DOI:
10.1039/D1PY01114F
(Paper)
Polym. Chem., 2021,
12, 5842-5850
One-pot synthesis and aqueous solution properties of pH-responsive schizophrenic diblock copolymer nanoparticles prepared via RAFT aqueous dispersion polymerization†
Received
16th August 2021
, Accepted 20th September 2021
First published on 29th September 2021
Abstract
Schizophrenic diblock copolymers can form two types of nanoparticles in aqueous solution, with such self-assembly typically being driven by a change in solution temperature, solution pH or salt concentration. In the present study, we report the first wholly aqueous one-pot synthesis of a doubly pH-responsive schizophrenic diblock copolymer. This is achieved using RAFT aqueous dispersion polymerization, which is an example of polymerization-induced self-assembly (PISA). First, 2-(diethylamino)ethyl methacrylate (DEA) is homopolymerized in its protonated form at pH 2 to produce a cationic polyelectrolytic precursor. Subsequently, the RAFT aqueous dispersion polymerization of 2-carboxyethyl acrylate (CEA) is conducted to produce sterically-stabilized diblock copolymer nanoparticles in which the cationic PDEA block acts as the hydrophilic stabilizer block and the neutral PCEA block forms the hydrophobic core. On addition of sufficient NaOH, the PCEA becomes highly anionic at pH 10 and hence acts as a stabilizer block while the deprotonated PDEA block forms the hydrophobic core. Characterizing such polyampholytes via aqueous gel permeation chromatography is challenging. Thus a selective methylation protocol was developed to esterify the anionic carboxylate groups in the PCEA block to enable GPC analysis using THF as an eluent. However, optimization of the reaction conditions was required because using too large an excess of the trimethylsilyldiazomethane reagent led to unwanted quaternization of the tertiary amine groups on the PDEA block, which prevented meaningful GPC analysis. The aqueous self-assembly behaviour of a series of PDEA–PCEA diblock copolymers was examined using transmission electron microscopy, dynamic light scattering, 1H NMR spectroscopy and aqueous electrophoresis.
Introduction
The micellar self-assembly of block copolymers in solution has been studied for more than fifty years.1,2 In 1998, the first example of an AB diblock copolymer that could form either A-core or B-core micelles in aqueous media was reported by Bütün et al.3,4 This study involved the synthesis of poly(2-(N-morpholino)ethyl methacrylate)–poly(2-(diethylamino)ethyl methacrylate) (PMEMA–PDEA) diblock copolymers via group transfer polymerization (GTP), which works well for methacrylic monomers in dry THF at ambient temperature. In 2001, Liu and co-workers reported that poly(propylene oxide)–poly(2-(diethylamino)ethyl methacrylate) (PPO–PDEA) diblock copolymers could form two types of micelles by adjusting the solution pH and temperature but neither micellar state was stable at ambient temperature.5 This second study was the first to coin the phrase ‘schizophrenic’ to describe such stimulus-responsive diblock copolymers.6 Subsequently, Laschewsky et al.7 prepared the first (meth)acrylamide example of a doubly thermoresponsive schizophrenic diblock copolymer via RAFT solution polymerization.8–10 However, such copolymers had relatively broad molecular weight distributions and also suffered from homopolymer contamination. In the same year, Weaver et al.11 reported a second example of a doubly thermoresponsive all-methacrylic schizophrenic diblock copolymer. In this case, GTP afforded relatively well-defined copolymer chains with little or no homopolymer contamination. Subsequently, various examples of doubly thermoresponsive schizophrenic diblock copolymers have been extensively studies by Papadakis et al.12–17
Of particular relevance to the present study, in 2002 Liu et al.18 designed a poly(4-vinyl benzoic acid)–poly(2-(diethylamino)ethyl methacrylate) (PVBA–PDEA) diblock copolymer that underwent spontaneous self-assembly in aqueous solution simply by adjusting the solution pH at room temperature. Unlike the poly(2-(dimethylamino)ethyl methacrylate)–poly(methacrylic acid) (PDMA–PMAA) diblock copolymers previously reported by several research groups,19–22 both the weakly acidic PVBA block and the weakly basic PDEA block proved to be sufficiently hydrophobic in their neutral form to produce PVBA-core micelles at low pH or PDEA-core micelles at high pH, respectively.18
More recently, schizophrenic diblock copolymer nanoparticles have been evaluated as stimulus-responsive Pickering emulsifiers. For example, Ranka et al.23 utilized a doubly thermoresponsive schizophrenic diblock copolymer to form stable emulsions at elevated temperature, with macroscopic phase separation occurring on cooling to ambient temperature. In principle, such schizophrenic nanoparticles may find applications in diverse fields such as enhanced oil recovery or catalysis,24,25 whereas doubly pH-responsive schizophrenic nanoparticles may prove to be useful in the context of pigment dispersion.26
Over the past decade or so, polymerization-induced self-assembly (PISA) has become widely recognised as a powerful technique for the synthesis of a wide range of functional block copolymer nano-objects.27–37 Most pertinently, Canning et al. reported the aqueous PISA synthesis of doubly pH-responsive diblock copolymers directly in the form of sterically-stabilized nanoparticles.38 More specifically, a PDEA homopolymer was first prepared via RAFT solution polymerization of DEA in THF. Subsequently, this precursor was dissolved in acidic aqueous solution and used to conduct the statistical copolymerization of benzyl methacrylate (BzMA) with methacrylic acid (MAA) via RAFT aqueous emulsion polymerization. 1H NMR spectroscopy studies confirmed that the acidic P(BzMA-stat-MAA) block became desolvated at low pH, while the basic PDEA block became desolvated at high pH. These observations were consistent with TEM, DLS and aqueous electrophoresis observations, which indicated the formation of cationic and anionic spherical nanoparticles, respectively.39 Moreover, suitable rhodamine- and fluorescein-based comonomers were statistically copolymerized into the polybase and polyacid blocks respectively in order to produce ‘self-reporting’ pH-responsive nanoparticles.39 However, the feasibility of developing a wholly aqueous one-pot formulation for schizophrenic diblock copolymers was not explored in this prior study.39
Herein we report the first wholly aqueous one-pot synthesis of doubly pH-responsive poly(2-(diethylamino)ethyl methacrylate)–poly(2-carboxyethyl acrylate) (PDEA–PCEA) diblock copolymers directly in the form of sterically-stabilized nanoparticles. This is achieved via RAFT aqueous solution polymerization of DEA followed by the RAFT aqueous dispersion polymerization of CEA (see Scheme 1). A suitably selective methylation protocol is optimized to enable the characterization of such polyampholytes using gel permeation chromatography (GPC). Finally, the schizophrenic behavior of such nanoparticles in aqueous solution is examined using transmission electron microscopy (TEM), dynamic light scattering (DLS), 1H NMR spectroscopy and aqueous electrophoresis.
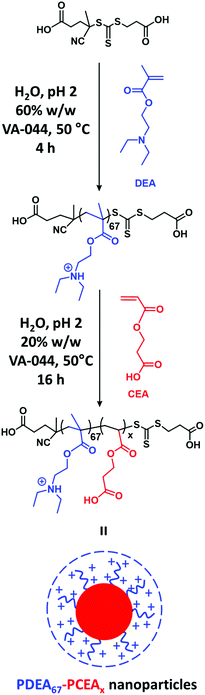 |
| Scheme 1 Wholly aqueous one-pot synthesis of a series of PDEA67–PCEAx diblock copolymer nanoparticles (where x = 50–200) via (i) RAFT aqueous solution polymerization of DEA at pH 2 followed by (ii) RAFT aqueous dispersion polymerization of CEA at the same pH to form cationic sterically-stabilized nanoparticles comprising PCEA cores. | |
Experimental
Materials
4-((((2-Carboxyethyl)thio)carbonothioyl)thio)-4-cyanopentanoic acid (CECPA) was purchased from Boron Molecular (Melbourne, Australia). 2-(Diethylamino)ethyl methacrylate (DEA), 2-carboxyethyl acrylate (CEA) and trimethylsilyldiazomethane (TMSDAM; supplied as a 2.0 M solution in hexane) were purchased from Sigma-Aldrich (Dorset, UK) and were used as received. 2,2′-Azobis(2-(2-imidazolin-2-yl)propane) dihydrochloride (VA-044) was purchased from Wako Pure Chemical Industries (Japan). CD3OD and CD2Cl2 were purchased from Goss Scientific Instruments Ltd (Cheshire, UK). CDCl3, D2O, KCl, sodium deuteroxide (NaOD) and deuterium chloride (DCl) were purchased from Sigma-Aldrich (Dorset, UK). All other solvents were purchased from Fisher Scientific (Loughborough, UK) and were used as received. Deionized water was used for all experiments and the solution pH was adjusted using either HCl or NaOH.
One-pot synthesis of poly(2-(diethylamino)ethyl methacrylate)–poly(2-carboxyethyl acrylate) (PDEA–PCEA) diblock copolymer
A typical protocol for the wholly aqueous one-pot synthesis of a PDEA67–PCEA100 zwitterionic diblock copolymer was conducted as follows. DEA (0.50 g, 2.70 mmol), CECPA (12.4 mg, 0.0403 mmol; target degree of polymerization, DP = 67), VA-044 (4.30 mg, 0.013 mmol; CECPA/VA-044 molar ratio = 3.0), 12 M HCl (0.232 g, 2.70 mmol) and deionized water (0.775 g) were added in turn to a 50 ml round-bottomed flask and the resulting mixture was stirred thoroughly to afford a 40% w/w acidic aqueous solution (pH 2), which was then purged for 30 min with nitrogen prior to heating up to 50 °C. After 100 min, the DEA polymerization had reached more than 99% conversion as determined by 1H NMR spectroscopy. In separate vials, CEA (0.58 g, 4.03 mmol; target DP = 100), VA-044 (4.34 mg, 0.0134 mmol; CECPA/VA-044 molar ratio = 3.0) and deionized water (3.61 g, target solids concentration = 20% w/w) were purged with nitrogen for 30 min. These degassed components were then added under a nitrogen atmosphere and the second-stage polymerization was allowed to proceed for 8–16 h at 50 °C. This one-pot protocol yielded a pale yellow dispersion of PCEA-core diblock copolymer nanoparticles, with a final CEA conversion of more than 99% as judged by 1H NMR spectroscopy. Alternative diblock copolymer compositions were targeted by fixing the conditions used to prepare the PDEA67 block and varying the amount of added CEA monomer, HCl and water required to achieve the desired PCEA DP at 20% w/w solids.
1H NMR spectroscopy
Most 1H NMR spectra were recorded using a 400 MHz Bruker Avance-400 spectrometer. The NMR solvents were CD3OD, CD2Cl2, CDCl3 or D2O and typically 64 scans were averaged per spectrum.
For in situ NMR studies of the RAFT aqueous solution polymerization of DEA at 40% w/w solids, a 0.75 mL aliquot of the reaction mixture (see above for formulation details) was placed in an NMR tube equipped with a J-Young tap and containing an external standard (0.10 M pyridine dissolved in 1,1,2,2,tetrachloroethane-d2, which also served as a lock solvent) within a separate inner tube. This double tube assembly was inserted into a Bruker AVANCE III HD spectrometer operating at 500.13 MHz and a reference spectrum was first recorded at 25 °C (no polymerization) prior to heating up to 50 °C. Spectra were recorded at 5 min intervals for 2 h during the RAFT aqueous solution polymerization of DEA. However, for the subsequent RAFT aqueous dispersion polymerization of CEA at 20% w/w solids (targeting a PDEA67–PCEA135 diblock copolymer), spectra were recorded at 15 min intervals for 8 h. All spectra were phase-adjusted and baseline-corrected using Bruker TopSpin 3.1 software.
Dynamic light scattering
Dilute (0.10% w/w) aqueous copolymer dispersions were analyzed at 25 °C in the presence of 0.50 M KCl using a Malvern NanoZS instrument. Scattered light was detected at 173° and hydrodynamic diameters were calculated using the Stokes–Einstein equation, which assumes dilute non-interacting spheres. Data were averaged over three consecutive measurements comprising eleven runs per measurement.
Aqueous electrophoresis
The same Malvern NanoZS instrument was used to determine electrophoretic mobilities at 25 °C, from which zeta potentials were calculated by cumulants analysis of the experimental correlation function using Dispersion Technology Software version 6.20. Measurements (averaged over twenty runs) were made on 0.05–0.10% w/w aqueous dispersions in the presence of 1 mM KCl as background salt over a range of solution pH. In each case, the solution pH was gradually lowered by adding 0.1 M HCl.
Transmission electron microscopy
Copper/palladium grids were surface-coated in-house to produce a thin film of amorphous carbon before being plasma glow-discharged for 40 seconds to produce a hydrophilic surface. Typically, a 1 μL droplet of a 0.1% w/w aqueous copolymer dispersion in 0.5 M KCl (solution pH adjusted using either 0.1 M HCl or 0.1 M NaOH) was placed onto a TEM grid for 30 seconds, then stained using a 0.75% w/v aqueous solution of either phosphotungstic acid or uranyl formate for 30 seconds. Excess stain was removed by careful blotting with filter paper and each grid was then dried using a vacuum hose. TEM images were recorded using a Philips CM100 instrument operating at 100 kV and equipped with a Gatan 1k CCD camera. ImageJ software was used to calculate number-average diameters and standard deviations from TEM images (at least 100 nanoparticles were analyzed per sample).
Methylation protocol prior to GPC analysis
Prior to GPC analysis, PDEA–PCEA diblock copolymers were derivatized by selective methylation of the pendent carboxylic acid groups in the PCEA block. The following protocol is representative. Excess TMSDAM (33.4 μL; 86.4 μmol) was added dropwise to a PDEA67–PCEA160 diblock copolymer (40 mg; 43.2 μmol CEA residues; TMSDAM/CEA molar ratio = 2.0) dissolved in a 2
:
3 methanol/toluene solution (5.0 mL) until the yellow color persisted. This reaction solution was then placed at the back of a fumehood and stirred for up to 48 h at 20 °C until all the solvent had evaporated. The mean degree of methylation was determined by 1H NMR spectroscopy by comparing the integrated methoxy proton signal of the methylated PCEA block at 3.7 ppm to that of the oxymethylene protons of the PCEA block at 4.3 ppm. In preliminary studies, a TMSDAM/CEA molar ratio of ten was employed but this relatively large excess led to an unwanted side-reaction with the PDEA block (see below for further details).
THF GPC analysis
The GPC set-up consisted of an Agilent 1260 Infinity II GPC/SEC system operating at 30 °C equipped with an autosampler and two 5 μm PL Mixed-C columns connected to a refractive index detector. The mobile phase was HPLC-grade THF at a flow rate of 1.0 mL min−1. Molecular weights were calculated using a series of near-monodisperse PMMA calibration standards. All copolymers were modified by selective methylation of CEA residues under mild conditions (see above for details of the derivatization protocol) prior to GPC analysis to ensure their solubility in THF.
Results and discussion
The synthesis of PDEAx–PCEAy diblock copolymers was initially attempted by conducting the RAFT solution polymerization of DEA in THF. This PDEA precursor was then isolated and purified prior to the RAFT polymerization of CEA in aqueous solution. However, this two-step approach consistently resulted in significant PDEA homopolymer contamination. Subsequently, a much more efficient wholly aqueous one-pot protocol was developed (see Scheme 1)26,40,41 that minimized this problem. This involved conducting the initial DEA polymerization at pH 2 using CECPA as a RAFT chain transfer agent (CTA). CECPA was preferred to other RAFT agents because it has appreciably higher water solubility.41 The resulting cationic PDEA chains are molecularly dissolved in their protonated form and the subsequent CEA polymerization is performed at the same pH by adding CEA after 100 min. Importantly, the CEA monomer is fully soluble in the acidic reaction solution and the growing PCEA chains become insoluble under such conditions (see Fig. S1†). Thus this aqueous PISA formulation is an interesting new example of a RAFT aqueous dispersion polymerization.42 In recent related studies involving the synthesis of polyampholytic diblock copolymers via RAFT aqueous solution polymerization, we examined whether it is better to prepare the cationic block first or the anionic block first.26,43 For the present aqueous PISA formulation, it is perhaps worth emphasizing that the methacrylic DEA monomer must be first. This is because if the acrylic CEA monomer were to be polymerized first instead, only very poor blocking efficiencies would be obtained owing to the highly inefficient chain transfer that occurs when switching from acrylic to methacrylic monomers.44–46
1H NMR spectroscopy studies of the polymerization kinetics confirm that the DEA polymerization is essentially complete within 100 min at 50 °C (see Fig. 1a and b). At this point, CEA monomer is added under a nitrogen atmosphere. Visual inspection indicated that the initial reaction mixture is transparent, as expected for an aqueous dispersion polymerization formulation (see Fig. S2†). The onset of turbidity after approximately 45 min indicates micellar nucleation, which occurs at a CEA monomer conversion of 37% (see Fig. 1b). When targeting a PDEA67–PCEA135 diblock copolymer, this corresponds to a critical PCEA DP of 50. The semi-logarithmic kinetic plot for this RAFT aqueous dispersion polymerization indicates first-order kinetics up to 90% CEA conversion (see Fig. S3†). However, no discernible rate acceleration was observed at the point of micellar nucleation. DLS studies indicate the formation of somewhat polydisperse nascent spherical nanoparticles with a z-average diameter of 33 nm (DLS polydispersity = 0.25). These monomer-swollen nanoparticles grow and become much more uniform in size as the CEA polymerization proceeds. After 8 h at 50 °C, 1H NMR studies confirm that the CEA conversion is more than 99% and DLS studies indicate a final z-average diameter of 43 nm (DLS polydispersity = 0.03), see Fig. 1b. Clearly, this one-pot aqueous synthesis protocol is rather efficient since each monomer is more or less fully consumed (see Fig. 1a).
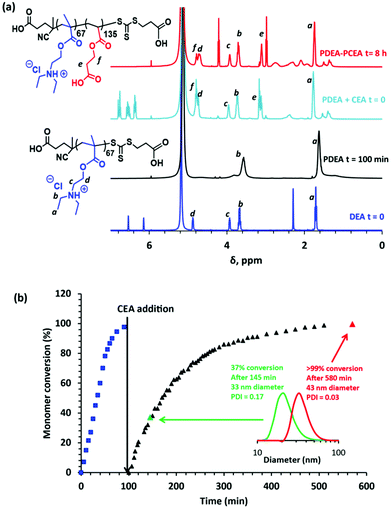 |
| Fig. 1
In situ
1H NMR spectroscopy studies recorded during the wholly aqueous one-pot synthesis of PDEA67–PCEA135 diblock copolymer nanoparticles via the RAFT dispersion polymerization of CEA at pH 2. (a) Typical 1H NMR spectra recorded at various stages of this aqueous PISA synthesis. (b) Monomer conversion vs. time curves indicating that essentially full DEA conversion is achieved within 100 min at 50 °C for the initial PDEA block prepared via RAFT aqueous solution polymerization (see blue data points). At this point, the CEA monomer is added, and the second-stage RAFT aqueous dispersion polymerization of CEA reaches full conversion within 8 h (total reaction time = 580 min) at 50 °C (see black data points). DLS intensity-average particle size distributions are shown in the inset for both the final nanoparticles after 580 min and also the nascent nanoparticles formed just after micellar nucleation (total reaction time = 145 min). | |
1H NMR spectra recorded for PDEA and PCEA homopolymers in CD3OD are shown in Fig. 2. PDEA (see blue spectrum) exhibits characteristic signals b, c and d at 3.4, 3.6 and 4.4 ppm respectively, plus a strong signal a representing the six pendent methyl protons at 1.5 ppm. The very weak signal at 2.73 ppm (see inset) was assigned to the thiamethylene end-group derived from the CECPA RAFT agent. This latter signal was compared to signal a to calculate a mean DP of 67 for the PDEA block via end-group analysis. PCEA homopolymer (see red spectrum) exhibits two distinctive proton signals e and f at 2.70 and 4.35 ppm assigned to the oxymethylene groups for the ester and carboxylic acid groups, respectively. As expected, all of the above PDEA and PCEA signals are observed in the 1H NMR spectrum recorded for a PDEA67–PCEA75 diblock copolymer (see green spectrum in Fig. 2).
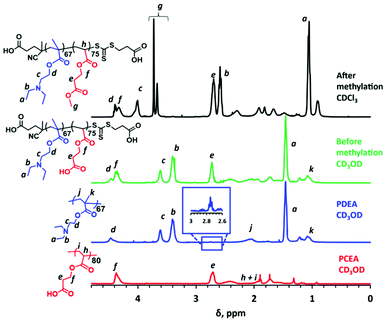 |
| Fig. 2 Representative 1H NMR spectra recorded for a PDEA67–PCEA75 diblock copolymer before (green spectrum recorded in CD3OD) and after (black spectrum recorded in CDCl3) selective methylation of its pendent carboxylic acid groups to afford the corresponding methyl esters. PDEA and PCEA homopolymer reference spectra are also shown to aid spectral assignments. | |
Selective methylation of the carboxylic acid residues in the PCEA block using TMSDAM is required prior to THF GPC analysis. 1H NMR studies of a methylated PDEA67–PCEA75 diblock copolymer (see black spectrum in Fig. 2) confirm that this derivatization is successful because a new signal g is observed at 3.72–3.66 ppm (moreover, the methylated diblock copolymer is no longer soluble in CD3OD). However, this new spectral feature clearly comprises two signals, rather than the expected single signal. This is the result of in situ transesterification of approximately 39% of the CEA repeat units to form methyl acrylate repeat units (in addition to the expected methyl ester of the CEA repeat units). This interpretation is supported by an 1H NMR spectrum recorded for a PDEA–poly(methyl acrylate) diblock copolymer reference prepared by RAFT solution polymerization of methyl acrylate in methanol using a PDEA67 precursor (see Fig. S4†). Given this unexpected side-reaction, it is perhaps worth emphasizing that there is no spectroscopic evidence for in situ hydrolysis of the ester bond in the CEA repeat units during the RAFT aqueous dispersion polymerization of CEA at pH 2.
Although excess TMSDAM is required to ensure complete methylation of the polyacid block, preliminary experiments indicated that too high an excess led to unwanted partial quaternization of the PDEA block. On the other hand, using a stoichiometric amount of TMSDAM led to an insufficient degree of methylation (<40%) of the polyacid block, with the resulting derivatized copolymer proving to be insoluble in the THF eluent used for GPC analysis. Fortunately, using a two-fold excess of TMSDAM enabled a high degree of methylation (>99%) to be achieved and such conditions did not lead to any unwanted quaternization of the PDEA block (see Fig. S5†).
According to Fig. 3, blocking efficiencies for the second-stage polymerization are reasonably high, particularly given that the chemical structure of the trithiocarbonate RAFT agent is arguably better suited for the polymerization of methacrylic monomers, rather than for acrylic monomers such as CEA. Notably, the dispersity of each diblock copolymer (Mw/Mn = 1.17–1.21) is always less than that of the PDEA67 precursor (Mw/Mn = 1.27). These results are consistent with our earlier studies, which indicate that such one-pot syntheses almost invariably offer better control over the molecular weight distribution than traditional syntheses involving isolation and purification of a homopolymer precursor (in this case, PDEA67).26,40
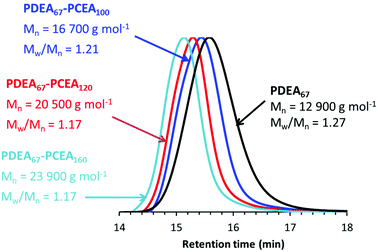 |
| Fig. 3 THF GPC curves recorded for three PDEA67–PCEAx diblock copolymers after their selective methylation using a two-fold excess of trimethylsilyldiazomethane. Comparison with the corresponding PDEA67 precursor (black curve) indicates that relatively high blocking efficiencies can be obtained using the wholly aqueous one-pot formulation outlined in Scheme 1. | |
THF GPC analysis indicate a systematic increase in Mn and relatively low dispersities (Mw/Mn ≤ 1.25) for the series of selectively methylated PDEA67–PCEAx diblock copolymers obtained when targeting PCEA DPs of 50 to 200, which suggests reasonably good RAFT control. These data are summarized in Table 1. Moreover, GPC analysis of the seven corresponding PDEA67 homopolymers (see Fig. S6†) indicated reasonably good reproducibility for the synthesis of this precursor block via RAFT aqueous solution polymerization at pH 2.
Table 1 Summary of comonomer conversions obtained by 1H NMR spectroscopy, molecular weight data from THF GPC analysis (after selective methylation of the carboxylic acid residues within the PCEA block) and mean z-average particle diameters at pH 2 and pH 10 determined by DLS and TEM for a series of PDEA67–PCEAx diblock copolymers prepared at 50 °C using a wholly aqueous one-pot protocol under the conditions summarized in Scheme 1. [N.B. The ‘n.d.’ for entry 1 denotes ‘not determined’]
Diblock copolymer composition |
1H NMR conversion (%) |
THF GPC (vs. PMMA standards) |
DLS diameter per nm (PDI) |
TEM diameter per nm ± SD |
M
n (g mol−1) |
M
w/Mn |
pH 2 |
pH 10 |
pH 2 |
pH 10 |
PDEA67–PCEA50 |
>99 |
12 800 |
1.25 |
220 (0.20) |
27 (0.07) |
n.d. |
21 ± 5 |
PDEA67–PCEA75 |
>99 |
15 600 |
1.18 |
24 (0.27) |
24 (0.03) |
27 ± 10 |
19 ± 3 |
PDEA67–PCEA100 |
>99 |
16 700 |
1.21 |
25 (0.23) |
25 (0.04) |
26 ± 4 |
18 ± 5 |
PDEA67–PCEA120 |
>99 |
20 500 |
1.17 |
39 (0.14) |
29 (0.02) |
22 ± 5 |
18 ± 3 |
PDEA67–PCEA135 |
>99 |
22 000 |
1.18 |
43 (0.03) |
30 (0.03) |
22 ± 5 |
16 ± 3 |
PDEA67–PCEA160 |
>99 |
23 900 |
1.17 |
49 (0.17) |
30 (0.03) |
24 ± 4 |
14 ± 3 |
PDEA67–PCEA200 |
>99 |
28 500 |
1.18 |
46 (0.04) |
32 (0.03) |
36 ± 3 |
13 ± 3 |
It is well known that polyampholytes such as PDMA–PMAA diblock copolymers do not normally form well-defined nanoparticles at ambient temperature, regardless of the solution pH.19,21,26,47 This is because the PDMA and PMAA blocks are not sufficiently hydrophobic in their neutral form to drive self-assembly under such conditions. In contrast, the PDEA–PCEA diblock copolymers targeted in the present study were expected to form anionic PDEA-core nanoparticles at high pH and cationic PCEA-core nanoparticles at low pH. Accordingly, we employed 1H NMR spectroscopy to seek evidence for the presence of these two types of nanoparticles (see Fig. 4). In these experiments, the pH (strictly, pD) of a 1.0% w/w solution of PDEA67–PCEA100 in D2O is adjusted as required using either NaOD or DCl. At pH 10, the ionized PCEA chains are highly anionic and are expected to act as the steric stabilizer, while the neutral PDEA block is hydrophobic and hence should form the nanoparticle cores. The corresponding 1H NMR spectrum (see blue spectrum in Fig. 4) supports this interpretation because only proton signals e, f and g assigned to the PCEA block are visible under such conditions. In contrast, the PCEA block is present in its neutral hydrophobic form at pH 2, while the pendent tertiary amine groups on the PDEA block (pKa ∼ 7.5)48 are fully protonated. Thus cationic PCEA-core nanoparticles should be formed under such conditions. In this case, 1H NMR signals a, b, c and d assigned to the PDEA block are observed (see red spectrum in Fig. 4). However, there is also an extra signal at 2.50 ppm, which is attributed to the proton signal e for the partially solvated PCEA block. This particular diblock copolymer composition exhibits an isoelectric point (IEP) at pH 3.6. Essentially no copolymer signals are detected at this solution pH, which is consistent with the observation of a macroscopic precipitate under such conditions (see green spectrum in Fig. 4).
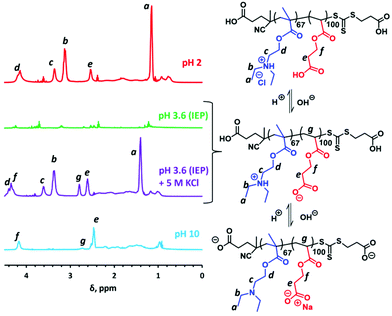 |
| Fig. 4
1H NMR spectra recorded for a 1.0% w/w dispersion of a PDEA67–PCEA100 diblock copolymer dissolved in D2O/NaOD at pH 10 (purple spectrum), in D2O at pH 3.6, which corresponds to the copolymer IEP (green spectrum), in D2O at pH 3.6 in the presence of 5 M KCl, which is added to prevent macroscopic precipitation at the IEP (purple spectrum), and in DCl/D2O at pH 2 (red spectrum). The chemical structure that is shown depicts this diblock copolymer in its neutral state; in practice, the PDEA block becomes protonated at low pH while the PCEA block becomes ionized at high pH. | |
It is well-known that the macroscopic precipitation of polyampholytes at their IEP can be suppressed by addition of sufficient salt because this screens the electrostatic attractive forces between the anionic and cationic blocks.49 Thus, addition of 5 M KCl prevents precipitation of the zwitterionic copolymer chains (see purple spectrum in Fig. 4). Under such conditions, the expected 1H NMR signals are observed for both blocks (albeit with downfield shifts).
In summary, our 1H NMR spectra are consistent with the doubly pH-responsive schizophrenic behavior expected for such PDEA–PCEA diblock copolymer nanoparticles, as shown in Scheme 2. More specifically, cationic PCEA-core nanoparticles are formed at pH 2 (i.e., during the aqueous PISA synthesis) whereas anionic PDEA-core nanoparticles are obtained on switching to pH 10.
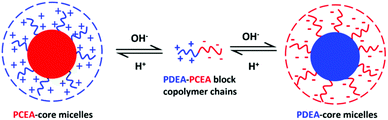 |
| Scheme 2 Schematic cartoon depicting the schizophrenic behavior exhibited by doubly pH-responsive PDEA–PCEA diblock copolymers, which form cationic PCEA-core nanoparticles at low pH and anionic PDEA-core nanoparticles at high pH. At intermediate solution pH, macroscopic precipitation occurs at (or around) the isoelectric point. | |
The RAFT aqueous dispersion polymerization of CEA results in the direct formation of PCEA-core nanoparticles at pH 2, as judged by DLS studies for the PDEA67–PCEA135 formulation (see Table 1 and Fig. 1). In this case, a well-defined spherical morphology is confirmed by TEM studies (see Fig. 5).
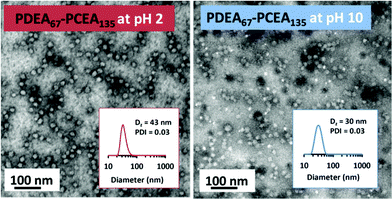 |
| Fig. 5 Representative TEM images obtained for a doubly pH-responsive schizophrenic PDEA67–PCEA135 diblock copolymer after drying from dilute aqueous dispersions adjusted to either pH 2 or pH 10, confirming the formation of cationic PCEA-core nanoparticles and anionic PDEA-core nanoparticles respectively. The corresponding DLS intensity-average particle size distributions are shown as insets within these images. | |
Digital image analysis of such TEM images enables the size of the nanoparticle cores to be estimated, with number-average diameters ranging from 22 to 36 nm (see Table 1). At pH 10, the same series of copolymers forms spherical nanoparticles with PDEA cores ranging from 13 to 21 nm. It is perhaps worth emphasizing that the best TEM images are obtained by drying nanoparticle dispersions prepared in the presence of 0.50 M KCl. The added salt screens the electrostatic repulsion from the highly charged coronal chains (i.e. cationic PDEA or anionic PCEA blocks), thus ensuring the formation of relatively compact nanoparticle cores in each case. The corresponding hydrodynamic z-average diameters recorded for these spherical nanoparticles by DLS studies at pH 2 ranged from 24 nm to 49 nm. This ‘wet’ technique is sensitive to the solvated steric stabilizer layer as well as the nanoparticle core. Moreover, it reports a z-average diameter that always exceeds the number-average diameter for a particle size distribution of finite width. Thus it is inevitable that DLS ‘oversizes’ relative to TEM.50–52 PDEA67–PCEA50 exhibited a DLS diameter of 220 nm for its PCEA-core nanoparticles at pH 2 (see Table 1), which is too large to be attributed to well-defined nanoparticles. Moreover, the scattered light intensity (or count rate) was much lower under these conditions than that determined for the same copolymer at pH 10. This is attributed to the formation of ill-defined aggregates with partially solvated cores at pH 2 owing to the relatively short PCEA block. This interpretation is consistent with the 1H NMR spectra shown in Fig. 4, which indicate that this particular polyacid block is not strongly hydrophobic.
In the case of the asymmetric PDEA67–PCEA135 diblock copolymer, TEM studies indicate that the PDEA-core nanoparticles formed at pH 10 have a number-average core diameter of approximately 16 ± 3 nm, whereas the PCEA-core nanoparticles produced at pH 2 have a number-average core diameter of approximately 22 ± 5 nm. Similarly, DLS studies indicate that the hydrodynamic z-average diameter is 43 nm for the cationic nanoparticles formed at pH 2, whereas it is only 30 nm for the anionic nanoparticles formed at pH 10. These differences are physically reasonable given that the DP of the PCEA block is almost double that of the PDEA block, which should lead to larger nanoparticles at low pH compared to those formed at high pH.
Zeta potential vs. pH curves recorded for three of the PDEA67–PCEAx diblock copolymers (where x ranges from 50 to 135) are shown in Fig. 6. In each case, the copolymer forms a macroscopic precipitate at its IEP but redissolution occurs at either higher or lower pH. The shaded area shown on each plot indicates the insolubility region. As expected, adjusting the DP of the PCEA block leads to a systematic shift in the IEP from pH 7.6 for PDEA67–PCEA50 to pH 3.6 for PDEA67–PCEA135.
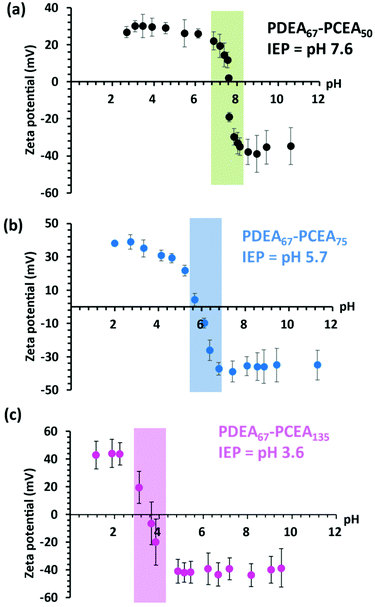 |
| Fig. 6 Zeta potential vs. pH curves obtained for three PDEA67–PCEAx zwitterionic diblock copolymers where (a) x = 50, (b) x = 75 or (c) x = 135. The shaded area shown on each plot represents the region of insolubility in each case (as judged by visual inspection). | |
Conclusions
A series of new doubly pH-responsive PDEAx–PCEAy diblock copolymers is prepared using a highly convenient, wholly aqueous one-pot formulation at pH 2 involving (i) RAFT aqueous solution polymerization of DEA followed by (ii) RAFT aqueous dispersion polymerization of CEA. After selective methylation of the PCEA block under suitably mild conditions, THF GPC studies indicate a systematic increase in copolymer Mn when targeting longer PCEA blocks using a PDEA67 precursor. Moreover, dispersities are relatively low (Mw/Mn ≤ 1.21) and there is minimal homopolymer contamination, indicating reasonably good RAFT control. The schizophrenic self-assembly behavior of such polyampholytes is examined in aqueous solution. TEM, DLS and 1H NMR spectroscopy studies confirm the formation of well-defined sterically-stabilized spherical nanoparticles comprising PCEA cores at pH 2 and PDEA cores at pH 10. Aqueous electrophoresis measurements indicate that systematic variation of the copolymer composition allows the IEP to be tuned. As expected, macroscopic precipitation occurs at around the IEP but this can be suppressed by the addition of salt. This new aqueous PISA formulation is the most convenient and efficient protocol for the synthesis of schizophrenic diblock copolymers yet reported in the literature.
Conflicts of interest
There are no conflicts to declare.
Acknowledgements
EPSRC is thanked for funding a CDT PhD studentship for the first author (EP/L016281). Lubrizol (Blackley, UK) is thanked for partial financial support of this PhD project and for permission to publish this work. S.P.A. also acknowledges EPSRC for an Established Career Particle Technology Fellowship (EP/R003009).
Notes and references
- S. Newman, J. Appl. Polym. Sci., 1962, 6, S15 CrossRef.
- S. Krause, J. Phys. Chem., 1964, 68, 1948 CrossRef CAS.
- V. Bütün, N. C. Billingham and S. P. Armes, J. Am. Chem. Soc., 1998, 120, 11818 CrossRef.
- V. Bütün, S. P. Armes, N. C. Billingham, Z. Tuzar, A. Rankin, J. Eastoe and R. K. Heenan, Macromolecules, 2001, 34, 1503 CrossRef.
- S. Y. Liu, N. C. Billingham and S. P. Armes, Angew. Chem., 2001, 113, 2390 CrossRef.
- V. Bütün, S. Liu, J. V. M. Weaver, X. Bories-Azeau, Y. Cai and S. P. Armes, React. Funct. Polym., 2006, 66, 157 CrossRef.
- M. Arotçaréna, B. Heise, S. Ishaya and A. Laschewsky, J. Am. Chem. Soc., 2002, 124, 3787 CrossRef PubMed.
- B. Y. K. Chong, T. P. T. Le, G. Moad, E. Rizzardo and S. H. Thang, Macromolecules, 1999, 32, 2071 CrossRef.
- G. Moad, Y. K. Chong, A. Postma, E. Rizzardo and S. H. Thang, Polymer, 2005, 46, 8458 CrossRef CAS.
- G. Moad, E. Rizzardo and S. H. Thang, Aust. J. Chem., 2006, 59, 669 CrossRef CAS.
- J. V. M. Weaver, S. P. Armes and V. Bütün, Chem. Commun., 2002, 2, 2122 RSC.
- C. M. Papadakis, P. Müller-Buschbaum and A. Laschewsky, Langmuir, 2019, 35, 9660 CrossRef CAS PubMed.
- N. S. Vishnevetskaya, V. Hildebrand, N. M. Nizardo, C.-H. Ko, Z. Di, A. Radulescu, L. C. Barnsley, P. Müller-Buschbaum, A. Laschewsky and C. M. Papadakis, Langmuir, 2019, 35, 6441 CrossRef CAS PubMed.
- V. Hildebrand, M. Heydenreich, A. Laschewsky, H. M. Möller, P. Müller-Buschbaum, C. M. Papadakis, D. Schanzenbach and E. Wischerhoff, Polymer, 2017, 122, 347 CrossRef CAS.
- N. S. Vishnevetskaya, V. Hildebrand, B.-J. Niebuur, I. Grillo, S. K. Fillippov, A. Laschewsky, P. Müller-Buschbaum and C. M. Papadakis, Macromolecules, 2016, 49, 6655 CrossRef CAS.
- N. S. Vishnevetskaya, V. Hildebrand, B.-J. Niebuur, I. Grillo, S. K. Fillippov, A. Laschewsky, P. Müller-Buschbaum and C. M. Papadakis, Macromolecules, 2017, 50, 3985 CrossRef CAS.
- N. S. Vishnevetskaya, V. Hildebrand, M. A. Dyakonova, B.-J. Niebuur, K. Kyriakos, K. N. Raftopoulos, Z. Di, P. Müller-Buschbaum, A. Laschewsky and C. M. Papadakis, Macromolecules, 2018, 51, 2604 CrossRef CAS.
- S. Liu and S. P. Armes, Angew. Chem., 2002, 41, 1413 CrossRef CAS.
- J. F. Gohy, S. Creutz, M. Garcia, B. Mahltig, M. Stamm and R. Jérôme, Macromolecules, 2000, 33, 6378 CrossRef CAS.
- W. Y. H. Chen, K. H. Chen, H. Chen and R. C. Ruaan, J. Chin. Inst. Chem. Eng., 2002, 33, 599 CAS.
- A. B. Lowe, N. C. Billingham and S. P. Armes, Macromolecules, 1998, 31, 5991 CrossRef CAS.
- S. Creutz, R. Jérôme, G. M. P. Kaptijn, A. W. Van Der Werf and J. M. Akkerman, J. Coat. Technol., 1998, 70, 41 CrossRef CAS.
- M. Ranka, H. Katepalli, D. Blankschtein and T. A. Hatton, Langmuir, 2017, 33, 13326 CrossRef CAS PubMed.
- A. Laschewsky, Polymers, 2014, 6, 1544 CrossRef.
- H. Liu, C. Xiong, Z. Tao, Y. Fan, X. Tang and H. Yang, RSC Adv., 2015, 5, 33083 RSC.
- S. M. North and S. P. Armes, Green Chem., 2021, 23, 1248 RSC.
- S. L. Canning, G. N. Smith and S. P. Armes, Macromolecules, 2016, 49, 1985 CrossRef CAS PubMed.
- J. Tan, H. Sun, M. Yu, B. S. Sumerlin and L. Zhang, ACS Macro Lett., 2015, 4, 1249 CrossRef CAS.
- G. Liu, Q. Qiu, W. Shen and Z. An, Macromolecules, 2011, 44, 5237 CrossRef CAS.
- L. D. Blackman, K. E. B. Doncom, M. I. Gibson and R. K. O'Reilly, Polym. Chem., 2017, 8, 2860 RSC.
- G. Mellot, J.-M. Guigner, L. Bouteiller, F. Stoffelbach and J. Rieger, Angew. Chem., 2019, 131, 3205 CrossRef.
- Z. An, Q. Shi, W. Tang, C.-K. Tsung, C. J. Hawker and G. D. Stucky, J. Am. Chem. Soc., 2007, 129, 14493 CrossRef CAS PubMed.
- P. G. Georgiou, H. L. Marton, A. Baker, T. R. Congdon, T. F. Whale and M. I. Gibson, J. Am. Chem. Soc., 2021, 143, 56 CrossRef PubMed.
- S. Sugihara, A. H. Ma'Radzi, S. Ida, S. Irie, T. Kikukawa and Y. Maeda, Polymer, 2015, 76, 17 CrossRef CAS.
- M. Inam, J. R. Jones, M. M. Pérez-Madrigal, M. C. Arno, A. P. Dove and R. K. O'Reilly, ACS Cent. Sci., 2018, 4, 63 CrossRef CAS PubMed.
- L. D. Blackman, S. Varlas, M. C. Arno, A. Fayter, M. I. Gibson and R. K. O'Reilly, ACS Macro Lett., 2017, 6, 1263 CrossRef CAS PubMed.
- G. Liu, Q. Qiu and Z. An, Polym. Chem., 2012, 3, 504 RSC.
- S. L. Canning, T. J. Neal and S. P. Armes, Macromolecules, 2017, 50, 6108 CrossRef CAS PubMed.
- V. Bütün, M. Vamvakaki, N. C. Billingham and S. P. Armes, Polymer, 2000, 41, 3173 CrossRef.
- S. J. Byard, C. T. O'Brien, M. J. Derry, M. Williams, O. O. Mykhaylyk, A. Blanazs and S. P. Armes, Chem. Sci., 2020, 11, 396 RSC.
- F. L. Hatton, A. M. Park, Y. Zhang, G. D. Fuchs, C. K. Ober and S. P. Armes, Polym. Chem., 2019, 10, 194 RSC.
- N. J. Warren and S. P. Armes, J. Am. Chem. Soc., 2014, 136, 10174 CrossRef CAS PubMed.
- S. M. North and S. P. Armes, Polym. Chem., 2021, 12, 4846 RSC.
- D. J. Keddie, Chem. Soc. Rev., 2014, 43, 496 RSC.
- Y. Zhang, L. Lie, X. Dai, L. Zhang and J. Tan, ACS Macro Lett., 2019, 8, 1102 CrossRef CAS.
- L. Yu, X. Dai, Y. Zhang, Z. Zeng, L. Zhang and J. Tan, Macromolecules, 2019, 52, 7267 CrossRef CAS.
- Y. Xia, X. Xu, H. Yu, C. Zhou, Z. Nie, J. Yang, J. Qian and H. Ni, Colloid Polym. Sci., 2021, 299, 663 CrossRef CAS.
- V. Bütün, S. P. Armes and N. C. Billingham, Polymer, 2001, 42, 5993 CrossRef.
- I. V. Portnov and I. I. Potemkin, J. Phys. Chem. B, 2020, 124, 914 CrossRef CAS PubMed.
- L. N. Pilon, S. P. Armes, P. Findlay and S. P. Rannard, Langmuir, 2005, 21, 3808 CrossRef CAS PubMed.
- J. Du and S. P. Armes, Langmuir, 2008, 24, 13710 CrossRef CAS PubMed.
- J. Madsen, S. P. Armes, K. Bertal, H. Lomas, S. MacNeil and A. L. Lewis, Biomacromolecules, 2008, 9, 2265 CrossRef CAS PubMed.
Footnote |
† Electronic supplementary information (ESI) available: Digital photographs of PCEA homopolymer in water at pH 10 and pH 2 to demonstrate its insolubility at low pH; digital photographs of the visual appearance of the reaction mixture; semi-logarithmic kinetic data for the RAFT aqueous dispersion polymerization of CEA targeting PDEA67-PCEA135; 1H NMR spectra for a methylated PDEA-PCEA diblock copolymer and also a PDEA-poly(methyl acrylate) diblock copolymer; 1H NMR spectra illustrating unwanted quaternization of the PDEA block when using a large excess of TMSDAM. See DOI: 10.1039/d1py01114f |
|
This journal is © The Royal Society of Chemistry 2021 |