Comprehensive Understanding of FeCo Coated with N-doped Carbon as A Stable Bi-Functional Catalyst in Acidic Media, NPG Asia Mater., 2016, 8, e312 CrossRef CAS.
- R. Bose, T.-H. Kim, B. Koh, C.-Y. Jung and S. C. Yi, Influence of Phosphidation on CoSe2 Catalyst for Hydrogen Evolution Reaction, ChemistrySelect, 2017, 2, 10661–10667 CrossRef CAS.
- H. Wang, F. Yin, P. Lv, T. Fan, X. He and B. Chen, Metal–Organic-Framework-Derived FeCo Alloy Core@Nitrogen-Doped Carbon Shell Nanoparticles Anchored on Carbon Nanotubes for Rechargeable LiO2 Battery, Int. J. Hydrogen Energy, 2017, 42, 2127–2133 CrossRef CAS.
- N. Wu, Y. Lei, Q. Wang, B. Wang, C. Han and Y. Wang, Facile Synthesis of FeCo@NC Core–shell Nanospheres Supported on Graphene as An Efficient Bifunctional Oxygen Electrocatalyst, Nano Res., 2017, 10, 2332–2343 CrossRef CAS.
- L. An, N. Jiang, B. Li, S. Hua, Y. Fu, J. Liu, W. Hao, D. Xia and Z. Sun, A Highly Active and Durable Iron/Cobalt Alloy Catalyst Encapsulated in N-doped Graphitic Carbon Nanotubes for Oxygen Reduction Reaction by A Nanofibrous Dicyandiamide Template, J. Mater. Chem. A, 2018, 6, 5962–5970 RSC.
- X. Liu, L. Wang, P. Yu, C. Tian, F. Sun, J. Ma, W. Li and H. Fu, A Stable Bifunctional Catalyst for Rechargeable Zinc–Air Batteries: Iron–Cobalt Nanoparticles
Embedded in a Nitrogen-Doped 3D Carbon Matrix, Angew. Chem., Int. Ed., 2018, 57, 16166–16170 CrossRef CAS.
- A. Samanta and C. R. Raj, Catalyst Support in Oxygen Electrocatalysis: A Case Study with CoFe Alloy Electrocatalyst, J. Phys. Chem. C, 2018, 122, 15843–15852 CrossRef CAS.
- S. Sultan, J. N. Tiwari, J.-H. Jang, A. M. Harzandi, F. Salehnia, S. J. Yoo and K. S. Kim, Highly Efficient Oxygen Reduction Reaction Activity of Graphitic Tube Encapsulating Nitrided CoxFey Alloy, Adv. Energy Mater., 2018, 8, 1801002 CrossRef.
- C. Li, M. Wu and R. Liu, High-Performance Bifunctional Oxygen Electrocatalysts for Zinc-Air Batteries over Mesoporous Fe/Co-N-C Nanofibers with Embedding FeCo Alloy Nanoparticles, Appl. Catal., B, 2019, 244, 150–158 CrossRef CAS.
- Z. Du, P. Yu, L. Wang, C. Tian, X. Liu, G. Zhang and H. Fu, Cubic Imidazolate Frameworks-Derived CoFe Alloy Nanoparticles-Embedded N-doped Graphitic Carbon for Discharging Reaction of Zn-air Battery, Sci. China Mater., 2020, 63, 327–338 CrossRef CAS.
- X. Luo, H. Ren, H. Ma, C. Yin, Y. Wang, X. Li, Z. Shen, Y. Wang and L. Cui, In Situ Integration of Co5.47N and Co0.72Fe0.28 Alloy Nanoparticles into Intertwined Carbon Network for Efficient Oxygen Reduction, J. Colloid Interface Sci., 2020, 569, 267–276 CrossRef CAS.
- K. Li, Y. Li, W. Peng, G. Zhang, F. Zhang and X. Fan, Bimetallic Iron–Cobalt Catalysts and Their Applications in Energy-Related Electrochemical Reactions, Catalysts, 2019, 9, 762 CrossRef CAS.
- J.-S. Li, S.-L. Li, Y.-J. Tang, M. Han, Z.-H. Dai, J.-C. Bao and Y.-Q. Lan, Nitrogen-Doped Fe/Fe3C@Graphitic Layer/Carbon Nanotube Hybrids Derived from MOFs: Efficient Bifunctional Electrocatalysts for ORR and OER, Chem. Commun., 2015, 51, 2710–2713 RSC.
- Q. Wang, Y. Lei, Z. Chen, N. Wu, Y. Wang, B. Wang and Y. Wang, Fe/Fe3C@C Nanoparticles Encapsulated in N-doped Graphene–CNTs Framework as An Efficient Bifunctional Oxygen Electrocatalyst for Robust Rechargeable Zn–Air Batteries, J. Mater. Chem. A, 2018, 6, 516–526 RSC.
- J.-T. Ren, L. Chen, Y.-S. Wang, W.-W. Tian, L.-J. Gao and Z.-Y. Yuan, FeNi Nanoalloys Encapsulated in N-Doped CNTs Tangled with N-Doped Carbon Nanosheets as Efficient Multifunctional Catalysts for Overall Water Splitting and Rechargeable Zn–Air Batteries, ACS Sustainable Chem. Eng., 2020, 8, 223–237 CrossRef CAS.
- Q. Lai, L. Zheng, Y. Liang, J. He, J. Zhao and J. Chen, Metal–Organic-Framework-Derived Fe-N/C Electrocatalyst with Five-Coordinated Fe-Nx Sites for Advanced Oxygen Reduction in Acid Media, ACS Catal., 2017, 7, 1655–1663 CrossRef CAS.
- H. Zhong, Y. Luo, S. He, P. Tang, D. Li, N. Alonso-Vante and Y. Feng, Electrocatalytic Cobalt Nanoparticles Interacting with Nitrogen-doped Carbon Nanotube in Situ Generated from A Metal-organic Framework for the Oxygen Reduction Reaction, ACS Appl. Mater. Interfaces, 2017, 9, 2541–2549 CrossRef CAS.
- S. G. Peera, J. Balamurugan, N. H. Kim and J. H. Lee, Sustainable Synthesis of Co@NC Core Shell Nanostructures from Metal Organic Frameworks via Mechanochemical Coordination Self-Assembly: An Efficient Electrocatalyst for Oxygen Reduction Reaction, Small, 2018, 14, 1800441–1800455 CrossRef.
- M. Zhong, D. H. Yang, L. J. Kong, W. Shuang, Y. H. Zhang and X. H. Bu, Bimetallic Metal-Organic Framework Derived Co3O4-CoFe2O4 Composites with Different Fe/Co Molar Ratios as Anode Materials for Lithium Ion Batteries, Dalton Trans., 2017, 46, 15947–15953 RSC.
- Y. Chi, W. Yang, Y. Xing, Y. Li, H. Pang and Q. Xu, Ni/Co Bimetallic Organic Framework Nanosheet Assemblies for High-Performance Electrochemical Energy Storage, Nanoscale, 2020, 12, 10685–10692 RSC.
- X. Li, W. Guo, Z. Liu, R. Wang and H. Liu, Fe-based MOFs for Efficient Adsorption and Degradation of Acid Orange 7 in Aqueous Solution via Persulfate Activation, Appl. Surf. Sci., 2016, 369, 130–136 CrossRef CAS.
- T. Mühge, T. Zeidler, Q. Wang, C. Morawe, N. Metoki and H. Zabel, Structural and Magnetic Studies of FexCo1−x(001) Alloy Films on MgO(001) Substrates, J. Appl. Phys., 1995, 77, 1055–1060 CrossRef.
- L. A. Estudillo-Wong, G. Ramos-Sanchez, L. Calvillo, G. Granozzi and N. Alonso-Vante, Support Interaction Effect of Platinum Nanoparticles on Non-, Y-, Ce-Doped Anatase and Its Implication on the ORR in Acid and Alkaline Media, ChemElectroChem, 2017, 4, 3264–3275 CrossRef CAS.
- G. Pourroy, S. Läkamp and S. Vilminot, Stabilization of iron-cobalt alloy isomorphous of α-Mn in a metal ferrite composite, J. Alloys Compd., 1996, 244, 90–93 CrossRef CAS.
-
H. Okamoto, M. E. Schlesinger and E. M. Mueller, ASM Handbook Volume 3: Alloy Phase Diagrams, ASM International, USA, 1st edn, 1992 Search PubMed.
- F. Sánchez-De Jesús, A. M. Bolarín-Miró, C. A. Cortés Escobedo, G. Torres-Villaseñor and P. Vera-Serna, Structural Analysis and Magnetic Properties of FeCo Alloys Obtained by Mechanical Alloying, J. Metall., 2016, 2016, 8347063 Search PubMed.
- H. Wang, F. Yin, P. Lv, T. Fan, X. He and B. Chen, Metal–organic-framework-derived FeCo alloy core@nitrogen-doped carbon shell nanoparticles anchored on carbon nanotubes for rechargeable Li-O-2 battery, Int. J. Hydrogen Energy, 2017, 42, 2127–2133 CrossRef CAS.
- M. Thommes, K. Kaneko, A. V. Neimark, J. P. Olivier, F. Rodriguez-Reinoso, J. Rouquerol and K. S. W. Sing, Physisorption of Gases, with Special Reference to the Evaluation of Surface Area and Pore Size Distribution (IUPAC Technical Report), Pure Appl. Chem., 2015, 87, 1051–1069 CAS.
- J. Meng, C. Niu, L. Xu, J. Li, X. Liu, X. Wang, Y. Wu, X. Xu, W. Chen, Q. Li, Z. Zhu, D. Zhao and L. Mai, General Oriented Formation of Carbon Nanotubes from Metal-Organic Frameworks, J. Am. Chem. Soc., 2017, 139, 8212–8221 CrossRef CAS.
- Y. Cao, H. Yu, J. Tan, F. Peng, H. Wang, J. Li, W. Zheng and N.-B. Wong, Nitrogen-, Phosphorous- and Boron-Doped Carbon Nanotubes As Catalysts for the Aerobic Oxidation of Cyclohexane, Carbon, 2013, 57, 433–442 CrossRef CAS.
- Y. Hou, Z. Wen, S. Cui, S. Ci, S. Mao and J. Chen, An Advanced Nitrogen-Doped Graphene/Cobalt-Embedded Porous Carbon Polyhedron Hybrid for Efficient Catalysis of Oxygen Reduction and Water Splitting, Adv. Funct. Mater., 2015, 25, 872–882 CrossRef CAS.
- A. Cuesta, P. Dhamelincourt, J. Laureyns, A. Martínez-Alonso and J. M. D. Tascón, Raman Microprobe Studies on Carbon Materials, Carbon, 1994, 32, 1523–1532 CrossRef CAS.
- Y.-Z. Chen, C. Wang, Z.-Y. Wu, Y. Xiong, Q. Xu, S.-H. Yu and H.-L. Jiang, From Bimetallic Metal-Organic Framework to Porous Carbon: High Surface Area and Multicomponent Active Dopants for Excellent Electrocatalysis, Adv. Mater., 2015, 27, 5010–5016 CrossRef CAS.
- C.-Y. Su, H. Cheng, W. Li, Z.-Q. Liu, N. Li, Z. Hou, F.-Q. Bai, H.-X. Zhang and T.-Y. Ma, Atomic Modulation of FeCo-Nitrogen-Carbon Bifunctional Oxygen Electrodes for Rechargeable and Flexible All-Solid-State Zinc-Air Battery, Adv. Energy Mater., 2017, 7, 1602420 CrossRef.
- W. J. Jiang, L. Gu, L. Li, Y. Zhang, X. Zhang, L. J. Zhang, J. Q. Wang, J. S. Hu, Z. Wei and L. J. Wan, Understanding the High Activity of Fe-N-C Electrocatalysts in Oxygen Reduction: Fe/Fe3C Nanoparticles Boost the Activity of Fe-N(x), J. Am. Chem. Soc., 2016, 138, 3570–3578 CrossRef CAS.
- Q. Wang, Y. Lei, Z. Chen, N. Wu, Y. Wang, B. Wang and Y. Wang, Fe/Fe3C@C nanoparticles encapsulated in N-doped graphene–CNTs framework as an efficient bifunctional oxygen electrocatalyst for robust rechargeable Zn–air batteries, J. Mater. Chem. A, 2018, 6, 516–526 RSC.
- P.-C. Shi, J.-D. Yi, T.-T. Liu, L. Li, L.-J. Zhang, C.-F. Sun, Y.-B. Wang, Y.-B. Huang and R. Cao, Hierarchically Porous Nitrogen-doped Carbon Nanotubes Derived from Core–shell ZnO@zeolitic Imidazolate Framework Nanorods for Highly Efficient Oxygen Reduction Reactions, J. Mater. Chem. A, 2017, 5, 12322–12329 RSC.
- C. V. Rao, C. R. Cabrera and Y. Ishikawa, In Search of the Active Site in Nitrogen-Doped Carbon Nanotube Electrodes for the Oxygen Reduction Reaction, J. Phys. Chem. Lett., 2010, 1, 2622–2627 CrossRef CAS.
- S. Maldonado and K. J. Stevenson, Influence of Nitrogen Doping on Oxygen Reduction Electrocatalysis at Carbon Nanofiber Electrodes, J. Phys. Chem. B, 2005, 109, 4707–4716 CrossRef CAS.
- S. K. Singh, K. Takeyasu and J. Nakamura, Active Sites and Mechanism of Oxygen Reduction Reaction Electrocatalysis on Nitrogen-Doped Carbon Materials, Adv. Mater., 2019, 31, 1804297–1804313 CrossRef.
Footnote |
† Electronic supplementary information (ESI) available: Physicochemical characterization details for all FexCoy–N–C presented herein including, but not limited to, ICP-AES, HRTEM, XPS, pore diameter size distribution curves, ORR–LSV curves, peroxide yields, electron transfer numbers, electrochemical double-layer capacitance, and accelerated stability tests. See DOI: 10.1039/d0qi01037e |
|
This journal is © the Partner Organisations 2021 |
Click here to see how this site uses Cookies. View our privacy policy here.