DOI:
10.1039/D0QI00902D
(Research Article)
Inorg. Chem. Front., 2021,
8, 12-25
Hofmeister effect in the Keggin-type polyoxotungstate series†
Received
27th July 2020
, Accepted 12th September 2020
First published on 18th September 2020
Abstract
The chaotropic character of Keggin-type polyoxotungstate anions was evaluated with respect to their ability to bind to γ-cyclodextrin (γ-CD) by varying the global charge density of the nanometer-sized polyanion. The strengths of the host–guest association were analyzed within the series of isostructural [XW12O40]n− anions where the ionic charge varies from 6- to 3- depending on the heteroatom, respectively, X = H22+, B3+, Si4+ or P5+. Titration experiments using complementary techniques (ITC, DOSY NMR, and electrochemistry) revealed that the affinity between γ-CD and polyoxometalates (POMs) is directly correlated to the charge density of the Keggin anion as reflected in the values of the binding constants K1:1. These constants increase dramatically following the order: [H2W12O40]6− < [BW12O40]5− < [SiW12O40]4− < [PW12O40]3−. Additionally, cloud point experiments on a non-ionic surfactant resulted in the same series of POMs, emphasizing the general affinity of these inorganic Keggin-ions to non-ionic organic soft matter due to a general solvent effect arising from the weakening of the hydration sphere with decreasing ionic charge. Furthermore, single crystal X-ray diffraction analysis showed distinct organizations of the POMs with γ-CD in the solid-state, where the moderate chaotrope [BW12O40]5− interacts with the external wall of the γ-CD, while on the other side of the series, [PW12O40]3− penetrates deeply into the cavity of the γ-CD through its secondary rim offering optimal contact area. Finally, our investigations revealed the unique behavior of [PW12O40]3−, which displayed not only the highest affinity of the POMs to γ-CD (K1:1 > 105 M−1), but also the ability to interact with both CD faces resulting in a wide variety of supramolecular aggregates.
Introduction
Polyoxometalates (POMs) are a remarkable class of anionic polynuclear metal oxide clusters of transition metal ions like WVI or MoVI.1 POMs are able to reversibly and massively exchange electrons without structural change, making them promising electro-active subcomponents of hybrid systems relevant for applications in the energy field.2 Recent studies highlighted that POMs are an appealing molecular ‘electron-reservoir’ to construct lithium-, sodium-ion and redox flow batteries,3–7 supercapacitors,8 fuel cells9,10 or catalytic systems11–14 relevant for sustainable processes. The development of straightforward preparation routes to hybrid materials including POM units through supramolecular contacts has led to unique molecular assemblies with novel properties and reactivity.15 In context, the exploration of ionic and other non-covalent interactions (hydrogen bonding, van der Waals forces, etc.) between POMs and other types of molecular systems has attracted considerable interest, revealing the remarkable supramolecular behavior of POMs to self-assemble across multiple length scales ranging from discrete systems to gigantic spherical single-layered nanostructures known as “blackberries”.12,16–20
One of the most striking supramolecular properties of POMs arises from their ability to form in solution resilient non-bonding contacts with neutral surfaces such as organic macrocycles, micelles, proteins or polymers.21–30 Actually, their solution behavior appears “counter-intuitive” because POMs are anionic inorganic discrete species considered generally as hydrophilic. Others and the authors established that association phenomena between POMs and organic moieties are driven by a solvent mediated effect arising from a water structure recovery upon release of the POM's and the moiety's hydration water.22,26,31 POMs as well as other polynuclear species (dodecaborates or electron-rich octahedral clusters) have been identified as water structure breakers, also named chaotropic entities after the Hofmeister classification.22,32–34 Based on the current understanding of the Hofmeister series, the chaotropicity of an ion should increase when its charge density decreases, but regarding POMs more insight is needed into the influence of the ionic charge, especially the effect of redox change on their chaotropic character.22,31 From a thermodynamic point of view, processes involving chaotropes are usually considered to be enthalpically driven accompanied by an entropic penalty, i.e. enthalpy–entropy compensation.26,35
Cyclodextrins (CDs) are natural cyclic oligosaccharides with a hydrophobic internal cavity and a hydrophilic external surface, and thus exhibit interesting properties as host molecules for a wide variety of inorganic species including polyoxometalates.36 CDs are readily available as α-, β- and γ-forms comprising six, seven, or eight glucose units, respectively. The host–guest assembly of the toroidal CDs and spherical POM clusters has attracted much attention and is a fast-growing topic. In 2015, Stoddart and coworkers published the first examples of host–guest complexes between γ- and β-CDs and phosphomolybdate [PMo12O40]3−.30 Since then, several hybrid (organic/inorganic) adducts or materials have also been reported with various archetypal polyoxotungstates including the Lindqvist-type [W6O19]2−,37,38 the Keggin-type ions [PW12O40]3− and [SiW12O40]4−,38–43 and the Dawson-type [P2W18O62]6−.25,26 Some of these hybrid CD/POM materials are promising for application in catalysis39,41 and energy storage,44 where surface effects and hybrid structures would play a crucial role. Therefore, detailed characterization of the interactions between CDs and POMs and a better understanding of the phenomena occurring at their interfaces are essential for further innovative development of redox-responsive systems and related applications.
Herein, we studied the formation of host–guest inclusion complexes of γ-CD and Keggin-type POM anions along the [XW12O40]n− series with anionic charges from 3- to 6- (Fig. 1) while keeping constant the shape and size of the host and of the guest. Using a set of complementary techniques (NMR, ITC and electrochemistry), we show in this article that affinity between γ-CD and Keggin-type anions increases dramatically with decreasing ionic charge. Simultaneously, we evaluate the chaotropic behavior using a simple experimental procedure based on the propensity of the POM species to adsorb on the non-ionic ethylene glycol-based surfactant C8E4. Thus, we establish unambiguously in this report the direct correlation between the chaotropic nature and the formation of highly stable supramolecular complexes. The present study might open new opportunities for the development of smart supramolecular devices with responsive behavior based on redox changing of the POM unit.
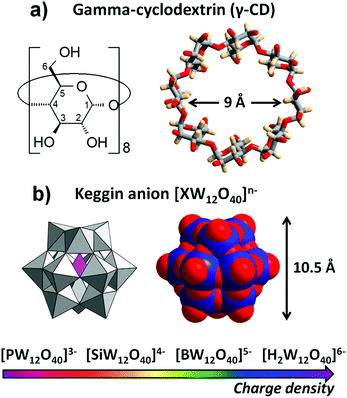 |
| Fig. 1 Structural representations of the molecular entities used as building blocks. (a) γ-cyclodextrin C48H80O40 (γ-CD) resulting from the condensation of eight glycopyranose units. The toroidal macrocycle delimits a hydrophobic cavity of 9 Å in diameter. (b) The Keggin-type POM, [XW12O40]n−, is composed of twelve octahedral WO6 polyhedra that form an anionic tetrahedral cage {W12O40}8− with a central cavity occupied by the heteroatom X (P5+, Si4+, B3+ or H22+). The choice of X allows the control of the charge density of the nano-sized inorganic POM. | |
Results and discussion
Synthesis
Attempts for the isolation of the supramolecular adducts have been carried out systematically using different ratios of sodium salt of POMs Nam[XW12O40]·nH2O with X = P5+, Si4+, B3+, H22+ and varying procedures of crystallization such as slow evaporation of aqueous solution or ethanol vapor diffusion into aqueous solution containing cesium chloride. In all cases, crystalline products were obtained but only [BW12O40]5− and [PW12O40]3− led to single crystals suitable for XRD analysis. Their composition was established combining different complementary techniques (elemental analysis, EDS, XRD, and TGA). The compounds CsK2H2{[BW12O40]·2(γ-CD)}·29H2O (BW12·2CD) and Na3{[PW12O40]@(γ-CD)}·10H2O (PW12@CD) present different molecular organization as further discussed in the next section.
Review of Keggin/γ-CD interactions in the solid-state
The crystal structure of PW12@CD was found to be isostructural to the recently published compounds NaAH6[(PW12O40)3(γ-CD)3]·nH2O (A = CoII or CuII).45 Zhan and coworkers introduced Co(NO3)2 or CuSO4 to aqueous solutions containing γ-CD and Na3[PW12O40] to obtain crystalline products.45 In our case, only Na+ and H+ were present evidencing that the POM-CD packing in PW12@CD is highly flexible to accommodate various types of counter-cations without structural change. PW12@CD's structure consists of a supramolecular 1
:
1 adduct involving a [PW12O40]3− unit partially incorporated into the cavity of γ-CD through its secondary rim (Fig. 2a). This configuration is quite unique among the few known polyoxometalate/γ-CD composites that mostly feature interactions with the primary face.26,30 Of particular interest, comparison with the isostructural Keggin phosphomolybdate [PMo12O40]3− reveals a different complexation mode with γ-CD involving the opposite face, namely, the primary rim (Fig. 2b).30 Furthermore, the stoichiometry of the [PMo12O40]3−
:
CD adduct is 1
:
2, while a 1
:
1 stoichiometry was found with [PW12O40]3− although higher stoichiometry numbers should be expected as observed with Dawson-type POMs with a stoichiometry of 1
:
1 up to 1
:
3.26 Indeed, while PW12@CD crystallized in a 1
:
1 solution mixture, other crystalline products were obtained with higher γ-CD contents (2 γ-CDs per POM unit), but we were unable, despite numerous repeated attempts, to solve the solid-state structures of these crystals featuring a tetragonal unit cell (a = b = 23.94 and c = 18.54 Å). The 1
:
1 adduct observed in PW12@CD exhibits several non-bonding contacts involving the terminal Ot and bridging Ob atoms of the POM and H3 and H5 protons (see Fig. 1a for H labeling) of the inner cavity of the γ-CD (see Fig. S1, in the ESI†). The short interatomic distances H3⋯Ot
W (from 2.69 to 3.31 Å), H3⋯Ob–W (from 2.41 to 2.88 Å), H5⋯Ot
W (from 2.29 to 3.38 Å), and H5⋯Ob–W (from 3.13 to 3.88 Å) reflect a strong and deep host–guest inclusion. As PW12@CD crystallizes in a cubic crystallographic system, the 1
:
1 host–guest adduct is surrounded by four host–guest units giving additional non-bonding contacts between the exposed face of the Keggin ion and the external surface of each of the neighboring γ-CDs (see Fig. S2, in the ESI†).
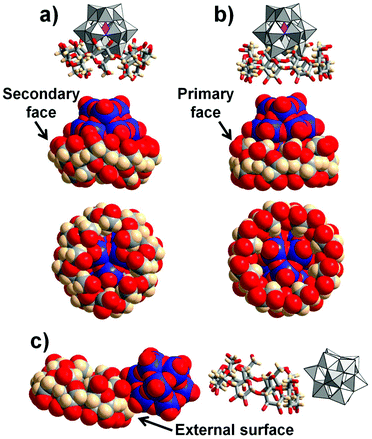 |
| Fig. 2 Illustration of the different supramolecular assemblies observed in the solid-state involving γ-CD and the Keggin anions (a) [PW12O40]3− in PW12@CD, (b) [PMo12O40]3− from reference,30 and (c) [BW12O40]5− in BW12·2CD. | |
In the solid-state, the organization of the [BW12O40]5− and γ-CD is significantly different since the crystalline structure of BW12·2CD reveals that the two components co-crystallize without forming an inclusion complex. Indeed, the [BW12O40]5− is positioned near the γ-CD in close contact with its external wall (Fig. 2c). One [BW12O40]5− unit is surrounded by eight γ-CDs and each γ-CD is in contact with four [BW12O40]5− through the three outward protons H1, H2, and H4 as well as the H6 of the methoxy arm (see Fig. S3, ESI†). This leads to a global stoichiometry of γ-CD/POM of 2:1. Two neighboring γ-CDs are face-to-face connected through a hydrogen bonding network from their secondary rims. The [BW12O40]5− is therefore positioned near the primary face with H6⋯Ot
W and H6⋯Ob–W distances in the 2.66–2.91 Å and 2.73–3.91 Å range, respectively. This arrangement with empty γ-CDs is unique in POM/γ-CD composites although it could occur with the smaller α-CD.44 Indeed, the [PW12O40]3− Keggin anion led to an extended framework with α-CD forming double layers of interconnected [PW12O40]3− and CD motifs through bridging alkali cations.44 This highlights the importance of the size-matching effect upon the molecular recognition process,46 however this could not explain the difference of binding interactions observed in the Keggin/γ-CD arrangement.
Finally, it is surprising to see how γ-CD interacts so differently with isostructural nano-sized objects, namely [PW12O40]3−, [PMo12O40]3−, and [BW12O40]5−. By keeping the charge constant, the basicity of Keggin molybdates is known to be stronger compared to their tungstate analogues as the charge density is higher on the oxygen atoms at the surface of the molybdates.47,48 Furthermore, the ionic charge must also play a crucial role in the supramolecular association.21 Both these aspects are expected to affect significantly solvent structuration around the POM, and should have important consequences in the formation and stability of the resulting POM-based supramolecular arrangement. Systematic studies in solution are thus needed to shed light on such effects.
Stability in solution
To investigate the complexation of the Keggin-type anions by γ-CD, titration experiments were conducted in solution by means of a set of complementary techniques including NMR spectroscopy (1H 1D and DOSY), electrochemistry, and isothermal titration calorimetry (ITC).
Isothermal titration calorimetry
ITC experiments were carried out to determine the thermodynamic fingerprint of the interaction of γ-CD respectively with the four Keggin-type heteropolytungstates. ITC thermograms and isotherms are shown in the ESI (see Fig. S4–6, ESI†), and a summary of binding constants, enthalpy ΔrH* and entropy ΔrS* changes at 298 K are given in Table 1. Besides the 1
:
1 complexation, a second 1
:
2 step process is systematically considered, according to eqn (1) and (2) shown below. | POMn− + CD → [POM@CD]n− | (1) |
| [POM@CD]n− + CD → [POM@2CD]n− | (2) |
Table 1 Binding constants (M−1) of γ-CD with the Keggin-type POMs and associated thermodynamic parameters (kJ mol−1) at T = 298 K measured by ITC. Calculated uncertainties are inferior to 10%
POM |
POM : CD |
K
|
ΔrH* |
TΔrS* |
ΔrG* |
[H2W12O40]6− |
— |
— |
— |
— |
— |
[BW12O40]5− |
1 : 1 |
1032 |
−53.5 |
−36.3 |
−17.2 |
[SiW12O40]4− |
1 : 1 |
17 209 |
−56.4 |
−32.3 |
−24.2 |
1 : 2 |
459 |
−55.0 |
−39.8 |
−15.2 |
[PW12O40]3− |
1 : 1 |
2919 |
−35.1 |
−15.3 |
−19.8 |
Nonetheless, only ITC data for silicotungstate were found to be consistent with the 2
:
1 binding model involving a sequential process. No significant heat exchange was observed in the case of [H2W12O40]6− indicating negligible interactions with γ-CD. For all other complexes, supramolecular binding revealed to be enthalpically driven. The enthalpic gain is accompanied by an entropic penalty according to the usual enthalpy–entropy compensation observed within CD-based host–guest series.49 These thermochemical fingerprints together with low values of both enthalpic and entropic changes suggest that Keggin anions behave as chaotropic species (water structure breakers).31 In such a case, the magnitude of the chaotropic character and the related binding constants are expected to increase with decreasing global charge. However, the highest enthalpic and entropic changes (and binding constants) were observed for [SiW12O40]4− and the lowest values were found for [PW12O40]3−. This result represents therefore an anomaly within the expected series, as confirmed by the electrochemical and NMR studies discussed below. The discrepancy here may have appeared for two reasons: (i) the partial hydrolysis of the [PW12O40]3− anion under the ITC conditions (non-buffered and diluted solution) or (ii) the simultaneous formation of numerous complexes with various stoichiometries, e.g. 1
:
1, 1
:
2, 2
:
1, etc., (overparametrization).
Electrochemical studies
Interactions of the Keggin-type ions with γ-CD have been studied further using cyclic voltammetry. As shown below, the presence of γ-CD alters significantly the redox properties of the POMs. Besides, the POM's redox state is expected to have a high impact on the binding constants. As shown in Fig. 3, CVs with γ-CD appear to be strongly dependent on the nature of the Keggin anion.
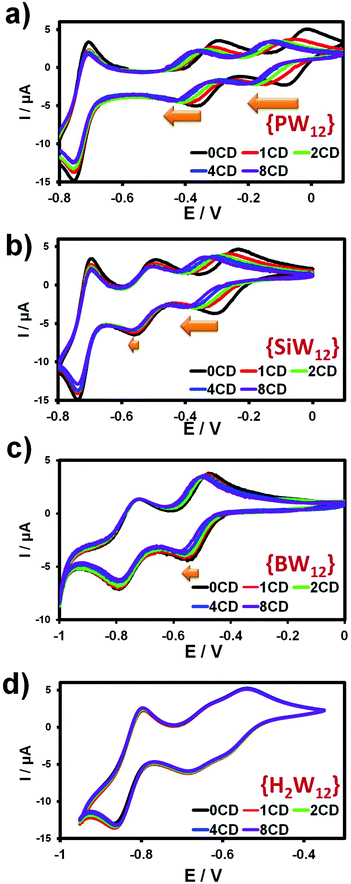 |
| Fig. 3 Cyclic voltammetry of the [XW12O40]n− anions with (a) X = P5+, (b) Si4+, (c) B3+ or (d) H22+ (0.5 mmol L−1, glassy carbon working electrode, scan rate 50 mV s−1) in the presence of increasing amounts of γ-CD (from 0 to 8 equivalents). The experiments involving [PW12O40]3−, [SiW12O40]4− and [BW12O40]5− have been performed in 25 mmol L−1 HClO4 aqueous solution, while acetate buffer solution (0.5 M CH3COONa; 0.5 M CH3COOH) has been used for the electrochemical measurements of [H2W12O40]6−. | |
For instance, as the amount of γ-CD increases up to 8 equivalents, the half-wave potential related to the two first monoelectronic transfers of the [PW12O40]3− ion decreases continuously (see Fig. 3). Actually, the electrochemical behavior of the [PW12O40]3− ion gives the most representative effect reflected by a rough dependency of about −60 mV/pCD where pCD = −Log[CD] (see Fig. S9, ESI†). This variation indicates that γ-CD is involved in the predominant redox process written in eqn (3), with two consecutive monoelectronic transfers, n = 3 or 4 for the first and the second redox processes, respectively. Furthermore, the complexation behavior of γ-CD should be rather consistent with x = 2 or 1 corresponding to a 2
:
1 and 1
:
1 supramolecular adduct, respectively.
| [POM(CD)x]n− + 1e− → [POM(CD)x−1](n+1)− + CD | (3) |
Along the Keggin series, the variation of the redox properties becomes less pronounced as the ionic charge of the Keggin ion increases. Thus, in the case of [SiW12O40]4−, the first mono-electronic redox wave appears strongly affected, whereas the second one varies weakly. For the boron derivative, only the first redox wave is moderately shifted and finally, the redox features of [H2W12O40]6− remain nearly unchanged in the presence of γ-CD (see Fig. 3). With the exception of [PW12O40]3−, the redox behavior reflects the trend highlighted by the ITC analysis.
Quantitative analysis of these electrochemical data can yield the equilibrium constants (K1:1 and K1:2) regarding the binding of the Keggin anions at various redox states by the γ-CD. Considering the three available oxidation states of POMn− (ox), POM(n+1)− (red1), POM(n+2)− (red2), associated with the three related complexed forms POM@xCD with x = 0, 1 or 2, the 3X3 matrix diagram can be drawn (see Scheme 1).
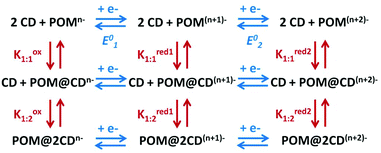 |
| Scheme 1 Equilibria involved in redox-dependent supramolecular association of γ-cyclodextrin (CD) and the Keggin type anions (POM). | |
Including the six redox components involved in each redox process, the Nernst equations are written as followed:
|  | (4) |
|  | (5) |
where
E01 and
E02 are the standard potentials of each redox process and [CD]
eq is the concentration in free γ-CD at the equilibrium. The values
K1:1 and
K1:2 correspond to the binding constants associated with
eqn (1) and (2), respectively, wherein the POM can be in its three redox states, oxidized (ox), one-electron reduced (red1) or two-electron reduced (red2) (see
Scheme 1). Depending on the Keggin-type POM,
eqn (4) or (5) were adapted and then used to extract the binding constants through the fitting of experimental shift of the apparent potential upon free γ-CD concentration (see the ESI, Fig. S7–9
†). The case of the metatungstate ion [H
2W
12O
40]
6− is trivial as neither ITC nor electrochemistry evidences any interaction with γ-CD. For the [BW
12O
40]
5− ion, ITC experiments showed the formation of a 1
![[thin space (1/6-em)]](https://www.rsc.org/images/entities/char_2009.gif)
:
![[thin space (1/6-em)]](https://www.rsc.org/images/entities/char_2009.gif)
1 adduct. Furthermore, the second wave is nearly independent of the γ-CD concentration meaning that the one-electron and the two-electron reduced forms do not interact with γ-CD. Under such conditions, the expression of the apparent potential
Epeak,1 is adapted from
eqn (4) by using only the binding constant
Kox1:1. Decreasing further the ionic charge produces a richer and more complex behavior. The first wave of the [SiW
12O
40]
4− anion undergoes a shift of about −60 mV per
pCD unit (see the ESI, Fig. S8
†) meaning the Nernst Equation can be derived from
eqn (3). The second wave exhibits only moderate variation, consistent with a weak complexation of the one-electron reduced form (red
1) and no complexation for the two-electron reduced form (red
2). Then
eqn (5) is derived by using only the binding constant

and matches the experimental data for

. Considering the first monoelectronic exchange, both the 1
![[thin space (1/6-em)]](https://www.rsc.org/images/entities/char_2009.gif)
:
![[thin space (1/6-em)]](https://www.rsc.org/images/entities/char_2009.gif)
1 and the 1
![[thin space (1/6-em)]](https://www.rsc.org/images/entities/char_2009.gif)
:
![[thin space (1/6-em)]](https://www.rsc.org/images/entities/char_2009.gif)
2 adduct must be considered for the oxidized form as shown by ITC. Then
eqn (4) should include
Kox1:1,
Kox1:2 and

= 130 M
−1. Suitable fitting with experimental data was obtained with numerical values 9500 and 150 M
−1 for
Kox1:1 and
Kox1:2, respectively. Finally, the electrochemical behavior of the [PW
12O
40]
3− ion consists of two CD-dependent monoelectronic waves which move both by about −60 mV per
pCD unit in accordance with
eqn (3). The second wave is satisfactory modeled by using

= 6000 M
−1 and

= 30 M
−1 while calculation of the first redox potentials requires the inclusion of
Kox1:1 and
Kox1:2 in addition to the binding constants related to the reduced form [PW
12O
40]
4−, namely red
1. From
eqn (4), good agreement was found between calculated and experimental data for
Kox1:1 = 90
![[thin space (1/6-em)]](https://www.rsc.org/images/entities/char_2009.gif)
000 M
−1 and
Kox1:2 = 1500 M
−1. The values of the overall binding constants are reported in
Table 2 and the comparison between experimental data and the simulated evolution of the observed apparent potential is reported in the ESI (see Fig. S7–9
†). The binding constants determined by electrochemistry for [BW
12O
40]
5− and [SiW
12O
40]
4− ions are significantly lower compared to those found by ITC (see
Table 1). This difference is likely due to the different medium used in the ITC experiments (pure water) and in the electrochemical measurements (aqueous solution of 0.025 mol L
−1 HClO
4) and may arise from the presence of hydronium ions H
3O
+ or perchlorate ion ClO
4− involved in competition processes with the POM-CD aggregation. Importantly, analysis of the electrochemical data allows us to determine the
K1:1 binding constants of the [PW
12O
40]
3− ion. With a value of 9 × 10
4 M
−1, the affinity of [PW
12O
40]
3− for γ-CD is about one order of magnitude higher than the affinity of [SiW
12O
40]
4−. Such a high value corresponds to one of the largest affinity constants reported so far with γ-CD.
32,50,51 Moreover, the second binding constant
K1:2 is also quite large, around one order of magnitude higher than the
K1:2 value observed for [SiW
12O
40]
4−.
Table 2 Binding constants K1:1 and K1:2 involving γ-CD with the Keggin-type anions at different oxidation states as determined from electrochemistry. The accuracy is estimated to be within 10% of the corresponding value
X |
POM : CD |
[XW12O40]3− |
[XW12O40]4− |
[XW12O40]5− |
P |
1 : 1 |
90 000 |
6 000 |
0 |
1 : 2 |
1 500 |
30 |
0 |
Si |
1 : 1 |
— |
9 500 |
130 |
1 : 2 |
— |
150 |
0 |
B |
1 : 1 |
— |
— |
600 |
1 : 2 |
— |
— |
0 |
This electrochemical study enabled to restore a logical sequence within the Keggin-type series, corroborating the fact that the POM/γ-CD association is mainly related to the global charge of POMs. For instance, plotting LogK1:1versus the ionic charge of the Keggin-type anion results in a quite perfect linear relationship (see the ESI, Fig. S10†). Furthermore, analysis of the electrochemical data provides access to the binding constants of the lower oxidation states of the Keggin anions (see Table 2). The binding constants of the reduced POM(n+1)− exhibit lower values compared to those determined for the oxidized parent (POMn−), evidencing again the preponderant effect of the charge on the association process with the macrocyclic host. For instance, the binding constants of the one-electron reduced [PW12O40]4− anion (K1:1 = 6000 M−1) fall in a similar range order to that found for the oxidized silicotungstate [SiW12O40]4− (K1:1 = 9500 M−1) (see Table 2). A similar observation is made for the one electron-reduced silicotungstate [SiW12O40]5− and the oxidized borotungstate [BW12O40]5−, which both exhibit comparable affinity toward γ-cyclodextrin. Besides, such a trend can be compared to results reported in a previous study which demonstrated that decreasing the ionic charge of a rhenium-selenide cluster [Re6Se8(CN)6]4− through a one-electron oxidative process provokes a dramatic increase of the K1:1 binding constant from 1.5 × 103 to 2.2 × 105 M−1.34 Nevertheless, alteration of the redox properties through supramolecular interactions remains quite intriguing in the case of the Keggin-type polyoxometalates. γ-CD being a non-ionic component, its interaction with POM does not change the ionic charge as observed generally with protons or lithium ions. In context, proton-coupled electron transfer (PCET) involving POM species is a well-known pH dependent process featured by an increase of the standard potential as pH decreases. Such an effect can even be extended to the POM-lithium aggregation process under reducing conditions in non-aqueous solvents.52 In the presence of γ-CD, the origin of the redox potential variation upon γ-CD interaction should arise mostly from a change in the solvation shell of the POM corresponding to a shed of surrounding water molecules upon binding to γ-CD resulting in a more hydrophobic environment around the POMs. The influence of the medium, i.e. solvent and electrolyte support, on the redox properties of the POM species is well documented experimentally as well as through theoretical calculations and related studies reinforce our interpretation of the γ-CD effect on the redox properties of Keggin-type POMs.53,54 For instance, the first one-electron wave of the [SiW12O40]4− ion undergoes a 600–700 mV potential shift toward the negative potential when the medium is changed from aqueous to organic such as acetonitrile or dimethyl sulfoxide. This effect has been attributed to the capacity of the solvent to stabilize the reduced derivatives and has been quantified by the acceptor number (AN) of the solvent showing that the reduction process becomes difficult as solvent AN decreases. Water exhibits a high AN value (58.4) while less hydrophilic or less polar media feature lower AN parameters.55 Furthermore, the intensity of the peak current decreases significantly in the presence of γ-CD. This behavior accounts for the complexation process which lowers the diffusion coefficient of the parent oxidized POM and then consecutively affects the peak current intensity, according to the Randles–Sevcik equation.56 This effect on the current peak intensity appears quite pronounced in the CVs of the [PW12O40]3− ion, which exhibits the strongest affinity with the γ-CD and diminishes as the ionic charge of the POM increases. The diffusion coefficient change upon γ-CD complexation is also nicely supported by the 1H DOSY NMR study presented below.
To sum up, electrochemical investigations demonstrate that the binding constants K1:1 and K1:2 increase by at least one order of magnitude as the global ionic charge decreases along the Keggin-type series [XW12O40]n−. From this observation, the Keggin/γ-CD association appears as an appealing component for redox switches and could be integrated into the design of stimuli-responsive supramolecular systems controlled by the redox state of the POMs.
NMR spectroscopy
The formation and stability of Keggin POM/γ-CD complexes in aqueous solution were further probed by 1H NMR spectroscopy in D2O. In previous separate studies, the interaction of γ-CD with both [PW12O40]3− and [SiW12O40]4− has been characterized by 1H NMR.39,45 Here, we conducted a comparative titration study of 2 mM aqueous γ-CD solution with the four Keggin-type anions. Fig. 4 shows the resulting 1H NMR spectra in the range 3.7–4.5 ppm corresponding to the resonance domain of the most exposed protons to guest species, namely H3 and H5 are located inside the cavity and H6 on the top of the secondary rim (see Fig. 1 for the proton numbering scheme and ESI Fig. S11–14† for the complete 1H NMR spectra). These protons are very sensitive to host–guest contact and are usually used as indicators to probe the inclusion phenomenon.
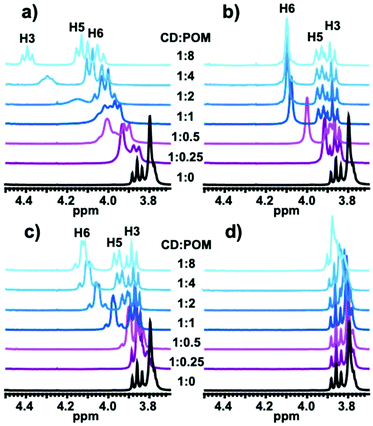 |
| Fig. 4
1H NMR spectra in the chemical shift range for H3, H5, and H6 resulting from the titration of 2 mmol L−1 aqueous γ-CD solution with [XW12O40]n− POMs. (a) [PW12O40]3−; (b) [SiW12O40]4−; (c) [BW12O40]5− and (d) [H2W12O40]6−. | |
Depending on the Keggin anion, the spectra underwent more or less strong alteration evidencing POM-specific recognition. Table 3 summarizes the extent of shift variations observed for H3, H5, and H6 after adding eight equivalents of each Keggin-type POM. As expected, the 1H NMR spectrum of the macrocyclic host remains nearly unchanged when [H2W12O40]6− was added to the γ-CD solution, and the maximum shifts observed were ca. 0.07 ppm for both H5 and H6 indicating very weak interaction. Notably, much larger effects were observed in the cases of [BW12O40]5− and [SiW12O40]4−, where shifts up to 0.33 and 0.18 ppm were observed for H6 and H5, respectively. These changes in the spectra are similar suggesting comparable behavior in solution for [BW12O40]5− and [SiW12O40]4−. Nevertheless, close inspection revealed some differences. First, the H6 (and also H5) signal evolves much more significantly with increasing [SiW12O40]4− amount compared to [BW12O40]5− although the final chemical shift at the highest ratio is the same. As the observed chemical shift corresponds to a weighted average of free and complexed γ-CD species, this means the binding constant of POM-CD complexation should be higher with [SiW12O40]4− than with [BW12O40]5−. The second observation is the splitting of the initial singlet of the H6 protons into two unresolved doublets in [BW12O40]5− containing solution. This may be due to constrained dynamic rotation of the methoxy arms which narrows the signal of the two methylenic diastereotopic H6 protons. Such steric hindrance would result from the interaction of the POM specifically with the primary rim of the γ-CD since the H3 protons present in the secondary rim did not show any significant perturbation. The situation is totally different with the [PW12O40]3− anion where, besides the shift of H6, we can also appreciate strong effects on both H5 and H3 resonances with up to 0.53 and 0.36 ppm downfield shifts, respectively, indicating involvement of the secondary face of the γ-CD in the strong interaction with [PW12O40]3−. Furthermore, we note that the line broadening of the H3 signal for POM:γ-CD ratio below two is the signature of strong POM/γ-CD interactions involved in a 1
:
2 stoichiometry supramolecular adduct.57
Table 3 Differences in chemical shifts (ppm) of protons H3, H5, and H6 of γ-CD before and after the addition of eight equivalents of POM
POM |
ΔδH3 |
ΔδH5 |
ΔδH6 |
[H2W12O40]6− |
0.02 |
0.06 |
0.07 |
[BW12O40]5− |
0.03 |
0.18 |
0.33 |
[SiW12O40]4− |
0.03 |
0.16 |
0.30 |
[PW12O40]3− |
0.53 |
0.36 |
0.28 |
By analyzing the chemical shift variation arising from the fast chemical exchange regime, quantitative treatment allows for a determination of the binding constants for 1
:
1 POM:γ-CD complexes (for further details, see Fig. S15 and 16, ESI†). The values obtained for K1:1 are 23 and 1100 M−1 for [H2W12O40]6−
:
CD and [BW12O40]5−
:
CD, respectively, that compare well with the ITC and electrochemistry results (see Tables 1 and 2). In summary, NMR results are fully consistent with electrochemistry showing that the interaction strength with γ-CD increases in the following order: [H2W12O40]6− < [BW12O40]5− < [SiW12O40]4− < [PW12O40]3−.
Further quantitative investigations were also carried out using DOSY NMR. This technique is a valuable tool to study dynamic equilibria, and similarly to the chemical shift, the observed diffusion coefficient D in the fast exchange limit can be described as a weighted average of a given species evolving between different states.58 Thus, the observed NMR of γ-CD in the presence of POM corresponds to an average situation weighted on residency times between free (solvated) and bound states. The observed diffusion coefficients D as a function of the POM
:
γ-CD molar ratio produce different profiles depending on the nature of the Keggin-type anions (see Fig. 5). From the initial value for solvated γ-CD measured at 254 μm2 s−1, the self-diffusion D drops more or less abruptly upon addition of POM and reaches a plateau at a large excess of POM at 226 μm2 s−1 in the case of [SiW12O40]4− and [BW12O40]5−, while for the [H2W12O40]6− ion, the decrease of the self-diffusion coefficient draws a smooth slope featured by larger self-diffusion values as an indication of very weak POM-γ-CD interactions. For the [PW12O40]3− ions, the D profile is characteristic of strong interactions. The decrease of D is sharper, reaching even the lower limit value measured at 219 μm2 s−1. We note also a minimum value for the curves of both [SiW12O40]4− and [PW12O40]3− at ca. POM
:
γ-CD = 0.5 suggesting the presence of bulkier intermediate species at these compositions. Both curve profiles have been modeled satisfactorily using either a one-step complexation model for [H2W12O40]6− and [BW12O40]5− or a two-step complexation model for [SiW12O40]4− and [PW12O40]3−. Simulations of these curves considering binding constants consistent with those determined previously from ITC, electrochemistry and also 1D NMR studies allowed to estimate diffusion coefficients of γ-CD within 1
:
1 and 1
:
2 adducts. Fair agreements between calculated and experimental data were found using D values of 226 and 163 μm2 s−1 for POM@CD and POM@2CD adducts, respectively. Noteworthy, the same D values were used for the 1
:
1 or 1
:
2 adduct independently of the nature of the Keggin-type anion (see the ESI for details of modeling, Fig. S17–20†), considering similar volume/size for the POM-CD adduct along the Keggin-type series. The observed lower limit value for D with [PW12O40]3− (219 vs. 226 μm2 s−1, see the ESI for modeling, Fig. S20†) highlights the remarkable solution behavior of the phosphotungstate ion as reported by Antonio et al.59 In this composition domain, it may result from some further aggregation which could be consistent with a γ-CD unit sandwiched by two [PW12O40]3− ions interacting with both primary and secondary faces of the γ-CD. This hypothesis is also quite consistent with the 1H NMR titration which reveals interactions involving simultaneously both rims of the γ-CD.
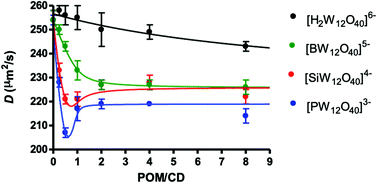 |
| Fig. 5 Diffusion coefficients of γ-CD as a function of the POM:γ-CD molar ratio, measured from 1H DOSY NMR of a solution of 2 mM of γ-CD with various POM contents. Lines correspond to calculated curves with one-step 1 : 1 POM : CD complexation for [H2W12O40]6− and [BW12O40]5−, and with two-step 1 : 2 POM : CD complexation for [SiW12O40]4− and [PW12O40]3−. | |
Furthermore, 1H NMR can also characterize the proton-containing [H2W12O40]6− anion exhibiting a resonance at ca. 6.0 ppm. Very small changes in the diffusion coefficient D (245–260 μm2 s−1) were observed over the titration experiment, reflecting very weak interaction with γ-CD as previously identified.
Driving force of the aggregation processes
This comparative study evidences clearly that Keggin-type anions bind to γ-CD to different extents despite their iso-structure. Although γ-CD is non-ionic, the charge of the POM plays a dramatic role in the molecular recognition process, both in its thermodynamic stability and in its supramolecular structure. All experimental measurements (ITC, electrochemistry and NMR) have shown that the interaction with [H2W12O40]6− is very weak (K1:1 ≈ 2.3 × 101 M−1), while it is very strong with [PW12O40]3− (K1:1 ≈ 1.6 × 105 M−1). It was proposed that the binding affinity is related to the charge density of the polyanion, which has an effect on the hydration strength, i.e. hydration free energy of the ions. The monotonous trend observed along the oxidized derivatives of the Keggin-type series could be understood by thermodynamic features of the hydration shell around the POM, which is directly related to its chaotropic nature. The weak charge density of the Keggin-type POM generates a loosely bound solvation shell of disordered water molecules with high structural entropy. The energy gain associated with the release of this hydration water into the bulk upon binding to non-ionic moieties (at micellar surfaces, water surfactant monolayer or macrocycles) was proposed as the main driving force of the superchaotropic effect of POMs and more generally of low charge density nano-ions.22 The evolution of the cloud point of a non-ionic surfactant, tetra-ethylene glycol mono-octyl ether (C8E4), upon addition of POMs was proposed as a tool to evaluate their chaotropic behavior.22 The higher the observed cloud point increase, the stronger the interaction with the non-ionic surfactant and the stronger the chaotropic nature of the tested ion. This procedure was carried out for the Keggin-type anions investigated here. Corresponding to the respective increase in the cloud point by each POM, Fig. 6 illustrates an increase in the chaotropic nature of the polyanions from [H2W12O40]6− to [PW12O40]3−, i.e. from high to low charge density. The superchaotropic P-, Si- and B-Keggin POMs produce much stronger increases in the cloud point compared to the classical chaotrope SCN−. The resulting ordering of POMs according to their chaotropic behavior is in full agreement with the series obtained by electrochemistry and NMR measurements.
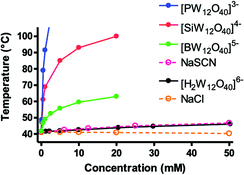 |
| Fig. 6 Cloud point evolution of 60 mM C8E4 as a function of salt concentration for the four isostructural Keggin-ions [PW12O40]3−, [SiW12O40]4−, [BW12O40]5− and [H2W12O40]6−. The classical salts NaCl (neutral salt) and NaSCN (classical chaotropic salt) are included as references. | |
SAXS measurements provided further insight into the superchaotropic character of the Keggin POMs (see Fig. S21 and S22, ESI†). [H2W12O40]6−in the presence of C8E4 shows identical scattering as in bare water, while with [BW12O40]5− a strong oscillation appears, which is even more pronounced with [SiW12O40]4−. This oscillation is characteristic of core–shell structuration and typical of POM-decorated micelles. The increase of the oscillation with the superchaotropic character of the Keggin-ion is a direct indicator of the extent of adsorption onto the C8E4-micelles resulting in the same ordering as in other experiments: [SiW12O40]4− > [BW12O40]5− > [H2W12O40]6. It is worth noting that in the case of [PW12O40]3− precipitation occurs in the presence of C8E4 micelles, preventing SAXS measurement. Nevertheless, precipitate formation hints at a particularly strong interaction of Na3[PW12O40] with the surfactant.
Overall, we showed herein that the Keggin-type POMs interact with the γ-CD through various recognition modes depending on the POM's chaotropic power. The different interaction types previously identified can be classified along the Keggin ion series related to the magnitude of the binding constants. As tentatively depicted in Fig. 7, lowering of the ionic charge gives rise to multimodal supramolecular processes consistent with our experimental observations. On the left side of the scale, the [H2W12O40]6− ion shows nearly no ability to interact with γ-CD and the [BW12O40]5− species reveals only weak interactions that involve preferably the outer surface of the CD as observed in the crystal structure of BW12·2CD (see Fig. 2c). Lowering further the ionic charge down to [SiW12O40]4− ion leads to the formation of 1
:
1 and 1
:
2 inclusion complexes involving mostly the primary face featuring higher binding constants. Finally, on the right side, the lowest ionic charge anion [PW12O40]3− gives rise to the highest binding constants reflected in the large diversity of supramolecular binding modes. As shown previously by 1H NMR, both primary and secondary faces contribute to the POM's complexation and the complementary DOSY NMR study suggested even the presence of an unusual supramolecular assembly corresponding to a γ-CD sandwiched by two [PW12O40]3− anions. Despite the lack of any structural model for such an arrangement, NMR showed clearly that in excess of POM, the primary face represented by H5 and H6 resonances and the secondary face detected by H3 resonances are both simultaneously involved in low self-diffusion coefficient assembly compatible with a {POM⋯CD⋯POM} arrangement. The binding on the primary and secondary faces of the CD, which results in a large contact surface with the POM, is favored over the binding of POMs on the exterior side of the CD
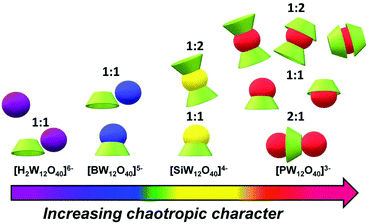 |
| Fig. 7 Illustration of the most representative binding modes involved in the supramolecular assemblies of γ-CD (green torus) and the Keggin anions (spheres). | |
Conclusions
A comparative study allowed for an establishment of the relationship between the ionic charge of Keggin-type polyoxotungstate anions and their affinity towards a non-ionic substrate, γ-cyclodextrin. As the charge is lowered systematically keeping the molecular size and shape, the ability to form supramolecular complexes increases continuously as was also observed for the interaction of the POMs with non-ionic surfactant micelles. The effects governing such supramolecular assemblies are suggested to be directly related to the solvation properties of the Keggin ions and to their propensity to shed hydration water upon the formation of POM-substrate contact. While the most charged POMs, [H2W12O40]6− and [BW12O40]5− showed weak to moderate affinity towards γ-CD, interacting mainly with its exterior wall, the lowest charge density species formed sandwich-type host–guest complexes involving either the primary face or the secondary face, featuring larger shared interfaces. Thus, in the Keggin-type series, the [PW12O40]3− ion previously identified as a superchaotrope60 exhibits a remarkable capacity to bind γ-CD through its two faces, thus presenting unique aggregation behavior. The host–guest adaptability allows maximizing contact interfaces through weak dispersion forces, highly amplified through a solvent effect arising from the pronounced superchaotropic nature of the [PW12O40]3− ion. This remarkable solution behavior of the [PW12O40]3− ion is not restricted to the systems involving cyclodextrins and the present study gives a better understanding of other self-assembly processes involving non-ionic substrates in water.21,59,61,62 Furthermore, POM/CD association has dramatic consequences on the POM's redox properties, i.e. a decrease in the standard redox potential. This effect can be understood as a partial removal of the hydration shell of the redox active ions arising from the presence of bulky γ-CD in the close vicinity of the POM. It is worth noting that opposite effects corresponding to a redox potential increase were commonly observed for interaction with cations such as protonation or ion-pairing with lithium cation.
Finally, the propensity of the Keggin-type anions to bind to γ-CD can be dramatically tuned by reversible electron transfer as demonstrated herein. This opens opportunities for the design of smart molecular materials like sensors, artificial muscles, and actuators, responsive to redox or possibly light stimuli.
Experimental section
Chemicals
All reagents were purchased from commercial sources and used without further purification. K4.3Na0.7[BW12O40]·17H2O,63 H5[BW12O40]·29H2O,64 K4[SiW12O40]·6H2O,65 Na0.6H3.4[SiW12O40]·16H2O,66 K3[PW12O40]·5H2O, Na1.4H1.6[PW12O40]·12H2O, and H3[PW12O40]·14H2O65,67 were prepared according to literature procedures and checked by routine analyses prior use. The synthesis of Rb6[H2W12O40]·11H2O is described below. Solutions were prepared in Milli-Q water.
Materials characterization
Fourier transform infrared (FT-IR).
Spectra were recorded on a 6700 FT-IR Nicolet spectrophotometer, using the diamond ATR technique. The spectra were recorded on non-diluted compounds and ATR correction was applied.
Energy-dispersive X-ray spectroscopy (EDS).
EDS measurements were performed using a SEM-FEG (scanning electron microscope enhanced by a field emission gun) equipment (JSM 7001-F, Jeol). The measures were acquired with a SDD XMax 50 mm2 detector and the Aztec (Oxford) system working at 15 kV and 10 mm working distance. The quantification is realized with the standard library provided by the constructor using Lα lines.
Thermal gravimetric analysis (TGA).
To determine water and organic contents, a Mettler Toledo TGA/DSC 1, STARe System apparatus was used under oxygen or nitrogen flow (50 mL min−1) at a heating rate of 5 °C min−1 up to 700 °C.
Single-crystal X-ray diffraction (XRD).
Intensity data collections were carried out at T = 200(2) K with a Bruker D8 VENTURE diffractometer equipped with a PHOTON 100 CMOS bidimensional detector using high brilliance IμS microfocus X-ray Mo Kα monochromatized radiation (λ = 0.71073 Å). Crystals were glued in paratone oil to prevent any loss of crystallization water. Data reduction was accomplished using SAINT V7.53a. The substantial redundancy in data allowed a semi-empirical absorption correction (SADABS V2.10) to be applied, on the basis of multiple measurements of equivalent reflections. Using Olex2,68 the structure was solved with the ShelXT69 structure solution program using Intrinsic Phasing and refined with the ShelXL70 refinement package using Least Squares minimization. Heavier atoms (W) for each structure were initially located by direct methods. The remaining nonhydrogen atoms were located from Fourier differences and were refined with anisotropic thermal parameters. Positions of the hydrogen atoms belonging to the γ-CDs were calculated and refined isotropically using the gliding mode. In the compound PW12@CD, numerous free water molecules (H2O) located inside the voids are disordered. Thereby the contribution of solvent-electron density was removed using the SQUEEZE routine in PLATON, producing a set of solvent-free diffraction intensities. Crystallographic data for single-crystal X-ray diffraction studies are summarized in Table S1.†
Isothermal titration calorimetry (ITC).
Formation constants and inclusion enthalpies were simultaneously determined for each γ-CD/POM system by the use of an isothermal calorimeter (ITC200, MicroCal Inc., USA). Degassed deionized water solutions were used in both cell (V0 = 202.8 μL) and syringe (40 μL). After the addition of an initial aliquot of 2 μL, 10 aliquots of 3.5 μL of the syringe solution were delivered over 7 s for each injection. The time interval between two consecutive injections was 70 s, which proved to be sufficient for a systematic and complete return to the baseline. The agitation speed was set to 1000 rpm. The resulting heat flow was recorded as a function of time. ITC titrations were realized at three temperatures (288, 298 and 308 K), with 5 mM γ-CD solution in the syringe and 0.25 mM POM solution in the cell. Values and uncertainties of formation constants and inclusion enthalpies were determined by global analysis of the binding isotherms, by means of a dedicated homemade program,71 implementing 1
:
1 and 1
:
1 + 1
:
2 binding polynomials.
Electrochemistry.
Purified water was used throughout. It was obtained by passing water through a RiOs 8 unit followed by a Millipore-Q Academic purification set. All reagents were of high-purity grade and were used as purchased without further purification. Cyclic voltammetry (CV) experiments were carried out with an Methrohm Autolab PGSTAT12 potentiostat/galvanostat associated with a GPES electrochemical analysis system (EcoChemie). Measurements were performed at room temperature in a conventional single compartment cell. A glassy carbon (GC) electrode with a diameter of 3 mm was used as the working electrode. The auxiliary electrode was a Pt plate placed within a fritted-glass isolation chamber and potentials are quoted against a saturated calomel electrode (SCE). The solutions were deaerated thoroughly for at least 30 minutes with pure argon and kept under a positive pressure of this gas during the experiments.
Nuclear magnetic resonance (NMR).
All solution NMR spectra were measured in D2O at 26 °C. 1H NMR spectra were recorded on a Bruker Avance 400 spectrometer at a Larmor frequency of 400.1 MHz, using 5 mm standard NMR tubes. The 1D 1H spectra were recorded with one pulse sequence at 30° flip angle (pulse duration 2.7 μs), using 0.1 s recycle delay, 3 s acquisition time, and 8 number of scans. Translational diffusion measurements were performed using Bruker's “ledbpgs2s” stimulated echo DOSY pulse sequence including bipolar and spoil gradients. Apparent diffusion coefficients were obtained using an adapted algorithm based on the inverse Laplace transform stabilized by maximum entropy.72 Chemical shifts are reported relative to tetramethylsilane (TMS).
Cloud point measurements (CP).
Aqueous solutions containing 60 mM C8E4 and a given amount of POM were prepared in 1 mL glass vials. The vials were placed in a temperature controlled water bath. The heating rate was constant at 1 K min−1 and the CP was detected by visual inspection after the occurrence of turbidity. The choice of the counter-ion has negligible effects on the cloud point evolution.22
Syntheses
Rb4.5Na1.5[H2W12O40]·11H2O.
The synthetic procedure is inspired from the syntheses of tetraalkylammonium (TAA) salts, TAA6[H2W12O40]·9H2O.73 Na2WO4·2H2O (10 g, 30 mmol) is dissolved in 30 mL of deionized water, and the resulting solution is acidified with HCl (6 mol L−1) until pH ∼ 3. The solution is kept under boiling conditions for 24 h and the pH is maintained at 3. After cooling to room temperature, the solution is then filtered, and rubidium chloride (5.5 g, 45 mmol) is added. The white solid product is collected, and finally dried in air. Yield: ∼3.8 g (33%). IR (cm−1): 956 (sh), 935 (s), 896 (s), 878 (s), 766 (vs), 183W NMR: −117.6 ppm; 1H NMR: 5.99 ppm. ICP-OES Anal. Calcd for H24Na1.5O51Rb4.5W12: Na, 1.00; Rb, 11.10; W, 63.66. Found: Na, 0.9; Rb, 10.5; W, 58.0. TGA showed a weight loss of 5.5% in the 20–220 °C temperature range corresponding to the eleven hydration water molecules (calculated 5.7%).
CsK2H2{[BW12O40]·2(C48H80O40)}·29H2O (BW12·2CD).
A mixture of K4.3Na0.7[BW12O40]·17H2O (68 mg; 0.02 mmol) and γ-CD (85 mg; 0.06 mmol) in 3.5 mL of aqueous solution of CsCl (0.05 mol L−1) and KCl (0.1 mol L−1) was stirred until a clear solution was obtained. The solution was then allowed to stand for crystallization in air. Colorless crystals of BW12·2CD were obtained by slow ethanol vapor diffusion into water solution (10 mL vessel containing 2 mL of water solution was placed into a 250 mL vessel with a tight-fitting lid containing 50 mL of ethanol) after 3 days, and were collected, washed with a water/ethanol mixture and dried in air. Yield 37 mg, 30%. The FT-IR spectrum is given in Fig. S23.† ICP-OES Anal. Calcd for BC1.5CsH220K2O149W12: B, 0.17; C, 18.64; K, 1.26; W, 35.66. Found: B, 0.2; C, 18.5; K, 1.2; W, 32.5. EDS showed Cs
:
K
:
W ratio = 1.7
:
2.1
:
12. TGA showed a weight loss of 8.4% in the 20–220 °C temperature range corresponding to the twenty-nine hydration water molecules (calculated 8.4%) and a weight loss of 42% in the 220–700 °C range assigned to the loss of 2 CD (calculated 41.1%).
Na3{[PW12O40]·(C48H80O40)}·10H2O (PW12@CD).
Na1.4H1.6[PW12O40]·12H2O (0.3 g; 0.096 mmol) was dissolved in 10 mL aqueous solution of NaCl (0.2 mol L−1) and γ-CD (0.138 g; 0.096 mmol) was added. The clear solution was stirred for 15 min. The solution was then allowed to stand for crystallization in air. Needle-like colorless crystals of PW12@CD suitable for single crystal X-ray diffraction appeared within 5 days. They were isolated by filtration and washed with cold water. Yield 0.35 g, 76%. The FT-IR spectrum is given in Fig. S24.† ICP-OES Anal. Calcd for C48H100Na3O90PW12: C, 13.03; Na, 1.56; P, 0.70; W, 49.87. Found: C, 12.8; Na, 1.6; P, 0.7; W, 48.9. EDS showed Na
:
P
:
W ratio = 2.7
:
1.1
:
12. TGA showed a weight loss of 3.9% in the 20–220 °C temperature range corresponding to the ten hydration water molecules (calculated 4.1%) and a weight loss of 26.8% in the 220–700 °C range assigned to the loss of 1.3 CD (calculated 27%).
Conflicts of interest
There are no conflicts of interest to declare.
Acknowledgements
This work is supported by a public grant overseen by the French National Research Agency as part of the “Investissements d'Avenir” program (Labex Charm3at, ANR-11-LABX-0039-grant) as well as the CNRS-MOMENTUM. This research is also supported by the China Scholarship Council (CSC) to S. Y. (201904910419). The authors are grateful to Flavien Bourdreux and Cyrielle Rey for their help with elemental analyses.
Notes and references
-
M. Pope, Heteropoly and Isopoly Oxometalates, Springer-Verlag, Berlin Heidelberg, 1983 Search PubMed.
- N. I. Gumerova and A. Rompel, Synthesis, structures and applications of electron-rich polyoxometalates, Nat. Rev. Chem., 2018, 2, 20 CrossRef.
- J. J. Chen, M. D. Symes and L. Cronin, Highly reduced and protonated aqueous solutions of P2W18O626− for on-demand hydrogen generation and energy storage, Nat. Chem., 2018, 10, 1042 CrossRef CAS.
- B. Huang, D. H. Yang and B. H. Han, Application of polyoxometalate derivatives in rechargeable batteries, J. Mater. Chem. A, 2020, 8, 4593 RSC.
- Y. Nishimoto, D. Yokogawa, H. Yoshikawa, K. Awaga and S. Irle, Super-Reduced Polyoxometalates: Excellent Molecular Cluster Battery Components and Semipermeable Molecular Capacitors, J. Am. Chem. Soc., 2014, 136, 9042 CrossRef CAS.
- L. E. VanGelder, A. M. Kosswattaarachchi, P. L. Forrestel, T. R. Cook and E. M. Matson, Polyoxovanadate-alkoxide clusters as multielectron charge carriers for symmetric nonaqueous redox flow batteries, Chem. Sci., 2018, 9, 1692 RSC.
- H. Wang, S. Hamanaka, Y. Nishimoto, S. Irle, T. Yokoyama, H. Yoshikawa and K. Awaga, In Operand X-ray Absorption Fine Structure Studies of Polyoxometalate Molecular Cluster Batteries: Polyoxometalates as Electron Sponges, J. Am. Chem. Soc., 2012, 134, 4918 CrossRef CAS.
- H. N. Wang, M. Zhang, A. M. Zhang, F. C. Shen, X. K. Wang, S. N. Sun, Y. J. Chen and Y. Q. Lan, Polyoxometalate-Based Metal-Organic Frameworks with Conductive Polypyrrole for Supercapacitors, ACS Appl. Mater. Interfaces, 2018, 10, 32265 CrossRef CAS.
- B. R. Limoges, R. J. Stanis, J. A. Turner and A. M. Herring, Electrocatalyst materials for fuel cells based on the polyoxometalates PMo12-nVnO40(3+n)− (n=0-3), Electrochim. Acta, 2005, 50, 1169 CrossRef CAS.
- W. Liu, W. Mu, M. J. Liu, X. D. Zhang, H. L. Cai and Y. L. Deng, Solar-induced direct biomass-to-electricity hybrid fuel cell using polyoxometalates as photocatalyst and charge carrier, Nat. Commun., 2014, 5, 8 CrossRef.
- M. Blasco-Ahicart, J. Soriano-Lopez, J. J. Carbo, J. M. Poblet and J. R. Galan-Mascaros, Polyoxometalate electrocatalysts based on earth-abundant metals for efficient water oxidation in acidic media, Nat. Chem., 2018, 10, 24 CrossRef CAS.
- M. Bonchio, Z. Syrgiannis, M. Burian, N. Marino, E. Pizzolato, K. Dirian, F. Rigodanza, G. A. Volpato, G. La Ganga, N. Demitri, S. Berardi, H. Amenitsch, D. M. Guldi, S. Caramori, C. A. Bignozzi, A. Sartorel and M. Prato, Hierarchical organization of perylene bisimides and polyoxometalates for photo-assisted water oxidation, Nat. Chem., 2019, 11, 146 CrossRef CAS.
- G. Paille, M. Gomez-Mingot, C. Roch-Marchal, M. Haouas, Y. Benseghir, T. Pino, M. H. Ha-Thi, G. Landrot, P. Mialane, M. Fontecave, A. Dolbecq and C. Mellot-Draznieks, Thin Films of Fully Noble Metal-Free POM@MOF for Photocatalytic Water Oxidation, ACS Appl. Mater. Interfaces, 2019, 11, 47837 CrossRef CAS.
- J. Tourneur, B. Fabre, G. Loget, A. Vacher, C. Meriadec, S. Ababou-Girard, F. Gouttefangeas, L. Joanny, E. Cadot, M. Haouas, N. Leclerc-Laronze, C. Falaise and E. Guillon, Molecular and Material Engineering of Photocathodes Derivatized with Polyoxometalate-Supported {Mo3S4} HER Catalysts, J. Am. Chem. Soc., 2019, 141, 11954 CrossRef CAS.
- M. Stuckart and K. Y. Monakhov, Polyoxometalates as
components of supramolecular assemblies, Chem. Sci., 2019, 10, 4364 RSC.
- S. Chakraborty, A. S. Grego, S. Garai, M. Baranov, A. Muller and I. A. Weinstock, Alcohols as Latent Hydrophobes: Entropically Driven Uptake of 1,2-Diol Functionalized Ligands by a Porous Capsule in Water, J. Am. Chem. Soc., 2019, 141, 9170 CrossRef.
- S. Kopilevich, H. Gottlieb, K. Keinan-Adamsky, A. Muller and I. A. Weinstock, The Uptake and Assembly of Alkanes within a Porous Nanocapsule in Water: New Information about Hydrophobic Confinement, Angew. Chem., Int. Ed., 2016, 55, 4476 CrossRef CAS.
- A. Misra, K. Kozma, C. Streb and M. Nyman, Beyond Charge Balance: Counter-Cations in Polyoxometalate Chemistry, Angew. Chem., Int. Ed., 2020, 59, 596 CrossRef CAS.
- N. Watfa, D. Melgar, M. Haouas, F. Taulelle, A. Hijazi, D. Naoufal, J. B. Avalos, S. Floquet, C. Bo and E. Cadot, Hydrophobic Effect as a Driving Force for Host-Guest Chemistry of a Multi-Receptor Keplerate-Type Capsule, J. Am. Chem. Soc., 2015, 137, 5845 CrossRef CAS.
- P. C. Yin, D. Li and T. B. Liu, Solution behaviors and self-assembly of polyoxometalates as models of macroions and amphiphilic polyoxometalate-organic hybrids as novel surfactants, Chem. Soc. Rev., 2012, 41, 7368 RSC.
- T. Buchecker, P. Schmid, I. Grillo, S. Prevost, M. Drechsler, O. Diat, A. Pfitzner and P. Bauduin, Self-Assembly of Short Chain PoIy-N-isopropylacrylamid Induced by Superchaotropic Keggin Polyoxometalates: From Globules to Sheets, J. Am. Chem. Soc., 2019, 141, 6890 CrossRef CAS.
- T. Buchecker, P. Schmid, S. Renaudineau, O. Diat, A. Proust, A. Pfitzner and P. Bauduin, Polyoxometalates in the Hofmeister series, Chem. Commun., 2018, 54, 1833 RSC.
- D. Kobayashi, H. Nakahara, O. Shibata, K. Unoura and H. Nabika, Interplay of Hydrophobic and Electrostatic Interactions between Polyoxometalates and Lipid Molecules, J. Phys. Chem. C, 2017, 121, 12895 CrossRef CAS.
- A. Malinenko, A. Jonchere, L. Girard, S. Parres-Maynadie, O. Diat and P. Bauduin, Are Keggin's POMs Charged Nanocolloids or Multicharged Anions?, Langmuir, 2018, 34, 2026 CrossRef CAS.
- M. A. Moussawi, M. Haouas, S. Floquet, W. E. Shepard, P. A. Abramov, M. N. Sokolov, V. P. Fedin, S. Cordier, A. Ponchel, E. Monflier, J. Marrot and E. Cadot, Nonconventional Three-Component Hierarchical Host-Guest Assembly Based on Mo-Blue Ring-Shaped Giant Anion, gamma-Cyclodextrin, and Dawson-type Polyoxometalate, J. Am. Chem. Soc., 2017, 139, 14376 CrossRef CAS.
- M. A. Moussawi, N. Leclerc-Laronze, S. Floquet, P. A. Abramov, M. N. Sokolov, S. Cordier, A. Ponchel, E. Monflier, H. Bricout, D. Landy, M. Haouas, J. Marrot and E. Cadot, Polyoxometalate, Cationic Cluster, and gamma-Cyclodextrin: From Primary Interactions to Supramolecular Hybrid Materials, J. Am. Chem. Soc., 2017, 139, 12793 CrossRef CAS.
- B. Naskar, O. Diat, V. Nardello-Rataj and P. Bauduin, Nanometer-Size Polyoxometalate Anions Adsorb Strongly on Neutral Soft Surfaces, J. Phys. Chem. C, 2015, 119, 20985 CrossRef CAS.
- T. J. Paul, T. N. Parac-Vogt, D. Quinonero and R. Prabhakar, Investigating Polyoxometalate-Protein Interactions at Chemically Distinct Binding Sites, J. Phys. Chem. B, 2018, 122, 7219 CrossRef CAS.
- A. Solé-Daura, J. M. Poblet and J. J. Carbo, Structure–Activity Relationships for the Affinity of Chaotropic Polyoxometalate Anions towards Proteins, Chem. – Eur. J., 2020, 26, 5799 CrossRef.
- Y. L. Wu, R. F. Shi, Y. L. Wu, J. M. Holcroft, Z. C. Liu, M. Frasconi, M. R. Wasielewski, H. Li and J. F. Stoddart, Complexation of Polyoxometalates with Cyclodextrins, J. Am. Chem. Soc., 2015, 137, 4111 CrossRef CAS.
- K. I. Assaf and W. M. Nau, The Chaotropic Effect as an Assembly Motif in Chemistry, Angew. Chem., Int. Ed., 2018, 57, 13968 CrossRef CAS.
- K. I. Assaf, M. S. Ural, F. F. Pan, T. Georgiev, S. Simova, K. Rissanen, D. Gabel and W. M. Nau, Water Structure Recovery in Chaotropic Anion Recognition: High-Affinity Binding of Dodecaborate Clusters to -Cyclodextrin, Angew. Chem., Int. Ed., 2015, 54, 6852 CrossRef CAS.
- M. Hohenschutz, I. Grillo, O. Diat and P. Bauduin, How Nano-Ions Act Like Ionic Surfactants, Angew. Chem., Int. Ed., 2020, 59, 8084 CrossRef CAS.
- A. A. Ivanov, C. Falaise, D. Landy, M. Haouas, Y. V. Mironov, M. A. Shestopalov and E. Cadot, Tuning the chaotropic effect as an assembly motif through one-electron transfer in a rhenium cluster, Chem. Commun., 2019, 55, 9951 RSC.
- G. Zhang, B. Keita, C. T. Craescu, S. Miron, P. de Oliveira and L. Nadjo, Polyoxometalate binding to human serum albumin: A thermodynamic and spectroscopic approach, J. Phys. Chem. B, 2007, 111, 11253 CrossRef CAS.
- D. Prochowicz, A. Kornowicz and J. Lewinski, Interactions of Native Cyclodextrins with Metal Ions and Inorganic Nanoparticles: Fertile Landscape for Chemistry and Materials Science, Chem. Rev., 2017, 117, 13461 CrossRef CAS.
- C. Falaise, M. A. Moussawi, S. Floquet, P. A. Abramov, M. N. Sokolov, M. Haouas and E. Cadot, Probing Dynamic Library of Metal-Oxo Building Blocks with gamma-Cyclodextrin, J. Am. Chem. Soc., 2018, 140, 11198 CrossRef CAS.
- P. Su, A. J. Smith, J. Warneke and J. Laskin, Gas-Phase Fragmentation of Host-Guest Complexes of Cyclodextrins and Polyoxometalates, J. Am. Soc. Mass Spectrom., 2019, 30, 1934 CrossRef CAS.
- Y. X. Fan, S. F. Lu and J. Cao, A novel inorganic-organic hybrid complex between polyoxometalate and cyclodextrin: Synthesis, structure and catalytic activity, Int. J. Mass Spectrom., 2019, 435, 163 CrossRef CAS.
- Y. X. Fan, Y. Zhang, Q. D. Jia, J. Cao and W. J. Wu, The Stabilizing Role of Cyclodextrins on Keggin Phosphotungstic Acid by Complexation Unveiled by Electrospray Mass Spectrometry, Mass Spectrom. Lett., 2015, 6, 13 CrossRef CAS.
- L. B. Ni, H. Li, H. J. Xu, C. Shen, R. Z. Liu, J. Xie, F. M. Zhang, C. Chen, H. X. Zhao, T. F. Zuo and G. W. Diao, Self-Assembled Supramolecular Polyoxometalate Hybrid Architecture as a Multifunctional Oxidation Catalyst, ACS Appl. Mater. Interfaces, 2019, 11, 38708 CrossRef CAS.
- B. Pacaud, L. Leclercq, J. F. Dechezelles and V. Nardello-Rataj, Hybrid Core-Shell Nanoparticles by “Plug and Play” Self-Assembly, Chem. – Eur. J., 2018, 24, 17672 CrossRef CAS.
- B. Zhang, W. M. Guan, F. F. Yin, J. X. Wang, B. Li and L. X. Wu, Induced chirality and reversal of phosphomolybdate cluster via modulating its interaction with cyclodextrins, Dalton Trans., 2018, 47, 1388 RSC.
- P. Yang, W. L. Zhao, A. Shkurenko, Y. Belmabkhout, M. Eddaoudi, X. C. Dong, H. N. Alshareef and N. M. Khashab, Polyoxometalate-Cyclodextrin Metal-Organic Frameworks: From Tunable Structure to Customized Storage Functionality, J. Am. Chem. Soc., 2019, 141, 1847 CrossRef CAS.
- Z. G. Jiang, W. T. Mao, Y. W. Huang, Y. Wang, X. J. Wang and C. H. Zhan, Nonconventional Host−Guest Cubic Assembly Based on γ-Cyclodextrin and Keggin-type Polyoxometalate, Nanoscale, 2020, 12, 10166 RSC.
- A. A. Ivanov, C. Falaise, K. Laouer, F. Hache, P. Changenet, Y. V. Mironov, D. Landy, Y. Molard, S. Cordier, M. A. Shestopalov, M. Haouas and E. Cadot, Size-Exclusion Mechanism Driving Host-Guest Interactions between Octahedral Rhenium Clusters and Cyclodextrins, Inorg. Chem., 2019, 58, 13184 CrossRef CAS.
- S. Himeno, M. Takamoto, R. Santo and A. Ichimura, Redox properties and basicity of Keggin-type polyoxometalate complexes, Bull. Chem. Soc. Jpn., 2005, 78, 95 CrossRef CAS.
- K. Kamata and K. Sugahara, Base Catalysis by Mono- and Polyoxometalates, Catalysts, 2017, 7, 24 CrossRef.
- M. V. Rekharsky and Y. Inoue, Complexation thermodynamics of cyclodextrins, Chem. Rev., 1998, 98, 1875 CrossRef CAS.
- K. I. Assaf, B. Begaj, A. Frank, M. Nilam, A. S. Mougharbel, U. Kortz, J. Nekvinda, B. Gruner, D. Gabel and W. M. Nau, High-Affinity Binding of Metallacarborane Cobalt Bis(dicarbollide) Anions to Cyclodextrins and Application to Membrane Translocation, J. Org. Chem., 2019, 84, 11790 CrossRef CAS.
- K. I. Assaf, J. Holub, E. Bernhardt, J. M. Oliva-Enrich, M. I. F. Perez, M. Canle, J. A. Santaballa, J. Fanfrlik, D. Hnyk and W. M. Nau, Face-Fusion of Icosahedral Boron Hydride Increases Affinity to gamma-Cyclodextrin: closo,closo- B21H18− as an Anion with Very Low Free Energy of Dehydration, ChemPhysChem, 2020, 21, 971 CrossRef CAS.
- T. Konishi, K. Kodani, T. Hasegawa, S. Ogo, S. X. Guo, J. F. Boas, J. Zhang, A. M. Bond and T. Ueda, Impact of the lithium cation on the voltammetry and spectroscopy of [XVM11O40]n− (X = P, As (n = 4), S (n = 3); M = Mo, W): Influence of charge and addenda and hetero atoms, Inorg. Chem., 2020, 59, 10522 CrossRef CAS.
- S. X. Guo, A. W. A. Mariotti, C. Schlipf, A. M. Bond and A. G. Wedd, A Systematic approach to the simulation of the voltammetric reduction of alpha-SiW12O404− in buffered aqueous electrolyte media and acetonitrile, J. Electroanal. Chem., 2006, 591, 7 CrossRef CAS.
- M. Sadakane and E. Steckhan, Electrochemical properties of polyoxometalates as electrocatalysts, Chem. Rev., 1998, 98, 219 CrossRef CAS.
- V. Gutmann, Emperical parameters for donor and acceptor properties of solvents, Electrochim. Acta, 1976, 21, 661 CrossRef CAS.
-
A. J. Bard and L. R. Faulkener, Electrochemical Methods: Fundamentals and Applications, John Wiley & Sons ed., New York, 2001 Search PubMed.
- A. A. Ivanov, C. Falaise, P. A. Abramov, M. A. Shestopalov, K. Kirakci, K. Lang, M. A. Moussawi, M. N. Sokolov, N. G. Naumov, S. Floquet, D. Landy, M. Haouas, K. A. Brylev, Y. V. Mironov, Y. Molard, S. Cordier and E. Cadot, Host-Guest Binding Hierarchy within Redox- and Luminescence-Responsive Supramolecular Self-Assembly Based on Chalcogenide Clusters and gamma-Cyclodextrin, Chem. – Eur. J., 2018, 24, 13467 CrossRef CAS.
-
Y. Cohen, L. Avram, T. Evan-Salem, S. Slovak, N. Shemesh and L. Frish, in Analytical Methods in Supramolecular Chemistry, ed. C. A. Schalley, John Wiley & Sons, Ltd, 2012, pp. 197 Search PubMed.
- M. R. Antonio and M. K. Bera, pH-Dependent Interactions between Keggin Heteropolyanions in Dilute Solutions, Eur. J. Inorg. Chem., 2019, 367 CrossRef CAS.
- C. Drummond, L. Perez-Fuentes and D. Bastos-Gonzalez, Can Polyoxometalates Be Considered as Superchaotropic Ions?, J. Phys. Chem. C, 2019, 123, 28744 CrossRef.
- M. K. Bera and M. R. Antonio, Crystallization
of Keggin Heteropolyanions via a Two-Step Process in Aqueous Solutions, J. Am. Chem. Soc., 2016, 138, 7282 CrossRef CAS.
- M. K. Bera, B. F. Qiao, S. Seifert, B. P. Burton-Pye, M. O. de la Cruz and M. R. Antonio, Aggregation of Heteropolyanions in Aqueous Solutions Exhibiting Short-Range Attractions and Long-Range Repulsions, J. Phys. Chem. C, 2016, 120, 1317 CrossRef CAS.
- H. Fletcher, C. C. Allen, R. C. Burns and D. C. Craig, Pentapotassium dodecatungstoborate(III) hexadecahydrate, Acta Crystallogr., Sect. C: Cryst. Struct. Commun., 2001, 57, 505 CrossRef CAS.
- C. Rocchicciolideltcheff, M. Fournier, R. Franck and R. Thouvenot, Vibrational investigations of polyoxometalates. 2. Evidence for anion anion interactions in molybdenum(VI) and tungsten(VI) compounds related to the Keggin structure, Inorg. Chem., 1983, 22, 207 CrossRef CAS.
-
P. Souchay, Ions Minéraux Condensés, Masson & Cie ed., Paris, 1969 Search PubMed.
-
A. Tézé and G. Hervé, in Inorg. Synth, John Wiley & Sons, 1990, vol. 27, p. 85 Search PubMed.
- H. Wu, Contribution to the chemistry of phosphomolybdic acids, phosphotungstic acids, and allied substances, J. Biol. Chem., 1920, 43, 189 CAS.
- O. V. Dolomanov, L. J. Bourhis, R. J. Gildea, J. A. K. Howard and H. Puschmann, OLEX2: a complete structure solution, refinement and analysis program, J. Appl. Crystallogr., 2009, 42, 339 CrossRef CAS.
- G. M. Sheldrick, SHELXT - Integrated space-group and crystal-structure determination, Acta Crystallogr., Sect. A: Found. Adv., 2015, 71, 3 CrossRef.
- G. M. Sheldrick, Crystal structure refinement with SHELXL, Acta Crystallogr., Sect. C: Struct. Chem., 2015, 71, 3 Search PubMed.
- E. Bertaut and D. Landy, Improving ITC studies of cyclodextrin inclusion compounds by global analysis of conventional and non-conventional experiments, Beilstein J. Org. Chem., 2014, 10, 2630 CrossRef.
- M. A. Delsuc and T. E. Malliavin, Maximum entropy processing of DOSY NMR spectra, Anal. Chem., 1998, 70, 2146 CrossRef CAS.
- M. Asami, H. Ichida and Y. Sasaki, The structure of hexakis(tetramethylammonium)dihydrogendodecatungstate enneahydrate, ((CH3)4N)6H2W12O40·9H2O, Acta Crystallogr., Sect. C: Cryst. Struct. Commun., 1984, 40, 35 CrossRef.
Footnote |
† Electronic supplementary information (ESI) available: Additional experimental data (single crystal XRD, ITC, electrochemistry, NMR, SAXS and FT-IR spectroscopy). CCDC 2015681 and 2015682. For ESI and crystallographic data in CIF or other electronic format see DOI: 10.1039/d0qi00902d |
|
This journal is © the Partner Organisations 2021 |