DOI:
10.1039/D0QI00954G
(Research Article)
Inorg. Chem. Front., 2021,
8, 79-89
“Double guarantee mechanism” of Ca2+-intercalation and rGO-integration ensures hydrated vanadium oxide with high performance for aqueous zinc-ion batteries†
Received
7th August 2020
, Accepted 21st October 2020
First published on 22nd October 2020
Abstract
Aqueous rechargeable zinc-ion batteries (ARZIBs) are widely considered to be potential energy storage devices because of their low toxicity, low cost and environment-friendliness. Recent studies have proved that hydrated vanadium oxides are significant cathode materials for ARZIBs. However, their low specific capacity and poor cycling stability limit their further development because of the structural instability of the resulting device. In this work, we developed a “double guarantee mechanism” composite of Ca2+-intercalated hydrated vanadium oxide (V2O5·nH2O, abbreviated as VOH) integrated with reduced graphene oxide (rGO), denoted as CaVOH/rGO, via a facile hydrothermal process and subsequent freeze-drying method. The inserted Ca2+ expanded the layer spacing, greatly reduced the electrostatic interactions and increased the reversibility of the vanadium oxide, while the integrated graphene improved the conductivity and made the composite material stable during the discharge/charge process with outstanding electrochemical performances. The CaVOH/rGO//Zn battery delivered an exceptional specific capacity of 409 mA h g−1 at 0.05 A g−1. It also exhibited an admirable capacity retention of more than 90% (299 mA h g−1) after 2000 cycles at 4.0 A g−1 and an impressive energy density (381 W h kg−1 at 48 W kg−1). To determine the main reaction mechanisms of Zn2+ reversible (de)intercalation, we employed multiple ex situ analytical methods to reveal the process of Zn2+ storage. The results illustrated that the CaVOH/rGO three-element composite has marvelous potential as a cathode material, and this work provides a novel method to enhance the electrochemical properties of V2O5·nH2O.
1. Introduction
Over the past few decades, lithium-ion batteries as energy storage systems have become common in daily life as smart grids, electronic watches, etc. However, due to the scarcity and high cost of lithium resources, this type of battery cannot meet the growing energy density requirements, and also causes environmental pollution and other safety problems in the process of its production and recovery.1–7 Thus, recently, aqueous rechargeable multivalent metal ion batteries, including Mg2+, Zn2+, Ca2+, and Al3+ batteries, have attracted wide attention because of their low toxicity and environmental friendliness.8–13 The energy storage in multivalent metal ion batteries can be improved due to the transfer of multiple electrons. Among the multivalent ion batteries, aqueous rechargeable zinc ion batteries (ARZIBs) have attracted huge interest because the Zn anode has great advantages, such as high capacity (up to 820 mA h g−1 theoretically), high density (5851 mA h mL−1), low cost and low redox potential.14–21 However, the lack of appropriate intercalation cathodes to make the structure of Zn2+ stable during the rapid (de)intercalation process has greatly hindered the development of ARZIBs. Therefore, exploiting new cathodes has become the primary task.14
Thus far, some cathode materials for ARZIBs have been developed,22,23 including manganese-based materials, Prussian blue and its analogues, and vanadium oxides. Benefiting from their low cost, green nature and low pollution, different crystal structures of manganese dioxide have been studied, such as α-MnO2, δ-MnO2, and γ-MnO2. However, the initial capacity of the corresponding batteries decreases dramatically due to their tunnel structure, and their performances are extremely poor at high current density.24–27 Prussian blue and its analogues are non-toxic, cheap and easy to synthesize. Their open framework structure allows the rapid diffusion of various metal ions, but their specific capacity is as low as 100 mA h g−1.28,29 Vanadium oxides can provide a high specific capacity and specific energy density, but they easily dissolve in the electrolyte and their structure collapses easily due to their own properties, thus leading to poor cycle stability.30–38 Recently, hydrated vanadium oxides (V2O5·nH2O, abbreviated as VOH) have attracted great interest owing to the following two aspects: (1) the charge shielding effect of crystal H2O can reduce the interaction between zinc ions and other ions and accelerate the intercalation/de-intercalation of zinc ions. (2) VOH can significantly improve the structural defects, and when the metal is intercalated into the interlayer of layered VOH, denoted as MxV2O5·nH2O (M = metal ion) (MVOH) (Scheme 1), the spacing between layers can be adjusted flexibly, thus increasing its electrochemical performance.39–48 MVOH with inserted water molecules has an open framework structure, and the intercalated metal ions can be used as a pillar to further expand the lattice spacing. When VOH and its derivatives are used as cathode materials for ARZIBs, the diffusion of zinc ions can be accelerated, and the quick and reversible process of zinc ion intercalation/de-intercalation can be ensured.43 For example, Linda F. Nazar's group intercalated Zn2+ into the interlayer of VOH (Zn0.25V2O5·nH2O) using a microwave hydrothermal technique for the first time.39 This fine structural enabled high specific capacities (250–300 mA h g−1) and good cycle stability (more than 80% after 1000 cycles). Subsequently, some other metal ions, such as Li+,40 Ca2+,41 Mg2+,47 Na+,49 and Al3+,44,46 intercalated into VOH, and showed good electrochemical performances. Among them, the intercalation of Ca2+ into VOH to regulate the interlayer spacing has attracted attention. For example, Chuan Xia et al. synthesized Ca0.24V2O5·0.83H2O41via a facile hydrothermal method, which exhibited an enhanced electrochemical performance. The specific capacity reached 340 mA h g−1 at 0.2 C, and it also exhibited a great rate ability of 96% after 3000 cycles at 80 C. However, although its performance exceeded that of most cathode materials, its specific capacity needs to be further increased. At present, numerous studies have shown that the introduction of carbon-based materials can enhance the electrical conductivity, and thus increase the rate performance of electrode materials. Specifically, due to its advantages of fast electron transport capability and mechanical strength, graphene not only improves the conductivity of electrodes, but also stabilizes the original structure of active materials.50–52 Pang et al. prepared and studied an H2V3O8/graphene composite electrode for ARZIBs with an impressive rate ability of 270 mA h g−1 at 20 C after 2000 cycles.51 To the best of our knowledge, the fabrication of metal-intercalated VOH integrated with reduced graphene oxide (rGO) as a cathode material for application in ARZIBs has rarely been reported. Therefore, it is desirable to fabricate composites of metal-intercalated VOH integrated with graphene as cathode materials for high rate ARZIBs.
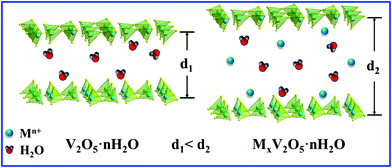 |
| Scheme 1 Structure of VOH and MVOH (M = Mn, Ca, Fe, and Zn), demonstrating the intercalation of metal ions. | |
In this work, we proposed the “double guarantee mechanism” of Ca2+-intercalated hydrated V2O5 (CaVOH) integrated with reduced graphene oxide (rGO), denoted as CaVOH/rGO, which was prepared through a simple hydrothermal method and subsequent freeze-drying treatment. The intercalation of the calcium ion can enlarge the layer spacing of VOH, and the integration of rGO can significantly enhance the conductivity and stability of the composite. This idea enabled the composite to an exhibit outstanding storage capacity of 409 mA h g−1 at 0.05 A g−1, and admirable cycling performance with a specific capacity of 299 mA h g−1 after 2000 cycles at 4 A g−1.
2. Results and discussion
2.1. Composition and structure of CaVOH/rGO
Fig. 1a presents a schematic of the synthetic process for the CaVOH/rGO composite via a facile hydrothermal method and freeze-drying treatment (detail synthesis presented in the ESI†). Firstly, the reaction between V2O5 and H2O2via the facile green method generates V-based complexes,53 and the GO is uniformly dispersed under ultrasonic treatment.54 Secondly, in the subsequent hydrothermal process, Ca2+ enters the interlayer space of VOH, expanding the VOH layer spacing like pillars. Simultaneously, CaVOH is fixed to the surface functional groups of GO by electrostatic attraction or hydroxyl bonding.44 As a large number of Ca2+ is constantly inserted into the interlayer of VOH, the lamellar structures of VOH transforms into the belt-like CaVOH, which adheres onto the GO surface. Finally, the CaVOH/rGO composite is obtained through a subsequent freeze-drying treatment.
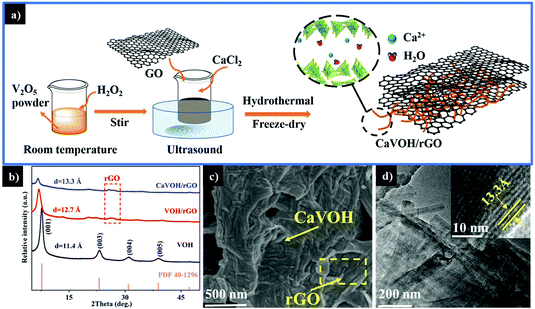 |
| Fig. 1 (a) Schematic illustration of the synthesis of CaVOH/rGO. (b) XRD patterns of VOH, VOH/rGO and CaVOH/rGO. (c) SEM and (d) TEM and HRTEM images of CaVOH/rGO. | |
Fig. 1b compares the X-ray diffraction (XRD) patterns of VOH, VOH/rGO and CaVOH/rGO. It can be observed that the peaks for VOH are consistent with the standard peaks (V2O5·1.6H2O, JCPDS#40-1296). The strongest peak of VOH is located at the 2θ value of 7.73° with the lattice spacing of 11.4 Å, which is in accordance with the preceding report.42 The strongest peak for VOH/rGO is located at 6.94° with the lattice spacing of 12.7 Å, which shows that the interlayer spacing was enlarged after the formation of the VOH and rGO composite.43 This may be attributed to the fact that more water molecules or tiny pieces of rGO entered the interlayer of VOH during the reaction with rGO. The strongest peak of CaVOH/rGO is located at 6.63°, indicating the lattice spacing of 13.3 Å. Compared to VOH/rGO, no impurity peak can be observed. Notably, the characteristic peak at around 25° belonging to rGO reveals that the material was successfully compounded with rGO. The results suggest that the layer spacing of CaVOH/rGO was further expanded upon the intercalation of Ca2+ and the crystal structure of CaVOH/rGO is more open than that of VOH, which provides an easier channel for Zn2+ to be inserted and removed.41 The morphology of the three materials was characterized by scanning electron microscopy (SEM) (at the accelerating voltage of 3000 V and working distance of 5 mm) and transition electron microscopy (TEM). As shown in Fig. S1† and Fig. 1c, unlike the large-scale nanosheets of rGO, striped nanobelts of VOH, VOH/rGO and CaVOH/rGO can be observed, and VOH/rGO and CaVOH/rGO are completely covered by rGO, which is consistent with the TEM image of CaVOH/rGO (Fig. 1d). It should be that the presence of rGO can be clearly seen in the yellow boxes in Fig. 1 and Fig. S1.† VOH and CaVOH grew uniformly on the large-scale nanosheets of rGO and their combination with rGO was very close. Because of the excellent mechanical strength of rGO, the composite can exhibit an enhanced ion transportation efficiency, which is beneficial to the kinetics of the electrochemical reaction.55 According to the HRTEM image shown in the insert in Fig. 1d, the obvious lattice fringes indicate the high crystallinity of the integrated composite, and the measured lattice distance of 13.3 Å corresponds to the (001) planes of CaVOH, which is consistent with the XRD pattern. These results demonstrate that we successfully synthesized CaVOH/rGO with an expanded layer spacing of up to 13.3 Å.
The structural characteristics of the products were further confirmed via Fourier-transform infrared (FTIR) spectroscopy, Raman spectroscopy and X-ray photoelectron spectroscopy (XPS). In the FTIR spectra (Fig. 2a), peaks appeared at 3400 cm−1 and 1612 cm−1, which are attributed to the stretching vibration of O–H and the bending vibration of H–O–H, respectively. The peaks located around 1005 cm−1 correspond to the stretching vibration of V
O. In the enlarged spectra, this peak splitting is divided into two small peaks. In the spectrum of VOH, the peaks at 1013 cm−1 and 1002 cm−1 are attributed to the stretching vibration of V5+
O and V4+
O, respectively.54 However, upon the intercalation of Ca2+ and compounding with rGO, the two peaks gradually merged into one peak (the peak of CaVOH/rGO is located at 1007 cm−1), which indicates that more V5+ was reduced to V4+ during this process, and thus the splitting of the peak became less obvious. Moreover, the peaks at 761 cm−1 and 517 cm−1 correspond to the asymmetric and symmetric stretching vibration of the V–O–V bands, respectively.56 Besides, the peaks of C–H (1380 cm−1) and C–O (1240 cm−1), which are characteristic peaks of rGO, can be observed, illustrating the successful integration of rGO.57Fig. 2b shows the Raman spectra of the three materials, as supplementary fingerprints for their FTIR spectra. The peak at 144 cm−1 belongs to the stretching vibration of (V2O2)n.58 The peaks at 284 cm−1 and 408 cm−1 for CaVOH/rGO correspond to the bending vibration of the V
O bond,59 which show a red shift compared with that of VOH (477 cm−1) and VOH/rGO (416 cm−1), in accordance with the value of the XRD minimum angle. The peak at 524 cm−1 is attributed to the stretching vibration of the V3–O bond formed by O3 with three coordinated V-ions. The peak at 689 cm−1 belongs to the stretching vibration of V2–O, indicating that there are some unordered V–O–V connections in the main lattice, while the peak at 991 cm−1 is related to the stretching vibration of V
O at the edge.60 Compared with the peaks at 1200–1800 cm−1 for the three materials, the two characteristic peaks near 1360 and 1610 cm−1 can be observed for VOH/rGO and CaVOH/rGO, indicating the successful integration of rGO. The peak at 1360 cm−1 is called the D-band, which is related to the in-plane stretching vibration of sp3 hybridized carbon atoms, while the peak at 1612 cm−1 is called the G-band, which is related to the stretching vibration of sp2 hybridized carbon atom.7 Compared with VOH/rGO (1604 cm−1), the G-peak of CaVOH/rGO (1612 cm−1) shifted to a higher frequency. This proves that the compression effect of the composite increased due to the intercalation of Ca2+, in other words, Ca2+, VOH and rGO were strongly combined to form CaVOH/rGO. Simultaneously, the XPS results further proved the composition of the composites. As shown in Fig. 2c, the peaks located at 347.1 and 350.7 eV correspond to the Ca 2p3/2 and Ca 2p1/2 levels of Ca2+ in CaVOH/rGO (the data was calibrated with the Ca 2p peak), which explains the successful insertion of Ca2+.41 In addition, the detailed peak separation of the V 2p peaks in the XPS spectra of the three materials is compared in Fig. 2d–f. The characteristic peaks at 516.5/523.9 eV and 517.1/525.0 eV in the XPS spectrum originate from V4+ and V5+, respectively.61 The increase of the strength of V4+/V5+ may be because of the reduction of V5+ to V4+ when VOH was compounded with rGO. The intercalation of Ca2+ also caused V5+ to be converted to V4+ due to the electroneutrality of the lattice. A small amount of V4+ was found in VOH, which may be due to a reduction reaction, where V2O5 and H2O2 react to form VO(O2)+.42 Meanwhile, Fig. S2a† displays the peaks corresponding to V, O, Ca and C in the XPS survey spectra of the three samples, in which CaVOH/rGO possesses characteristic element of Ca. Also, Fig. S2b–f† display the detailed peak separation of the C 1s and O 1s peaks in the XPS spectra of VOH, VOH/rGO and CaVOH/rGO, where the C 1s peak was deconvoluted into three peaks corresponding to C–C, C–O and C
O (Fig. S2d and e†), which originate from the integration of rGO of VOH/rGO and CaVOH/rGO (the data was calibrated with standard data). According to the XPS analysis (Table S1†), the ratio of Ca and V is about 0.12
:
1, while according to the inductively coupled plasma-atomic emission spectroscopy (ICP-AES) test (Table S2†), the corresponding value is about 0.11
:
1. Thus, these values are consistent. Furthermore, the weight percentage of rGO in the composite was confirmed by thermogravimetric analysis (TGA) (∼9.14 wt%, Fig. S3†) and elemental analysis (EA) (Table S3†). As shown in Table S3,† the element content of C is 5.21%. Also, considering that there was a certain amount of H and O in rGO, the two test results are consistent. The composition of CaVOH/rGO can be denoted as Ca0.22V2O5·nH2O/rGO based on the above characterizations.
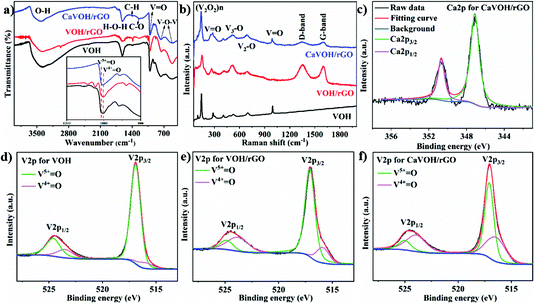 |
| Fig. 2 (a) FTIR and (b) Raman spectra of VOH, VOH/rGO and CaVOH/rGO. (c) High-resolution Ca element XPS spectra of CaVOH/rGO. (d–f) High resolution V element XPS spectra of VOH, VOH/rGO and CaVOH/rGO, respectively. | |
2.2. Electrochemical performances of CaVOH/rGO
Fig. 3a presents a schematic of the setup of the ARZIB, which was made up of CaVOH/rGO as the cathode, Zn metal as the anode and 3 M Zn(CF3SO3)2 aqueous solution as the electrolyte. Fig. 3b shows a comparison of the cyclic voltammetry (CV) curves of the three materials recorded at the scanning rate of 1 mV s−1, where two pairs of similar redox peaks can be observed. The two pairs of peaks are divided into a pair of peaks at around 0.6 V for V4+/V3+ and 1.0 V for V5+/V4+. Notably, some small reduction peaks around 0.2 V and 0.8 V were observed, demonstrating the multistep (de)intercalation of Zn2+ in CaVOH/rGO. By calculation, it is more obvious that the voltage difference in the redox pairs of CaVOH/rGO (0.16 V) is smaller than that of VOH (0.22 V) and VOH/rGO (0.20 V). Furthermore, both the oxidation and reduction potentials in CaVOH/rGO are higher than that for the other two materials, suggesting that the polarization and reaction kinetics of the cathode of CaVOH/rGO are smaller and superior in the electrochemical process.46 Galvanostatic charge–discharge tests (GCD) were performed to further understand the electrochemical performance of the CaVOH/rGO composite. Fig. 3c and Fig. S4a, b† present the corresponding charge/discharge profiles of the three materials at different current densities in the range of 0.05 to 4 A g−1, and the corresponding voltage plateaus in the GCD curves are all in good agreement with the CV curves (Fig. 3b). The comparison of these images shows that with an increase in the current density, the specific capacity decreased slightly. However, the range of changes for CaVOH/rGO is smaller than that of VOH and VOH/rGO. In addition, Fig. 3d more intuitively shows that at different current densities ranging from 0.05 to 4 A g−1, the maximum specific capacity of CaVOH/rGO decreased from 408.3 mA h g−1 to 268.7 mA h g−1, while the capacities of VOH/rGO and VOH are much lower. The electrochemical properties of CaVOH/rGO are superior to the reported vanadium-based cathode materials for ARZIBs (a comparison of the data is shown in Table S4 in the ESI†). The rate capability of the three materials was tested via GCD at different current densities (Fig. 3e). The current density gradually increased from 0.1 A g−1 to 2 A g−1 and then returned to 0.1 A g−1. At the current density of 0.1 A g−1, the initial specific capacity of the CaVOH/rGO electrode was 325.8 mA h g−1, which was much higher than that of the VOH/rGO electrode (272.1 mA h g−1) and VOH electrode (161.5 mA h g−1). At 2 A g−1, the capacity could still reach 289.1 mA h g−1, indicating that the capacity retention rate was 89%. However, the capacities of VOH and VOH/rGO were only 60.1 mA h g−1 and 235.9 mA h g−1 at 2 A g−1, respectively, which are much lower than that of CaVOH/rGO. Compared with VOH, VOH/rGO and CaVOH/rGO exhibited a higher specific capacity and better stability owing to the presence of rGO. When the current density returned to 0.1 A g−1, the capacity of CaVOH/rGO could be restored to 348.7 mA h g−1, which shows its good rate performance and strong electrochemical stability. To further verify the excellent cycle performance, the long-term cycle stability of the four materials was investigated at 4 A g−1 (Fig. 3f). The initial capacity of the CaVOH/rGO electrode at 4 A g−1 was 263.1 mA h g−1, followed by a gradual increase to 330.5 mA h g−1, and then the capacity tended to be stable (full activation after about 300 cycles), which is similar with the result from the rate capability test at the same current density. Even at a very high current density of 4 A g−1, there was no significant energy attenuation in the CaVOH/rGO cathode after 2000 cycles, exhibiting an ideal capacity of 299 mA h g−1 with 100% coulombic efficiency. Meanwhile, the capacities of VOH, VOH/rGO and CaVOH were 66.1 mA h g−1, 109.7 mA h g−1 and 130.8 mA h g−1 after 2000 cycles, respectively, indicating that the CaVOH/rGO cathode has outstanding long-term cycling stability. In addition, EDS element mapping was carried out on the material after 2000 cycles (Fig. S4c†), and it was found that the elements were uniformly distributed in the material, further demonstrating the excellent stability of CaVOH/rGO. Its performance is superior to that of reported graphene composites as cathodes for ARZIBs, such as Na1.1V3O7.9@rGO,62 RGO/VO2,63 H11Al2V6O23.2@graphene.44Fig. 4a displays the Ragone plots, where the CaVOH/rGO composite is compared with the reported electrodes applied in ARZIBs. It can be observed that the Zn//CaVOH/rGO cell delivers the maximum energy and power density of 380.8 W h kg−1 and 2044.4 W kg−1, respectively, which are superior to that of most vanadium-based cathodes reported in the literatures, for instance, Ca0.24V2O5·0.83H2O (267 W h kg−1),41 Zn3V2O7(OH)2·2H2O (214 W h kg−1),64 NaV3O8·1.5H2O (285 W h kg−1),65 Na3V2(PO4)3 (101 W h kg−1),66 and Zn0.25V2O5·nH2O (215 W h kg−1),39 demonstrating the obvious superiority of the CaVOH/rGO cathode.
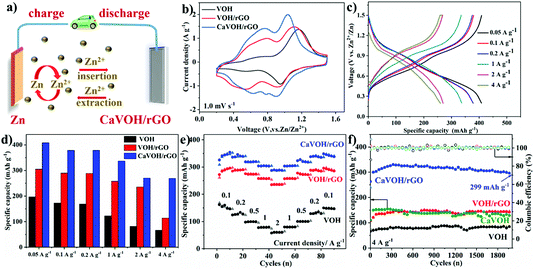 |
| Fig. 3 (a) Illustration of the charge/discharge process for the obtained aqueous zinc-ion battery. (b) CV curves of VOH, VOH/rGO and CaVOH/rGO at 1 mV s−1. (c) GCD curve and (d) bar chart showing the specific capacity of VOH, VOH/rGO and CaVOH/rGO at various sweep rates. (e) Rate capability and (f) cycling stability at 4 A g−1 of VOH, VOH/rGO, CaVOH and CaVOH/rGO. | |
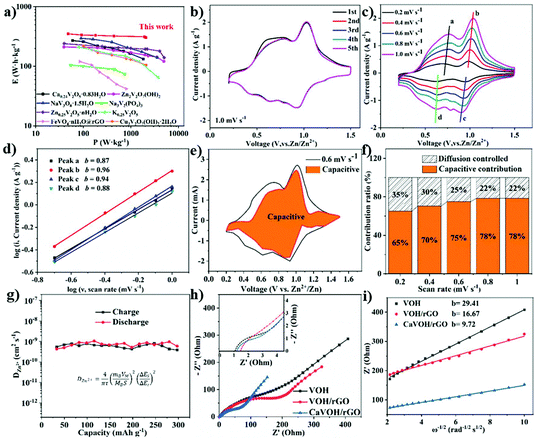 |
| Fig. 4 (a) Ragone plots of CaVOH/rGO composite compared with previously reported vanadate electrodes applied in AZIBs. (b) CV curves of CaVOH/rGO in the first five cycles at 1 mV s−1. (c and d) CV curves at various sweep rates and the relationship between peak current and sweep rate for CaVOH/rGO. (e) CV curve with the calculated capacitive fraction shown by the shaded area at 0.6 mV s−1 and (f) contribution rate of calculated capacitance at 0.2–1 mV s−1. (g) Corresponding Zn2+ (DZn) diffusion coefficients of CaVOH/rGO. (h) EIS spectra and (i) Nyquist plots after 10th discharge/charge cycle for VOH, VOH/rGO and CaVOH/rGO. | |
The redox reaction of CaVOH/rGO was analyzed via cyclic voltammetry (CV). Fig. 4b shows the initial five cycle CV curves recorded at a scanning rate of 1 mV s−1, reflecting the intercalation process of Zn2+ in CaVOH/rGO. Two redox couples are observed, namely the V5+/V4+ transition at 1.03/0.93 V, showing a pair of distinct peaks, and the V4+/V3+ transition at 0.80/0.64 V, showing a pair of broad peaks, which indicate that the intercalation/de-intercalation of Zn2+ is a multistep reaction.46 Besides the slight deviation in the first cycle, the stable position of the redox peak and its almost same shape show that the electrode is highly reversible.59 A large part of the rate performance of Zn//CaVOH/rGO battery depends on its electrochemical kinetics. Based on the excellent electrochemical behavior of the CaVOH/rGO composite, the CV curves of Zn//CaVOH/rGO battery at various sweeping rates were compared through a detailed cyclic voltammetry analysis. It can be seen in Fig. 4c that with an increase in the scanning rate, the redox peaks are more obvious and the area of the CV curve with a similar shape gradually increases. Because of the polarization effect, the cathodic peaks and anodic peaks move to higher and lower voltages, respectively. In general, the peak current (i) and scanning rates (v) have an empirical power-law relationship as follows:
| log(i) = log(a) + b log(υ) | (2) |
where
a and
b are adjustable parameters. Generally,
b is in the range of 0.5 to 1. When the coefficient
b is 0.5, it indicates that the electrochemical process is completely diffusion controlled, whereas a value of 1 indicates that the capacitance plays a dominant role in the process of charging and discharging.
38,43 The value of
b can be obtained by calculating the slopes of formula
(2). Compared with VOH (0.50, 0.71, 0.75, and 0.70) (Fig. S5a and b
†) and VOH/rGO (0.72, 0.86, 0.83, and 0.80) (Fig. S5c and d
†), the
b values for the four peaks of CaVOH/rGO are 0.87, 0.96, 0.94, and 0.88 (
Fig. 4d), which are closer to 1. With the addition of rGO and calcium ions, the redox reaction changes from ion-diffusion controlled to dominantly pseudocapacitive-like kinetics.
39,42,43 Furthermore, the pseudocapacitance contributions at different scan rates can be calculated according to
eqn (3) and (4) as follows:
Under a certain voltage, the current is considered as two parts, where k1v is the capacitive process and the k2v1/2 is the diffusion-controlled process. k1 and k2 are adjustable parameters, which change at different scanning rates. For example, it can be seen in the orange area of Fig. 4e, at the scanning rate of 0.6 mV s−1, the pseudocapacitance contribution of CaVOH/rGO is 75%. The results show that the chemical reaction process is mainly limited by the electrochemical reaction rate itself, rather than by the ion diffusion rate. The bar graph in Fig. 4f shows the percentage of calculated pseudocapacitive contribution at five different scanning rates. It can be intuitively found that the ratio of the capacitance contribution to diffusion current contribution increased with an increase in the scanning rate. When the scanning rate increased from 0.2 mV s−1 to 1.0 mV s−1, the pseudocapacitive contribution generally improved from 65% to 78%. However, under the same conditions, the pseudocapacitive contributions of VOH and CaVOH were only 37% to 53% and 51% to 67%, respectively, which are lower than that of CaVOH/rGO (Fig. S6a–d†). Thus, the cause for the superior power performance, especially the rate capability of the prepared CaVOH/rGO material was investigated.21 To further demonstrate the advantages of its electrochemical performance, the galvanostatic intermittent titration technique (GITT) was employed to measure the ion diffusion coefficient of zinc ions in the CaVOH/rGO cathode. Based on the formula in Fig. S6e† and Fig. 4g, during the charging and discharging process, the CaVOH/rGO cathode presents a high Zn2+ diffusion coefficient (DZn2+) about 10−9 cm2 s−1, which is higher than some state-of-the-art vanadium oxide cathodes for ARZIBs.12,49,67 In contrast, as shown in Fig. S6f–k,† the DZn2+ of VOH, VOH/rGO and CaVOH is about 10−10 cm2 s−1, which is less than that of CaVOH/rGO. This result clearly demonstrates that the CaVOH/rGO material has a high Zn2+ diffusion coefficient and its open framework structure can ensure the rapid transfer of Zn2+, thus leading to a good rate performance. According to the GCD results, it can be found that the capacity of CaVOH/rGO gradually increased in the first few cycles under a certain current density, and thus the reason for this phenomenon was studied by electrochemical impedance spectroscopy (EIS) in the frequency range of 100 kHz to 0.01 Hz. Fig. 4h presents the EIS results of the three materials after 10 cycles. The EIS curve consists of two parts as follows: (i) a semicircular arc radius in the high frequency region, which represents the charge transfer resistance (Rct) at the interface between the electrode and the electrolyte. Its size depends on the Faraday reaction. (ii) A linear line in the low frequency region, which illustrates the Warburg diffusion impedance and is related with the diffusion of Zn2+ in the electrode material.68 The inherent resistance (Rc) depends on the intercept at high frequency. After 10 cycles at 1.0 A g−1, the Rct values of VOH, VOH/rGO and CaVOH/rGO were 2.98, 2.08 and 1.51 Ω, and the Rc values were 1.09, 1.21 and 1.47 Ω, respectively. The slope of the Nyquist plot of the Zn//CaVOH/rGO battery at low frequencies is shown in Fig. 4i, which indicates that the diffusion of Zn2+ at the electrode/electrolyte interface of CaVOH/rGO is easier (the slope with a linear tendency is smaller, and thus the zinc-ion diffusion in the electrochemical process is faster).35 The EIS spectra and Nyquist plots of CaVOH/rGO after the 1st, 10th and 50th cycle at 1.0 A g−1 are shown in Fig. S7a and b.† The observed change is related to the activation process of the CaVOH/rGO cathode and the reduced overpotential for the intercalation/de-intercalation of Zn2+. This property endowed CaVOH/rGO with excellent cycle stability at a high current density of 4 A g−1 (Fig. 3f).
To better understand the mechanism of zinc ion storage, the relationship between the crystal structure and electrochemical reaction of CaVOH/rGO was investigated using ex situ XRD, ex situ XPS and EDS. Fig. 5a exhibits the GCD curve of the first and second cycles with the Zn//CaVOH/rGO battery at the current density of 0.1 A g−1, in which five different discharge/charge states are marked for the ex situ test, and the corresponding ex situ XRD pattern is shown in Fig. 5b. When the original electrode was first fully discharged to 0.2 V, the (001) peak shifted from the original state to a smaller 2θ angle, which indicates that the interlayer spacing increases with the insertion of zinc ions in the discharge process. Besides, it was observed that a series of new diffraction peaks appeared in the XRD curve (the black heart symbol). This phenomenon indicates that a new phase was formed after the first full discharge. The new phase possesses an analogous structure to that of zinc vanadate (Zn3(OH)2V2O7·2H2O, JCPDS#50-0570),69 illustrating that an irreversible phase transition of Zn3(OH)2V2O7·2H2O occurred in the first discharge process, which corresponds to the slight deviation the CV curves from the first cycle (Fig. 4b). When completely charged to 1.5 V, the (001) peak returned to the original peak value, which indicates that the increased interlayer spacing gradually returned to the original state with the release of zinc ions during the charging process. Notably, the peaks formed during the first discharge process still existed after the first discharge, specifically, the following fully discharged/charged electrode displayed the same XRD peaks as the electrode after the first cycle. Therefore, it can be inferred that a reversible conversion occurs between ZnxCaVOH/rGO/Zn3(OH)2V2O7·2H2O and ZnyCaVOH/rGO/Znz(OH)2V2O7·2H2O (x > y) after the primary cycle. It should be noted that the by-product Zn3(OH)2V2O7·2H2O is a layered porous crystal, which is a favorable structure to accelerate the intercalation/de-intercalation of Zn2+ in the electrochemical process.37 Meanwhile, VOH shows a similar zinc-storage mechanism to that of CaVOH/rGO, as shown in Fig. S8.† To further research the chemical state of CaVOH/rGO in the initial and discharge/charge states, an ex situ XPS test was carried out. Fig. 5c displays the detailed spectrum of Zn2+ in the fully charged and discharged state of the CaVOH/rGO cathode. It is obvious that Zn2+ is dominant in the discharge process at 1023.0 eV (2p3/2) and 1046.2 eV (2p1/2). In the original state, there is no characteristic peak for the Zn ion, and the strength of Zn 2p in the charged state is weaker than that in the discharged state. The residual Zn2+ in the charged state may be due to the formation of irreversible by-products, or the Zn2+ in the electrolyte adsorbed on the surface of the electrode. Additionally, the V 2p peaks also exhibited great changes during the discharging/charging process (Fig. 5d–f). After full discharge (Fig. 5e), most of the V5+ was reduced to V4+ or V3+. The change in the vanadium signal means that Zn2+ inserted into the VOH interlayer, resulting in bond rearrangement among Vn+.70,71 When completely charged to 1.5 V (Fig. 5f), the V 2p peaks recovered to the original state, which demonstrates that most of the available zinc ions de-intercalated from the VOH interlayer. In summary, a change in the crystal structure occurred during the cycling process (Fig. 5g). According to the ex situ XRD analysis, the electrochemical reaction is different between the first discharge process and the other discharge processes. The corresponding electrochemical mechanism of the Zn//CaVOH/rGO rechargeable battery can be written as follows.
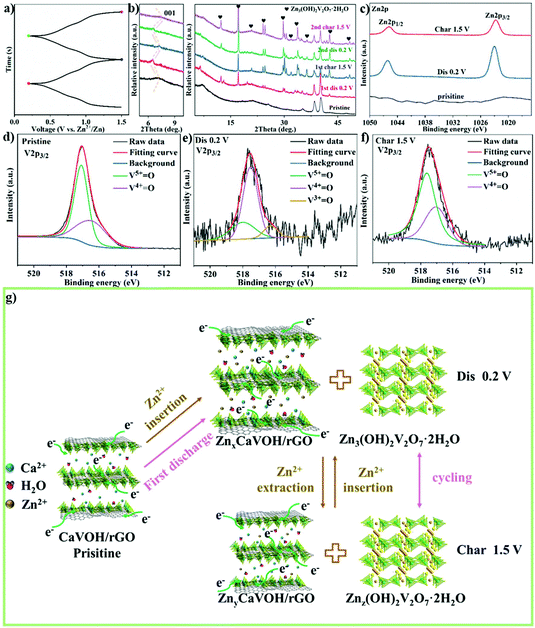 |
| Fig. 5 (a and b) Ex situ XRD patterns of CaVOH/rGO in the fully discharged and charged states in the first and second cycles. (c) XPS survey spectra of Zn 2p peaks of CaVOH/rGO in the pristine, fully discharged and charged states. (d–f) XPS survey spectra of vanadium peaks of CaVOH/rGO in the pristine, fully discharged and charged states. (g) Schematic illustration of the zinc storage mechanism in the CaVOH/rGO electrode. | |
The reactions on the zinc anode:
First discharge process in the cathode:
The other discharge processes in the cathode:
The EDS element mapping further verified the change in the element content of CaVOH/rGO in the electrochemical reaction (Fig. S9†). In the fully discharged and charged states, the signal of Zn2+ after the CaVOH/rGO cathode was discharged was obviously stronger than that after it was charged. It was also observed that the other elements were evenly distributed during the whole process of the electrochemical reaction. This again proves that reversible intercalation/de-intercalation occurs during the electrochemical process.
3. Conclusion
In summary, we successfully synthesized a CaVOH/rGO composite through a simple hydrothermal reaction, which is a novel and viable cathode material for ARZIBs. The “double guarantee mechanism” of Ca2+-intercalation and rGO-integration in the composite resulted in an admirable electrochemical performance with the energy density of 381 W h kg−1 at 48 W kg−1 and prominent capacity of 409 mA h g−1 at 0.05 A g−1. These brilliant properties are mainly attributed to the following reasons: (1) Ca2+ expands the interlayer spacing of VOH like pillars and accelerates the intercalation/de-intercalation of zinc ions during the electrochemical process. (2) The highly conductive rGO nanosheets provide more charge transfer paths and its ultrahigh mechanical strength makes the original structure of the composite more stable. Therefore, the results demonstrated that it is feasible to use CaVOH/rGO as a cathode material with superb security and stability. In this work, a new synthetic method was designed, which greatly increased the storage capacity of VOH.
Conflicts of interest
There are no conflicts to declare.
Acknowledgements
This work was partially supported by the National Natural Science Foundation of China (Grant No. 21771030), Natural Science Foundation of Liaoning Province (2020-MS-113), Fundamental Research Funds for the Central Universities (DUT18RC(6)008).
References
- M. Li, J. Lu, Z. Chen and K. Amine, 30 Years of Lithium-Ion Batteries, Adv. Mater., 2018, 30, 1800561 CrossRef.
- R. Schmuch, R. Wagner, G. Hörpel, T. Placke and M. Winter, Performance and cost of materials for lithium-based rechargeable automotive batteries, Nat. Energy, 2018, 3, 267–278 CrossRef CAS.
- M. Winter, B. Barnett and K. Xu, Before Li Ion Batteries, Chem. Rev., 2018, 118, 11433–11456 CrossRef CAS.
- X. Chen, T. Hou, K. A. Persson and Q. Zhang, Combining theory and experiment in lithium–sulfur batteries: Current progress and future perspectives, Mater. Today, 2019, 22, 142–158 CrossRef CAS.
- Y. Zhang, H. Jiang, Q. Wang and C. Meng, In-situ hydrothermal growth of Zn4Si2O7(OH)2·H2O anchored on 3D N, S-enriched carbon derived from plant biomass for flexible solid-state asymmetrical supercapacitors, Chem. Eng. J., 2018, 352, 519–529 CrossRef CAS.
- Y. Zhang, C. Wang, H. Jiang, Q. Wang, J. Zheng and C. Meng, Cobalt-Nickel Silicate Hydroxide on Amorphous Carbon Derived from Bamboo Leaves for Hybrid Supercapacitors, Chem. Eng. J., 2019, 375, 121938 CrossRef CAS.
- Y. Cheng, Y. Zhang, H. Jiang, X. Dong, C. Meng and Z. Kou, Coupled cobalt silicate nanobelt-on-nanobelt hierarchy structure with reduced graphene oxide for enhanced supercapacitive performance, J. Power Sources, 2020, 448, 227407 CrossRef CAS.
- A. Ponrouch, J. Bitenc, R. Dominko, N. Lindahl, P. Johansson and M. R. Palacin, Multivalent rechargeable batteries, Energy Storage Mater., 2019, 20, 253–262 CrossRef.
- D. Chao, W. Zhou, F. Xie, C. Ye, H. Li, M. Jaroniec and S.-Z. Qiao, Roadmap for advanced aqueous batteries: From design of materials to applications, Sci. Adv., 2020, 6, eaba4098 CrossRef CAS.
- Y. Liang, H. Dong, D. Aurbach and Y. Yao, Current status and future directions of multivalent metal-ion batteries, Nat. Energy, 2020, 5, 646–656 CrossRef CAS.
- Z. Pan, J. Yang, J. Yang, Q. Zhang, H. Zhang, X. Li, Z. Kou, Y. Zhang, H. Chen, C. Yan and J. Wang, Stitching of Zn3(OH)2V2O7·2H2O 2D Nanosheets by 1D Carbon Nanotubes Boosts Ultrahigh Rate for Wearable Quasi-Solid-State Zinc-Ion Batteries, ACS Nano, 2020, 14, 842–853 CrossRef CAS.
- H. Jiang, Y. Zhang, L. Xu, Z. Gao, J. Zheng, Q. Wang, C. Meng and J. Wang, Fabrication of (NH4)2V3O8 nanoparticles encapsulated in amorphous carbon for high capacity electrodes in aqueous zinc ion batteries, Chem. Eng. J., 2020, 382, 122844 CrossRef CAS.
- Z. Pan, J. Yang, Y. Zhang, X. Gao and J. Wang, Quasi-solid-state fiber-shaped aqueous energy storage devices: recent advances and prospects, J. Mater. Chem. A, 2020, 8, 6406–6433 RSC.
- X. Xu, F. Xiong, J. Meng, X. Wang, C. Niu, Q. An and L. Mai, Vanadium-Based Nanomaterials: A Promising Family for Emerging Metal-Ion Batteries, Adv. Funct. Mater., 2020, 30, 1904398 CrossRef CAS.
- P. Yu, Y. Zeng, H. Zhang, M. Yu, Y. Tong and X. Lu, Flexible Zn-Ion Batteries: Recent Progresses and Challenges, Small, 2019, 15, 1804760 CrossRef.
- G. Fang, J. Zhou, A. Pan and S. Liang, Recent Advances in Aqueous Zinc-Ion Batteries, ACS Energy Lett., 2018, 3, 2480–2501 CrossRef CAS.
- F. Wang, O. Borodin, T. Gao, X. Fan, W. Sun, F. Han, A. Faraone, J. A. Dura, K. Xu and C. Wang, Highly reversible zinc metal anode for aqueous batteries, Nat. Mater., 2018, 17, 543–549 CrossRef CAS.
- J. Ming, J. Guo, C. Xia, W. Wang and H. N. Alshareef, Zinc-ion batteries: Materials, mechanisms, and applications, Mater. Sci. Eng., R, 2019, 135, 58–84 CrossRef.
- F. Wan and Z. Niu, Design Strategies for Vanadium-based Aqueous Zinc-Ion Batteries, Angew. Chem., Int. Ed., 2019, 58, 16358–16367 CrossRef CAS.
- Y. Zhang, H. Jiang, L. Xu, Z. Gao and C. Meng, Ammonium Vanadium Oxide [(NH4)2V4O9] Sheets for High Capacity Electrodes in Aqueous Zinc Ion Batteries, ACS Appl. Energy Mater., 2019, 2, 7861–7869 CrossRef CAS.
- H. Jiang, Y. Zhang, Z. Pan, L. Xu, J. Zheng, Z. Gao, T. Hu and C. Meng, Facile hydrothermal synthesis and electrochemical properties of (NH4)2V10O25·8H2O nanobelts for high-performance aqueous zinc ion batteries, Electrochim. Acta, 2020, 332, 135506 CrossRef CAS.
- B. Tang, L. Shan, S. Liang and J. Zhou, Issues and opportunities facing aqueous zinc-ion batteries, Energy Environ. Sci., 2019, 12, 3288–3304 RSC.
- H. Jia, Z. Wang, B. Tawiah, Y. Wang, C.-Y. Chan, B. Fei and F. Pan, Recent advances in zinc anodes for high-performance aqueous Zn-ion batteries, Nano Energy, 2020, 70, 104523 CrossRef CAS.
- Y. Zeng, X. Zhang, Y. Meng, M. Yu, J. Yi, Y. Wu, X. Lu and Y. Tong, Achieving Ultrahigh Energy Density and Long Durability in a Flexible Rechargeable Quasi-Solid-State Zn–MnO2 Battery, Adv. Mater., 2017, 29, 1700274 CrossRef.
- D. Chao, W. Zhou, C. Ye, Q. Zhang, Y. Chen, L. Gu, K. Davey and S.-Z. Qiao, An Electrolytic Zn–MnO2 Battery for High-Voltage and Scalable Energy Storage, Angew. Chem., Int. Ed., 2019, 58, 7823–7828 CrossRef CAS.
- V. Mathew, B. Sambandam, S. Kim, S. Kim, S. Park, S. Lee, M. H. Alfaruqi, V. Soundharrajan, S. Islam, D. Y. Putro, J.-Y. Hwang, Y. Sun and J. Kim, Manganese and Vanadium Oxide Cathodes for Aqueous Rechargeable Zinc-Ion Batteries: A Focused View on Performance, Mechanism, and Developments, ACS Energy Lett., 2020, 5, 2376–2400 CAS.
- L. Chen, Z. Yang, F. Cui, J. Meng, Y. Jiang, J. Long and X. Zeng, Ultrathin MnO2 nanoflakes grown on N-doped hollow carbon spheres for high-performance aqueous zinc ion batteries, Mater. Chem. Front., 2020, 4, 213–221 RSC.
- Z. Liu, G. Pulletikurthi and F. Endres, A Prussian Blue/Zinc Secondary Battery with a Bio-Ionic Liquid–Water Mixture as Electrolyte, ACS Appl. Mater. Interfaces, 2016, 8, 12158–12164 CrossRef CAS.
- G. Zampardi and F. La Mantia, Prussian blue analogues as aqueous Zn-ion batteries electrodes: Current challenges and future perspectives, Curr. Opin. Electrochem., 2020, 21, 84–92 CrossRef CAS.
- S. Liu, L. Kang, J. M. Kim, Y. T. Chun, J. Zhang and S. C. Jun, Recent Advances in Vanadium-Based Aqueous Rechargeable Zinc-Ion Batteries, Adv. Energy Mater., 2020, 10, 2000477 CrossRef CAS.
- G. Yang, Q. Li, K. Ma, C. Hong and C. Wang, The degradation mechanism of vanadium oxide-based aqueous zinc-ion batteries, J. Mater. Chem. A, 2020, 8, 8084–8095 RSC.
- Y. Zhang, A. Chen and J. Sun, Promise and challenge of vanadium-based cathodes for aqueous zinc-ion batteries, J. Energy Chem., 2021, 54, 655–667 CrossRef.
- B. He, Z. Zhou, P. Man, Q. Zhang, C. Li, L. Xie, X. Wang, Q. Li and Y. Yao, V2O5 nanosheets supported on 3D N-doped carbon nanowall arrays as an advanced cathode for high energy and high power fiber-shaped zinc-ion batteries, J. Mater. Chem. A, 2019, 7, 12979–12986 RSC.
- P. Hu, M. Yan, T. Zhu, X. Wang, X. Wei, J. Li, L. Zhou, Z. Li, L. Chen and L. Mai, Zn/V2O5 Aqueous Hybrid-Ion Battery with High Voltage Platform and Long Cycle Life, ACS Appl. Mater. Interfaces, 2017, 9, 42717–42722 CrossRef CAS.
- F. Liu, Z. Chen, G. Fang, Z. Wang, Y. Cai, B. Tang, J. Zhou and S. Liang, V2O5 Nanospheres with Mixed Vanadium Valences as High Electrochemically Active Aqueous Zinc-Ion Battery Cathode, Nano-Micro Lett., 2019, 11, 25 CrossRef CAS.
- L. Xu, Y. Zhang, H. Jiang, J. Zheng, X. Dong, T. Hu and C. Meng, Facile hydrothermal synthesis and electrochemical properties of (NH4)2V6O16 nanobelts for aqueous rechargeable zinc ion batteries, Colloids Surf., A, 2020, 593, 124621 CrossRef.
- H. Jiang, Y. Zhang, Y. Liu, J. Yang, L. Xu, P. Wang, Z. Gao, J. Zheng, C. Meng and Z. Pan, In situ grown 2D hydrated ammonium vanadate nanosheets on carbon cloth as a free-standing cathode for high-performance rechargeable Zn-ion batteries, J. Mater. Chem. A, 2020, 8, 15130–15139 RSC.
- H. Jiang, Y. Zhang, Z. Pan, L. Xu, J. Zheng, Z. Gao, T. Hu, C. Meng and J. Wang, NH4V3O8·0.5H2O nanobelts with intercalated water molecules as a high performance zinc ion battery cathode, Mater. Chem. Front., 2020, 4, 1434–1443 RSC.
- D. Kundu, B. D. Adams, V. Duffort, S. H. Vajargah and L. F. Nazar, A high-capacity and long-life aqueous rechargeable zinc battery using a metal oxide intercalation cathode, Nat. Energy, 2016, 1, 16119 CrossRef CAS.
- Y. Yang, Y. Tang, G. Fang, L. Shan, J. Guo, W. Zhang, C. Wang, L. Wang, J. Zhou and S. Liang, Li+ intercalated V2O5·nH2O with enlarged layer spacing and fast ion diffusion as an aqueous zinc-ion battery cathode, Energy Environ. Sci., 2018, 11, 3157–3162 RSC.
- C. Xia, J. Guo, P. Li, X. Zhang and H. N. Alshareef, Highly Stable Aqueous Zinc-Ion Storage Using a Layered Calcium Vanadium Oxide Bronze Cathode, Angew. Chem., Int. Ed., 2018, 57, 3943–3948 CrossRef CAS.
- C. Liu, Z. Neale, J. Zheng, X. Jia, J. Huang, M. Yan, M. Tian, M. Wang, J. Yang and G. Cao, Expanded hydrated vanadate for high-performance aqueous zinc-ion batteries, Energy Environ. Sci., 2019, 12, 2273–2285 CAS.
- M. Yan, P. He, Y. Chen, S. Wang, Q. Wei, K. Zhao, X. Xu, Q. An, Y. Shuang, Y. Shao, K. T. Mueller, L. Mai, J. Liu and J. Yang, Water-Lubricated Intercalation in V2O5·nH2O for High-Capacity and High-Rate Aqueous Rechargeable Zinc Batteries, Adv. Mater., 2018, 30, 1703725 CrossRef.
- W. Zhang, S. Liang, G. Fang, Y. Yang and J. Zhou, Ultra-High Mass-Loading Cathode for Aqueous Zinc-Ion Battery Based on Graphene-Wrapped Aluminum Vanadate Nanobelts, Nano-Micro Lett., 2019, 11, 69 CrossRef.
- Y. Liu, Z. Pan, D. Tian, T. Hu, H. Jiang, J. Yang, J. Sun, J. Zheng, C. Meng and Y. Zhang, Employing “one for two” strategy to design polyaniline-intercalated hydrated vanadium oxide with expanded interlayer spacing for high-performance aqueous zinc-ion batteries, Chem. Eng. J., 2020, 399, 125842 CrossRef CAS.
- J. Zheng, C. Liu, M. Tian, X. Jia, E. P. Jahrman, G. T. Seidler, S. Zhang, Y. Liu, Y. Zhang, C. Meng and G. Cao, Fast and reversible zinc ion intercalation in Al-ion modified hydrated vanadate, Nano Energy, 2020, 70, 104519 CrossRef CAS.
- F. Ming, H. Liang, Y. Lei, S. Kandambeth, M. Eddaoudi and H. N. Alshareef, Layered MgxV2O5·nH2O as Cathode Material for High-Performance Aqueous Zinc Ion Batteries, ACS Energy Lett., 2018, 3, 2602–2609 CrossRef CAS.
- L. Xu, Y. Zhang, J. Zheng, H. Jiang, T. Hu and C. Meng, Ammonium ion intercalated hydrated vanadium pentoxide for advanced aqueous rechargeable Zn-ion batteries, Mater. Today Energy, 2020, 18, 100509 CrossRef.
- P. Hu, T. Zhu, X. Wang, X. Wei, M. Yan, J. Li, W. Luo, W. Yang, W. Zhang, L. Zhou, Z. Zhou and L. Mai, Highly Durable Na2V6O16·1.63H2O Nanowire Cathode for Aqueous Zinc-Ion Battery, Nano Lett., 2018, 18, 1758–1763 CrossRef CAS.
- M. Chen, Y. Zhang, Y. Liu, Q. Wang, J. Zheng and C. Meng, Three-Dimensional Network of Vanadium Oxyhydroxide Nanowires Hybridize with Carbonaceous Materials with Enhanced Electrochemical Performance for Supercapacitor, ACS Appl. Energy Mater., 2018, 1, 5527–5538 CAS.
- Q. Pang, C. Sun, Y. Yu, K. Zhao, Z. Zhang, P. M. Voyles, G. Chen, Y. Wei and X. Wang, H2V3O8 Nanowire/Graphene Electrodes for Aqueous Rechargeable Zinc Ion Batteries with High Rate Capability and Large Capacity, Adv. Energy Mater., 2018, 8, 1800144 CrossRef.
- X. Wang, Y. Li, S. Wang, F. Zhou, P. Das, C. Sun, S. Zheng and Z.-S. Wu, 2D Amorphous V2O5/Graphene Heterostructures for High-Safety Aqueous Zn-Ion Batteries with Unprecedented Capacity and Ultrahigh Rate Capability, Adv. Energy Mater., 2020, 10, 2000081 CrossRef CAS.
- Y. Li, J. Yao, E. Uchaker, J. Yang, Y. Huang, M. Zhang and G. Cao, Leaf-Like V2O5 Nanosheets Fabricated by a Facile Green Approach as High Energy Cathode Material for Lithium-Ion Batteries, Adv. Energy Mater., 2013, 3, 1171–1175 CrossRef CAS.
- T. Hu, Y. Liu, Y. Zhang, M. Chen, J. Zheng, J. Tang and C. Meng, 3D hierarchical porous V3O7·H2O nanobelts/CNT/reduced graphene oxide integrated composite with synergistic effect for supercapacitors with high capacitance and long cycling life, J. Colloid Interface Sci., 2018, 531, 382–393 CrossRef CAS.
- Y. Zhang, M. Chen, T. Hu and C. Meng, 3D Interlaced Networks of VO(OH)2 Nanoflakes Wrapped with Graphene Oxide Nanosheets as Electrodes for Energy Storage Devices, ACS Appl. Nano Mater., 2019, 2, 2934–2945 CrossRef CAS.
- Y. Zhang, X. Jing, Y. Cheng, T. Hu and C. Meng, Controlled synthesis of 3D porous VO2(B) hierarchical spheres with different interiors for energy storage, Inorg. Chem. Front., 2018, 5, 2798–2810 RSC.
- X. Dong, Y. Zhang, Q. Wang, X. Zhang, M. Gao and M. Changgong, Synthesis of urchin-like Ni3Si2O5(OH)4 hierarchical hollow spheres/GO composite with enhanced electrochemical properties for high-performance hybrid supercapacitors, Dalton Trans., 2019, 48, 11749–11762 RSC.
- Y. Zhang, X. Jing, Q. Wang, J. Zheng, H. Jiang and C. Meng, Three-dimensional porous V2O5 hierarchical spheres as a battery-type electrode for a hybrid supercapacitor with excellent charge storage performance, Dalton Trans., 2017, 46, 15048–15058 RSC.
- P. He, G. Zhang, X. Liao, M. Yan, X. Xu, Q. An, J. Liu and L. Mai, Sodium Ion Stabilized Vanadium Oxide Nanowire Cathode for High-Performance Zinc-Ion Batteries, Adv. Energy Mater., 2018, 8, 1702463 Search PubMed.
- J. Zheng, Y. Zhang, T. Hu, T. Lv and C. Meng, New Strategy for the Morphology-Controlled Synthesis of V2O5 Microcrystals with Enhanced Capacitance as Battery-type Supercapacitor Electrodes, Cryst. Growth Des., 2018, 18, 5365–5376 CrossRef CAS.
- J. Mendialdua, R. Casanova and Y. Barbaux, XPS studies of V2O5, V6O13, VO2 and V2O3, J. Electron Spectrosc. Relat. Phenom., 1995, 71, 249–261 CrossRef CAS.
- Y. Cai, F. Liu, Z. Luo, G. Fang, J. Zhou, A. Pan and S. Liang, Pilotaxitic Na1.1V3O7.9 nanoribbons/graphene as high-performance sodium ion battery and aqueous zinc ion battery cathode, Energy Storage Mater., 2018, 13, 168–174 CrossRef.
- X. Dai, F. Wan, L. Zhang, H. Cao and Z. Niu, Freestanding graphene/VO2 composite films for highly stable aqueous Zn-ion batteries with superior rate performance, Energy Storage Mater., 2019, 17, 143–150 CrossRef.
- C. Xia, J. Guo, Y. Lei, H. Liang, C. Zhao and H. Alshareef, Rechargeable Aqueous Zinc–Ion Battery Based on Porous Framework Zinc Pyrovanadate Intercalation Cathode, Adv. Mater., 2020, 32, 1907798 CrossRef CAS.
- F. Wan, L. Zhang, X. Dai, X. Wang, Z. Niu and J. Chen, Aqueous rechargeable zinc/sodium vanadate batteries with enhanced performance from simultaneous insertion of dual carriers, Nat. Commun., 2018, 9, 1656 CrossRef.
- G. Li, Z. Yang, Y. Jiang, C. Jin, W. Huang, X. Ding and Y. Huang, Towards polyvalent ion batteries: A zinc-ion battery based on NASICON structured Na3V2(PO4)3, Nano Energy, 2016, 25, 211–217 CrossRef CAS.
- F. Cui, J. Zhao, D. Zhang, Y. Fang, F. Hu and K. Zhu, VO2(B) nanobelts and reduced graphene oxides composites as cathode materials for low-cost rechargeable aqueous zinc ion batteries, Chem. Eng. J., 2020, 390, 124118 CrossRef CAS.
- J. Zheng, Y. Zhang, Q. Wang, H. Jiang, Y. Liu, T. Lv and C. Meng, Hydrothermal encapsulation of VO2(A) nanorods in amorphous carbon by carbonization of glucose for energy storage devices, Dalton Trans., 2018, 47, 452–464 RSC.
- B. Tang, G. Fang, J. Zhou, L. Wang, Y. Lei, C. Wang, T. Lin, Y. Tang and S. Liang, Potassium vanadates with stable structure and fast ion diffusion channel as cathode for rechargeable aqueous zinc-ion batteries, Nano Energy, 2018, 51, 579–587 CrossRef CAS.
- J.-Q. Huang, X. Guo, X. Lin, Y. Zhu and B. Zhang, Hybrid Aqueous/Organic Electrolytes Enable the High-Performance Zn-Ion Batteries, Research, 2019, 2019, 2635310 CAS.
- Z. Peng, Q. Wei, S. Tan, P. He, W. Luo, Q. An and L. Mai, Novel layered iron vanadate cathode for high-capacity
aqueous rechargeable zinc batteries, Chem. Commun., 2018, 54, 4041–4044 CAS.
Footnote |
† Electronic supplementary information (ESI) available: Experimental section and various characterizations. See DOI: 10.1039/d0qi00954g |
|
This journal is © the Partner Organisations 2021 |