DOI:
10.1039/D1NR01401C
(Minireview)
Nanoscale, 2021,
13, 9147-9159
Extending photocatalysis to the visible and NIR: the molecular strategy
Received
3rd March 2021
, Accepted 22nd April 2021
First published on 10th May 2021
Abstract
Photocatalysis exploits light to perform important processes as solar fuel production by water splitting, and CO2 reduction or water and air decontamination. Therefore, photocatalysis contributes to the satisfaction of the increasing needs for clean energy, environmental remediation and, most recently, sanification. Most of the efficient semiconductor nanoparticles (NP), developed as photocatalysts, work in the ultraviolet (UV) spectral region and they are not able to exploit either visible (Vis) or near infrared (NIR) radiation. This limitation makes them unable to fully exploit the broad band solar radiaton or to be applied in indoor conditions. Recently, different approaches have been developed to extend the spectral activity of semiconductor NP, like for example band-gap engineering, integration with upconversion NP and plasmonic enhancement involving also hot-electron injection. Nevertheless, the use of organic molecules and metal complexes, for enhancing the photoactivity in the Vis and NIR, was one of the first strategies proposed for sensitization and it is still one of the most efficient. In this minireview we highlight and critically discuss the most recent and relevant achievements in the field of photocatalysis obtained by exploiting dye sensitization either via dynamic or static quenching.
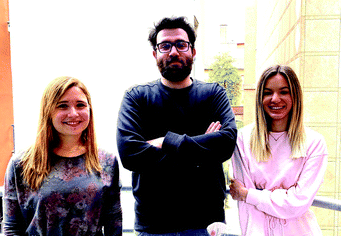 Alexandra Mavridi-Printezi (right), Arianna Menichetti (left) and Moreno Guernelli (center) | Alexandra Mavridi-Printezi (right), Arianna Menichetti (left) and Moreno Guernelli (center) are PhD students in the group of Prof. Marco Montalti. Their main research activity is the development of organic and inorganic photoactive supramolecular and/or nanostructured platforms for energy conversion, environmental remediation and ROS photogeneration. The materials on which their research projects are focused include metal–oxide and metal nanoparticles, graphene and related materials, organic chromophores and melanin-like NP. |
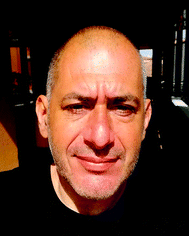 Marco Montalti | Marco Montalti received his PhD in Chemical Sciences in 2001 and he is presently Professor of Chemistry at the University of Bologna. In 2002 he started his independent research career in the field of luminescent silica and metal nanoparticles for sensing and bioimaging. The main research topics of his group are the design, production, and characterization of Vis-NIR photoactive and/or stimuli responsive supramolecular and nanostructured systems and nanocomposites. Applications of these materials include photocatalysis for energy conversion and environmental remediation, bioimaging and theranostic. |
1 Introduction
Photocatalytic processes exploit light, typically sunlight, to achieve chemical reactions of enormous importance for the human community.1 These processes can be utilized e.g. for storing solar energy in the form of clean and abundant fuel, H2, starting from water2–4 or to convert potentially hazardous pollutants such as CO2 into useful compounds.5–7 In organic synthesis light-driven reactions have much weaker impact on the environment than traditional thermal processes and they can allow a better control on the selectivity.8–12 Finally, photocatalysis can be exploited for the removal of chemical and biological contaminants from water and air both in outdoor and indoor conditions.13–15
Photocatalysis is a multistep process where a photoactive system, the photocatalyst (PC), completes, in the simplest case, three sequential processes: (i) photon absorption, (ii) charge separation to produce hole and electrons and (iii) redox reaction with reactants. The overall efficiency is hence, the product of the efficiencies of the three processes. To be more precise, a photocatalytic reaction is a thermodynamically downhill process, while the thermodynamically uphill process with ΔG > 0, like solar fuel production, is a photosynthetic reaction.16 Nevertheless, in most cases this distinction is not considered in the scientific literature. The most important photocatalytic processes and in particular solar fuel production, are not only strongly energetically demanding (ΔG ≫ 0), but also they require additional energy to overcome the high kinetic energy barriers (over-potentials), even in the presence of co-catalysts. Additionally, as shown in Fig. 1, before the actual available reactive electrons and holes species are formed, part of the energy of the initially absorbed photon is dissipated through relaxation processes. As a consequence, when simple schemes as the one in Fig. 1 are considered, high energy UV photons are typically needed. For example, photocatalytic water splitting with a 96% quantum efficiency was recently reported by Domen and co-workers using aluminium-doped strontium titanate PC under UV light irradiation.17
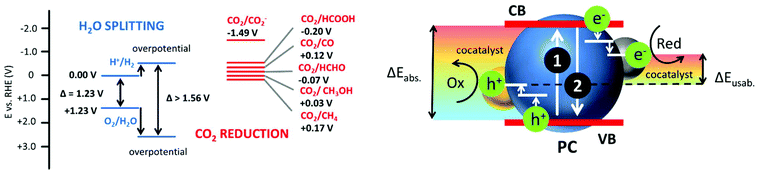 |
| Fig. 1 Left. Simplified energy diagrams for water splitting and CO2 photoreduction. The actual energy need ΔG for water splitting is only 1.23 V that corresponds to just about 1000 nm and hence to NIR region photons. Nevertheless, the overpotential for this multistep process is considerable and higher energy is needed even in the case of the use of cocatalyst for reduction and oxidation. Additionally, as shown on the right, photon energy is absorbed by the PC (ΔEads.) transferring an electron from the valence band (VB) to the conduction band (CB), therefore producing the electron–hole pair (process 1). Nevertheless, even in the case of poor charge recombination (process 2), several processes occur before the separated and trapped electron are available for the reaction (including charge transfer to the cocatalysts). This reduces the amount of energy actually available for redox (ΔEusab.). | |
Indeed, depending on the application, different spectral signatures are required. In particular, broad spectrum activity, ranging from the UV to the NIR18,19 is needed in order to achieve highly efficient exploitation of the whole solar energy spectrum for efficient solar fuel production and environmental remediation. Good efficiency upon Vis light absorption is necessary for most indoor applications involving the use of artificial light sources. PC for organic synthesis need to be responsive to powerful, safe and easily available light source typically in Vis-NIR range.20
In general, the strategies to enhance the response of PC in the Vis and NIR regions can be, to some extent, grouped in few general categories.2 Among others, band-gap engineering, integration with up-conversion NP and plasmonic enhancement21 involving also hot-electron injection,22 are included. The use of molecular photosensitizers (PS), as those shown in Fig. 2, was proposed for extending the spectral response of photocatalytic systems since four decades, nevertheless this approach is still one of the most efficient.23 The most recent and promising approaches for molecular based sensitization include the incorporation of the PS in the PC nanostructure, especially in the case of Metal Organic Framework (MOF)24 or Covalent Organic Framework (COF),25 or the exploitation of multiple sequential photon absorption.26 Depending on the strategy, the PS can be either (i) integrated in the PC, (ii) bound covalently or non-covalently to PC or can be (iii) free in solution. In the first two cases, during the excitation the PS is already “interacting” with the PC and the excited PS is quenched by the PC “statically”, typically via electron transfer.27 In the third case, the free PS is excited and then it diffuses toward the PC creating some delay between excitation and sensitization through the “dynamic” process. As a drawback, relatively long excited state lifetimes are requested for the dynamic sensitization. On the other hand, back-diffusion after electron injection can lead to kinetic stabilization of the charge separated pair, while in the case of static processes, fast charge recombination typically occurs, unless charge migration and stabilization takes place (also involving cocatalysts).6
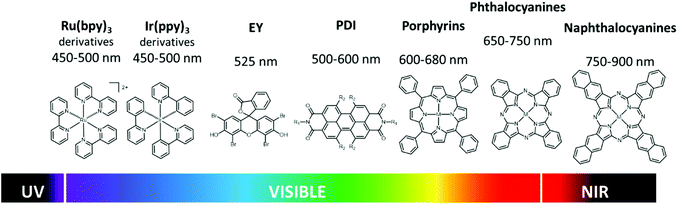 |
| Fig. 2 Examples of PS molecules covering the Vis-NIR absorption window. The optical properties can be finely tuned by changing the substituents or the central metal (in the case of porphyrins, phthalocyanines and naphthalocyanines. The choice of the substituents affects also the solubility, aggregation properties, and the interaction with the different photocatalytic materials. | |
In addition, as shown in Fig. 3, sensitization occurs, in the simplest case, via electron injection from the excited PS to the PC VB producing the oxidized PS. Often sacrificial agents (e.g. triethanol amine, TEOA) are used to restore the PS but this actually alters the energetic balance of the overall photocatalytic process. As a result, for a better exploitation of the energy of Vis-NIR photons, more sophisticated photocatalytic schemes involving multiple photon (sequential) absorption, as the Z-scheme shown in Fig. 3, are necessary.
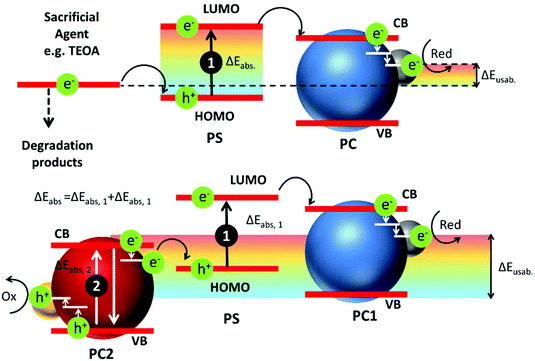 |
| Fig. 3 Top. A molecular PS can absorb photon of lower energy with respect to the PC and then inject an electron into the CB of the PC that then can perform reduction. Differently from the direct excitation of the PC sensitization does not produce h+ in the PC but in the oxidized PS (PS+). If a sacrificial agent is used for reducing the PS, its degradation has to be considered in the energetic balance, reducing the actual amount of usable energy. Bottom. An effective way to achieve more energetic processes is the combination of the energy of two photon absorbed by the PS (process 1) and by a second PC (PC2, process 2) in a Z-scheme. | |
Another parameter that has to be considered in photocatalysis is oxygen, that is typically present in the reaction environment, and which can quench the PS or be involved in alternative photocatalytic cycles.28
Going back to spectral sensitivity, as shown in Fig. 2, the optical properties of the molecular PS are strongly affected by the presence of substituents. Interestingly the effect of substitution can be, to a large extent predicted, by computational chemistry.29,30 Recently it was, for example, demonstrated as a slight difference in the structure, as the one between chlorophyll and bacterochlorophyll, results in substantial shift of the absorption energy.31,32
Even though one of the main challenges in photocatalysis is the development of materials that exploit light energy more and more efficiently, it is still difficult to univocally quantify this efficiency and its dependency on the irradiation wavelength.33
Considering for example the definition of quantum efficiency or quantum yield of a photoreaction34 it may appear surprising that a PC can, in principle, absorb light poorly at a given wavelength showing a high quantum efficiency anyway. For this reason, new definitions have been introduced to consider the effect of the wavelength as the apparent quantum yield or, specifically for hydrogen production, the solar-to-hydrogen efficiency (STH).2 Nevertheless, the actual comparison of results from different research groups is still in many cases very difficult, especially when multiple photon absorption is involved, where the efficiency of the process becomes dependent on the irradiation intensity and the spectral distribution.
In this minireview we will focus on the role of molecular PS in photocatalysis as a strategy able to extend the spectral activity of PC in the Vis and NIR region. We would like to underline that, also considering the vastity of this topic, in this work we do not aim to give a comprehensive overview of the scientific production in the field, but rather to present briefly a selection of the most recent and interesting developments, focussing on different materials used either in heterogeneous or homogeneous photocatalysis.
2 Heterogeneous photocatalysis
Several different semiconductors have been proposed for heterogeneous photocatalysis. A large number of them is very effective upon UV exposure, but they show poor photocatalytic efficiency upon irradiation in the Vis or in the NIR.
2.1 Metal oxide NP
Most metal oxide semiconductor NP, and in particular TiO2, are efficient photocatalysts under UV irradiation, but because of their broad electronic band gap they do not absorb Vis light.2 Structure modification by hydrogenation and reduction treatments, to produce “black” TiO2, contributed only in part to the increase of the Vis-driven photocatalytic activity.35 In fact, this activity of inorganic semiconductor NP in the Vis-NIR can be enhanced by conjugating them either to up-conversion nanoparticles (UCNP) or to plasmonic metal structures.36–38 Sensitization with inorganic quantum dots and carbon or graphene dots has been also reported.39 Moreover, the successful application of metal oxide as PC for solar light based H2 photogeneration,2,40 CO2 photoreduction, environmental remediation and organic synthesis have been described bibliographically.41,42 It must be noted that the sensitization of TiO2 by ruthenium(II) trisdiimine complexes (in particular [Ru(bpy)3]2+, bpy = 2,2′-bipyridine) in order to achieve photocatalytic H2 production under Vis light was already reported in 1981.23 More recently, various dyes, including diketopyrrolopyrrole,43 phenothiazine derivatives,44 phenoxazine and carbazole derivatives,45 as well as perylene monoimide,46 have been immobilized on semiconductors.
An interesting alternative to conventional photosensitization was recently proposed by Wurthner and Xie (Fig. 4), who developed a TiO2 modified PC47 for hydrogen evolution based on a sequential dual photon absorption according to a scheme previously proposed by König and coworkers.48,49 The PC was obtained by grafting to TiO2 NP a perylene bisimide (PBI) dye able to absorb green light, forming a stable radical anion. The previously formed species could absorb in a second step NIR giving finally, a stable PBI dianion. In a further stage, the dianion absorbed red light photons and, once excited, injected an electron into the TiO2 NP leading to hydrogen evolution at a rate as high as 1216 μmol h−1 g−1. Being still under discussion,50 this mechanism has been recently investigated by ultrafast transient spectroscopy.51
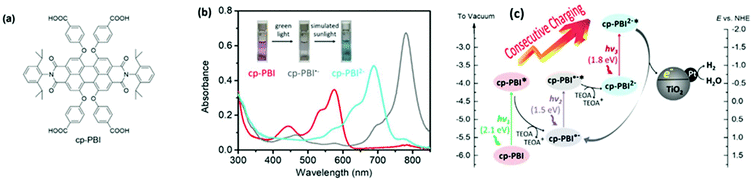 |
| Fig. 4 (a) Illustration of the chemical structure of PBI dye. (b) UV/Vis absorption spectra of PBI in the absence of oxygen and in the presence of sacrificial thioethanolamine in the dark, and of its two anion forms produced by light irradiation in the presence of the same sacrificial agent. Inset in (b) exhibits the images of the solutions in cells. (c) Schematic representation of the hydrogen evolution including the charging processes of PBI through multistep photo-induced electron transfer and the upcoming charge transfer from the PBI dianion to TiO2 NP. The positions of the energy levels for both radical anion and the dianion are estimated based on the cyclic voltammetry results. Normal hydrogen electrode is symbolized as NHE. This figure has been reproduced from ref. 47 with permission from John Wiley and Sons, copyright 2020. | |
A different PBI was also proposed by Bonchio and Prato as a PS for achieving the most critical step of water splitting, the water photo-oxidation to produce oxygen.52 More in detail, a photo-anode, that was able to mimic the natural photosystem II, was constructed by the functionalization of nano-WO3 with a hierarchically organized PBI/polyoxometalates (POM)53 system. In this system the formation of large two-dimensional paracrystalline domains was observed and a light-harvesting efficiency higher than 40%, with a peak quantum efficiency under green light irradiation (λ > 500 nm), was demonstrated.
In another interesting example, Huang and Liu adopted also a supramolecular approach in order to expand the photocatalytic efficiency of TiO2 in the Vis region. In this case, TiO2 NP, after being functionalized with a new calix[4]arene dye, were loaded with a rhenium complex Re(bpy)(CO)3Cl for CO2 reduction to CO (Fig. 5).54
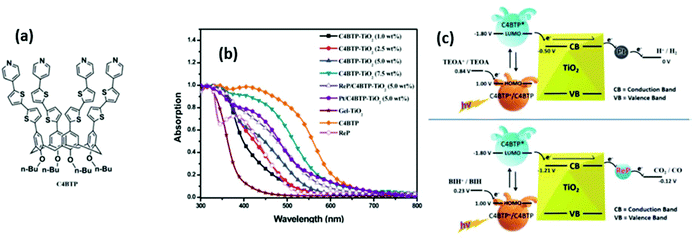 |
| Fig. 5 (a) Chemical structure of calix[4]arene-based dye. (b) UV-Vis absorption spectra of calix[4]arene dye, gel-TiO2, and dye-TiO2 with different content of the first (c) Illustration of the band structure and total mechanism of the system for (top) H2 evolution in water solution and (bottom) CO2 reduction of calix[4]arene -TiO2 in DMF solution. All the potential noted are against normal hydrogen electrode (NHE). This figure has been reproduced from ref. 54 with permission from Royal Society of Chemistry, copyright 2020. | |
Targeting the same photocatalytic process Meyer and co-workers functionalized NiO NP, using a Zr4+ based layer-by-layer procedure and a phosphonate derivative of [Ru(bpy)3]2+, in order to produce a donor-chromophore-catalyst assembly for the photoreduction of CO2 to CO.55
Inorganic semiconductor nanomaterials offer as major advantages high chemical and photochemical stability and a relatively high efficiency of the charge separation process with the formation of long-lived reactive species suitable for solar fuel production. Nevertheless, it is worth considering that in the case of photosensitization the stability of the PS itself may become a major concern and the long term efficiency of hybrid organic PS/inorganic semiconductor in the Vis-NIR region has not been clearly explored yet.
2.2 Perovskites
The use of metal halide perovskite (MHP) in photocatalysis is limited56 mostly because of their poor stability and of the fast electron–hole recombination.57,58 Although several strategies have been proposed to overcome these obstacles2,56,59,60 it is worth noticing that even in the case of perovskite-based PC the use of a molecular PS can allow their easy integration in a Z-scheme by coupling two photocatalytic processes, as recently demonstrate for overall water splitting (Fig. 6).61
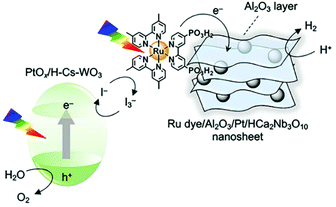 |
| Fig. 6 Schematic illustration of Z-scheme splitting using Ru(II) tris-diimine dye for sensitization of Al2O3/Pt/HCa2Nb3O10 nanosheets in combination with PtOx/H–Cs–WO3. This figure has been reproduced from ref. 61 with permission from American Chemical Society, copyright 2020. | |
2.3 2D Materials
2D materials like graphene,62,63 TMDs,64,65 MXenes66 and black phosphorus absorb efficiently Vis/NIR light and they have been extensively used for Vis-NIR photocatalysis.15 However, these materials are either conductors or they show fast recombination of the photo-generated electron–hole pair, therefore they found application in photocalatysis mostly as cocatalysts.6,41
Recently, graphitic carbon nitride (g-CN) on the contrary, has found wide application as PC.67
Graphene.
Recent attempts to open the band gap of graphene in order to exploit it as PC, include nitrogen doping and production of graphene quantum.68 In this last case, sensitization with rhodamine was utilized for H2 generation.69
MXene.
Thanks to its 2D structure and electronic conductivity, MXene was recently exploited as an electronic acceptor able to favor the charge separation in semiconductors, recalling the role of graphene.70,71 In particular, MXenes were used as co-catalysts for Vis-NIR based H2 production in combination with COFs,72 CdS nanorods73 or Pt NP.74 On the other hand, sensitization with molecular PS has been rarely documented. A recently reported exception was the use of Eosin Y for the sensitization of a TiO2/Ti3C2 composite on amorphous carbon.75
Black phosphorous.
Differently from other phosphorous-based materials,76–78 black phosphorous (BP) is a 2D material with a bandgap (0.3–1.5 eV) dependent on the number of layers.79 However, even if BP absorbs Vis/NIR, its photocatalytic efficiency is still low and its quantum efficiency has not been extensively studied yet.80,81 BP is almost always associated with other materials in order to be competitive for photocatalytic applications.82,83 For example H2 production under Vis/NIR irradiation was achieved by 2D/2D heterojunction of BP and carbon nitride (BP/g-CN)84 and either by combination with CdS NP85 or with MoS2-BP/GO.86
Similarly to MXene, BP was rarely sensitized with molecular PS. A recent example was presented by Xue et al., who suggested an Erythrosin B-sensitized system, using BP/Pt as a co-catalyst for photocatalytic H2 production under Vis light irradiation.87
Graphitic carbon nitride (g-CN).
This organic semiconductor exhibits Vis light response, because of its narrow and tunable band gap and an excellent chemical stability.67 Among the strategies proposed, in order to increase the small surface, reduce the high electron–hole recombination and tune the optical properties of this material, are included non-metal doping, thermal exfoliation, carbon-dots functionalization88,89 and more recently incorporation into heterojunction.67 All these strategies aimed to the production of PC suitable for solar water splitting,90 CO2 reduction,38,91,92 environmental remediation18,93 and organic synthesis.94–96
In the past, several light-harvesting units have been exploited to extend the spectral activity of g–CN, such as Eosin Y,97 phthalocyanine,98–100 and porphyrin.101–104
Da Silva and co-workers sensitized g-CN by means of free-based porphyrins to promote H2 production under UV-Vis irradiation.105 Moreover, several metalloporphyrins can be used as PS in carbon nitride for H2 production.106 However, porphyrin often has a double role in the enhancement of g-CN photocatalytic activity, behaving both as PS and co-catalyst by inhibiting charge recombination.107–111 Resiner, in particular developed a system where a polymeric cobalt phthalocyanine was used to functionalize g-CN working purely as a co-catalyst for CO2 reduction.112
g-CN sensitization by phthalocyanine was applied also for H2 production. For this purpose, Zeng et al. exploited a zinc phthalocyanine113 and Yi et al. a copper phthalocyanine.114
Moving toward different examples, Yuan et al. enhanced the photocatalytic water oxidation under Vis light by using 3,4,9,10-perylenetetracarboxylic acid anhydride as a sensitizer.115 In another interesting example, Chlorine e6 was used as a NIR biosafe PS.116 The resulting system had absorbance within 200–1400 nm and could be suitable in energetic, environmental and biological applications.
In a late work, oxidized g-CN rich in hydroxyl and carboxyl functional groups was selected as a support, able to bind with multiporphyrin arrays via a layer-by-layer assembling to achieve a nanocomposite, that eventually exhibited dramatic photocatalytic Vis-light degradation of Rhodamine B and phenol.108 Although g-CN is surely a promising material for Vis-NIR based photocatalysis both for its intrinsic low band gap, chemical composition, simplicity of preparation and low cost, it still has not been demonstrated to be competitive with respect to inorganic semiconductor in term of charge separation efficiency and long term stability.
2.4 Metal organic frameworks (MOF)
Metal–organic frameworks (MOF), also known as porous coordination polymers, have been applied effectively for a variety of Vis light-activated processes like H2 production,117,118 CO2 photo-reduction,119–121 photo-degradation of dyes and pollutants,122,123 disinfection of bacteria124 and various organic reactions.125,126 Advantages of MOF include the presence of pores for selective introduction of substrates,24 easy modification for single atom catalysis127 and bandgap tunability with plasmonic NP.128 On the other hand, main concerns are the moderate water stability of MOF129 and the low mobility of photogenerated electrons and holes.130 Unfortunately, numerous MOF exhibit optical absorption only in the UV region.24 A simple and effective molecular strategy in order to overcome the aforementioned obstacles, and therefore tune the optical properties, is based on the choice of the ligands, which can act as an ‘incorporated’ light-absorbing antenna exploiting ligand to metal charge transfer (LMCT) processes.124,131,132 Another possibility is the use of an ‘external’ photosensitizers (PS) either via dynamic or static quenching.4
An interesting example of the first strategy was recently proposed by Cadiau et al. who exploited a titanium MOF containing a pyrene derivative as Vis light-responsive photocatalyst for a range of reactions including hydrogen evolution (Fig. 7a).133 Isaka et al. developed a set of titanium based MOF with different organic ligands able to absorb light up to 500 nm and they demonstrated their applicability for the photocatalytic synthesis of hydrogen peroxide in a two phase system.134
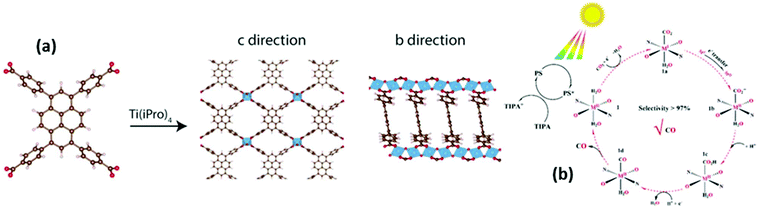 |
| Fig. 7 (a) Schematic illustration of the one-step synthesis of the titanium-based MOF from the 4,4′,4′′,4′′′-(pyrene-1,3,6,8-tetrayl)tetrabenzoic acid ligand. In the c direction there are views of the stimulated structure emphasizing on the microporous channels, while in the b direction the views of the Ti–O–Ti chains along the b axis are presented. Ti is illustrated with blue colour, C with dark brown and O with red. H atoms are excluded. (a) This figure has been reproduced from ref. 133 with permission from John Wiley and Sons, copyright 2020. (b) Proposed photocatalytic mechanism of MOFs in the presence of PS for the CO2 photoreduction to CO. (b) This figure has been reproduced from ref. 135 with permission from American Chemical society, copyright 2019. | |
Dynamic sensitization was proposed by Wang et al. in the case of three isostructural MOF, namely MOF-Ni, MOF-Co, and MOF-Cu, which were used in combination with the PS [Ru(bpy)3]2+ for enhanced Vis absorption. In particular, due to the matched LUMO positions between the two components, the photogenerated electrons in the LUMO of the PS could migrate to the surface of the MOF-based catalyst, eventually leading to effective CO2 reduction with selectivity up to 97.7% in the case of MOF-Ni (Fig. 7b).135 Similarly Xu et al. suggested a NH2-MIL-101(Fe) MOF-derived hybrid hollow metal phosphide with high photocatalytic activity and with a H2 evolution rate up to 13.81 mmol g−1 h−1 upon Eosin Y sensitization.136 A recent case of static photosensitization was reported for Rhodamine B, which was exploited as a PS, able to improve the photocatalytic capacity of MIL-125(Ti). The dye could be strongly anchored on the surface of the MOF leading to 90% efficient MO degradation under Vis light via hole and superoxide free radical generation.136 Similarly, Li et al. encapsulated different dyes (Basic Yellow 24, Basic Red 14 and Methylene Blue) to Cu-MOF, with significantly lowered energy band gap values and enhanced photodegradation ability up to 97.3%.137
Recently porphyrinic Zirconium MOF have also be demonstrated to be efficient visible light activated PC for PET-RAFT polymerization.138 MOF are very versatile structures in which molecular PS can be easily integrated to extend the spectral responsivity to the Vis-NIR window. Although, the chemical stability of these materials is still a major concern together with the poor charge mobility and electron/hole separation efficiency.
2.5 Covalent organic framework (COF) and conjugated organic polymers (COP)
Covalent organic frameworks (COF) are polymers with tunable porosity, π-conjugated structure, and good chemical stability used for Vis light-based water splitting, CO2 photo-reduction, dye degradation, and organic synthesis.16,25,139–143
Even though COF are semiconductor materials, their design is based on a molecular approach, and the photophysical and photochemical properties strongly depend on the choice of the incorporated molecular building blocks: triazine, pyrene, porphyrin, thiophene, or sulfone, etc.144 An interesting strategy, able to promote efficiency of photo-generated electron–hole pairs, avoid recombination of charge, and improve light harvesting ability, was achieved by Li et al. by the introduction of electron D–A (donor–acceptor) molecular moieties in COF for sunlight-driven H2 evolution (Fig. 8).145
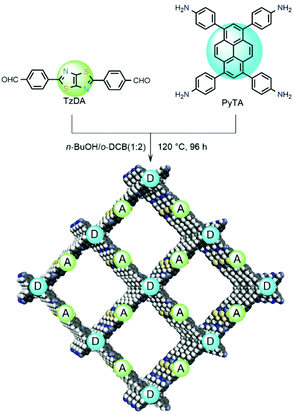 |
| Fig. 8 Donor–Acceptor (D–A) MOF system synthesized under solvothermal condition. This figure has been reproduced from ref. 145 with permission from John Wiley and Sons, copyright 2021. | |
In a different approach a molecular PS, [Ru(bpy)3]2+, was incorporated in the pores of a new water-soluble 3D COF together with POM to achieve H2 photoproduction.146
Integration of a Re(bpy)(CO)3Cl homologous in the structure of a crystalline COF was exploited by Fu et al. for highly specific Vis photo-conversion of CO2 into fuels.147 In another case, in order to extent the spectral activity of terpyridyl-based, 2D lamellar COF-909 nanorods coordination to copper was applied by Dong et al.148 The added metal centers enhanced also the electrons-holes separation providing specific binding sites, able to improve the recognition of target organic toxicants.
An interesting synthetic approach of COF was achieved by Yu et al. by polymerizing an Eosin Y precursor (Fig. 9) in a conjugated structure that exhibited high activity for the photocatalytic reduction of CO2 to CO in the presence of gaseous H2O.149 Importantly, the production rate was up to 33 μmol g−1 h−1 and the selectivity as high as 92%. A rational design of COF, reported by Kang et al., allowed to combine programmed spectral sensitivity to photocatalytic selectivity in organic synthesis. These authors were the first who showed an example of Vis light-driven asymmetric photocatalysis.150 As for other polymeric materials, because of the lack of a crystalline structure, photo-induced charge separation and migration is poorly efficient in COF and COP. Nevertheless, this drawback is partially compensated by the high versatility of design that allows the simple tuning of the optical properties by incorporating specific molecular absorbers.
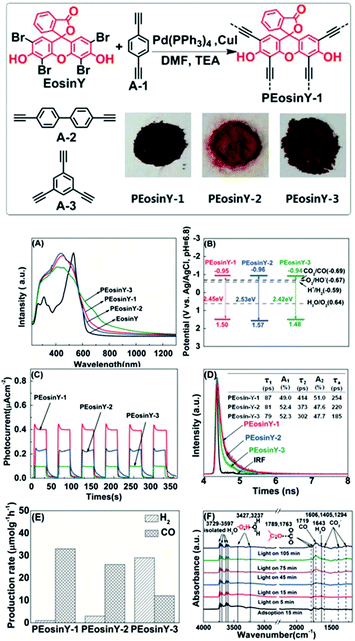 |
| Fig. 9 (Top) Synthetic pathway for the production of the conjugated Eosin Y-functionalized COFs. (A) UV-Vis absorption spectra of Eosin Y and of the Eosin Y functionalized polymers, PEosin Y–N, where N = 1–3 (B) Schematic illustration of CB and VB of PEosin Y–N (C) Transient photocurrent responses of PEosin Y–N at λ > 420 nm (D) time-resolved fluorescent decay spectra (TRFDS) of PEosin Y–N at 640 nm upon irradiation at 405 nm (E) average production rates of CO from CO2 photoreduction in the presence of PEosin Y–N for 50 h at λ > 420 nm (F) reflectance infrared Fourier transform spectra (DRIFT) of PEosin Y–N for CO2 and H2O absorption and their photoreactions under Vis light irradiation. The bands with significant changes are represented with full lines referring to the decreasing or gradually disappearing bands, and with dashed lines referring to the newly appearing or increasing bands. This figure has been reproduced from ref. 149 with permission from John Wiley and Sons, copyright 2019. | |
3 Homogeneous photocatalysis
In homogeneous photocatalysis photochemical reactions are typically initiated by the absorption of light by a molecular PS in the reaction solvent.9
The excited PS is hence involved either in single electron transfer (SET), in the case of photoredox catalysis, or triplet energy transfer (EnT) via Dexter mechanism schematized in Fig. 10.8,10,151 Recently, special attention have been devoted to a more general understanding of the role of singlet and triplet excited states in photoredox catalysis.152 Advantages of using Vis-NIR light for photoexcitation include: (i) use of light sources safer than UV, (ii) reduced photodamage of the PS, (iii) increased selectivity and (iv) for NIR, the possibility of using non-transparent reaction vessels. The main strategies to exploit the Vis-NIR region in homogenous photocatalysis are two and namely: (i) development of new PS and (ii) multiphoton (sequential) absorption.26 Regarding the former strategy, the efficiency in specific spectral regions depends on the optical properties of the PS itself. Typically used PS, like [Ru(bpy)3]2+ and [Ir(ppy)3]+, absorb only partially in the Vis region (λ < 500 nm) possessing a moderate molar absorption coefficient (ε ∼ 103–104 M−1 cm−1). The search for new PS with enhanced absorption in the Vis-NIR is hence very active. Castellano for example recently proposed a new cyclometalated Ir(III) diimine PS for homogeneous photocatalytic hydrogen production under Vis light.153
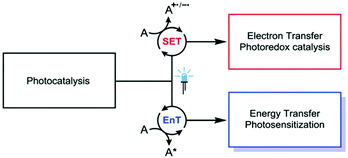 |
| Fig. 10 Simplified schematic illustration of the concept of photocatalysis. This figure has been reproduced from ref. 10 with permission from Royal Society of Chemistry, copyright 2018. | |
At the same time the number and variety of chemical processes that can be achieved with Vis-NIR active PS is impressively increasing. Recent examples of visible light driven photocatalysis include, for example, direct isotopic carboxylate exchange of C(sp3) acids with labeled CO2.154 Strong bis-cyclometalated iridium PS with electron-rich b-diketiminate ancillary ligands were demonstrated to yield effcient photoredox transformations of unactivated substrates using blue or green visible-light.155
Synthesis of unnatural α-amino acids via stereoselective C-radical addition to a chiral glyoxylate-derived N-sulfinyl imine was achieved by visible light-promoted photoredox catalysis using either metal-bases and metal-free PS.156
A novel strategy was also proposed where CO2 released by decarboxylation of amino acids (a typical waste byproduct) was used for photoredox-neutral carbocarboxylation of alkenes.157
In general, large scale application of photocatalysis needs largely available, inexpensive PS that do not contain precious metal.
For this reason Chen et al. developed a new strong Vis-light-absorbing copper-based PS with an ε as high as 160
000 M−1 cm−1 at 518 nm.158 By adopting a through-bond energy transfer from boron dipyrromethene (Bodipy) the excitation efficiency of Cu(I) complex was 62 times enhanced. In another important development, Zhang et al. proposed a zirconium(IV) PS with ligand-to-metal charge-transfer excited states and demonstrated its applicability for photoredox catalysis. This Zr complex showed a broad absorption band, centered at 525 nm, and an exceptionally long-lived triplet LMCT excited state (τ = 350 μs), featuring highly efficient photoluminescence emission (Φ = 0.45) and a peculiar delayed fluorescence.159
Regarding the development of new PS, it is worth underlining that absorption of low energy photons produces excited state with poor energy content, hardly exploitable for most valuable chemical reactions. Consequently, the combination of photons of low energy to produce high energy species, in new photocatalytic scheme is surely a promising strategy.26 In this context, some years ago the reduction of aryl halides by consecutive Vis light-induced electron transfer processes was reported.48 The photocatalytic mechanism was investigated more in detail by the group of Schanze.51
In alternative, the sequential absorption of two low energy photons has been recently proposed for example by Ravetz et al. who demonstrated that molecules absorbing infrared light can be used for photoredox catalysis exploiting triplet fusion upconversion (Fig. 11); a process that transforms two low energy poorly reactive triplet excited states into a high energy reactive one. The resulting system containing a PS and an annihilator is able to accomplish a wide range of photoredox reactions under NIR light illumination.160 Similarly, Mahammed et al. reported triplet–triplet annihilation upconversion for phosphorus corrole complexes.161 Recently, Nicewicz's group demonstrated the formation, upon sequential multiple photon absorption, of a neutral acridine radical with a maximum excited-state oxidation potential similar to elemental lithium.162
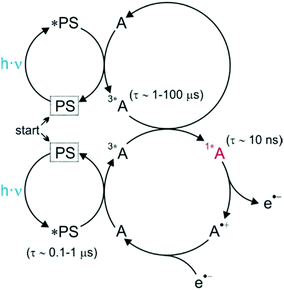 |
| Fig. 11 Schematic illustration of photoredox catalysis via sensitized triplet–triplet annihilation upconversion. The photosensitizer is symbolized as PS, while the annihilator as A. This figure has been reproduced from ref. 26 with permission from John Wiley and Sons, copyright 2020. | |
4 Conclusions and perspective
In this minireview, Vis-NIR activation of PC by using molecular absorbers has been demonstrated to be still a valid approach almost even after 40 years from its introduction. Indeed, the most recent sensitization strategies exploit both new PC and new PS, but also new synthetic approaches as the incorporation of molecular absorber in the PC structure. Eventually, the development of new PS and new scheme of photoreaction involving multiple photon absorption has been achieved in many cases. In general, the molecular strategy is very direct and can be easily adapted by most PC. On the contrary, a main concern is still the use of PS based on rare transition metal complexes like Ru and Ir. In principle, the materials discussed in this minireview have been demonstrated to be suitable for multiple Vis-NIR based photocatalytic applications, ranging from solar fuel production to environmental remediation. Nevertheless inorganic semiconductor nanostructures demonstrated to be still superior in term of stability and charge separation efficiency and they are still a primary choice for long term use as in the case of platforms for solar energy conversion. On the other hand, materials like MOF and COF can be easily designed to exploit Vis-NIR light and thanks to their porosity and ability of binding target molecules like CO2 are promising alternative to inorganic semiconductor, although further efforts are required in order to improve their efficiency and stability.
In general, it must be noted that the global scale application, as solar fuel production (from water or CO2 abetment) or environmental remediation, require a photocatalytic scheme based on low cost, non-toxic materials easily producible on the large scale.40 For this materials like g-CN are attracting increasing attention although their photocatalytic properties still need improvement.
Even if less strictly, the above mentioned features are also important in the case of homogenous photocatalysis applied to organic synthesis.
In this context, the use of organic PS and multiphotonic scheme is surely promising. Nevertheless, the long-term stability of organic PS in view of their real application needs still to be demonstrated and reassured. Up to now, the comparison of the actual photocatalytic efficiency of the different systems is complicated by the variety of experimental conditions and in particular, by the use of sacrificial agents, the decomposition of which needs indeed to be considered in the overall energetic storage balance. In general, the efficiencies that have been reported for photocatalytic solar fuel production do not seem to meet the huge energy demands of the modern society, demonstrating how challenging photocatalysis is and the need for even more dedication and effort, also in terms of investment.
Author contributions
A. M.-P., A. M., M. G. and M. M. contributed to the bibliographic search and to the writing of this minireview.
Conflicts of interest
There are no conflicts to declare.
Acknowledgements
This research was funded by the Italian Ministry of Education, University and Research (MIUR), (PRIN project: PRIN 2017) 2017E44A9P (BacHound).
Notes and references
- N. Armaroli and V. Balzani, Angew. Chem., Int. Ed., 2007, 46, 52–66 CrossRef CAS PubMed.
- Q. Wang and K. Domen, Chem. Rev., 2020, 120, 919–985 CrossRef CAS PubMed.
- Z. Wang, C. Li and K. Domen, Chem. Soc. Rev., 2019, 48, 2109–2125 RSC.
- X. Zhang, T. Peng and S. Song, J. Mater. Chem. A, 2016, 4, 2365–2402 RSC.
- W. H. Zhang, A. R. Mohamed and W. J. Ong, Angew. Chem., Int. Ed., 2020, 59, 22894–22915 CrossRef CAS.
- X. Li, J. G. Yu, M. Jaroniec and X. B. Chen, Chem. Rev., 2019, 119, 3962–4179 CrossRef CAS PubMed.
- S. C. Shit, I. Shown, R. Paul, K. H. Chen, J. Mondal and L. C. Chen, Nanoscale, 2020, 12, 33 RSC.
- Q. Q. Zhou, Y. Q. Zou, L. Q. Lu and W. J. Xiao, Angew. Chem., Int. Ed., 2019, 58, 1586–1604 CrossRef CAS PubMed.
- L. Buzzetti, G. E. M. Crisenza and P. Melchiorre, Angew. Chem., Int. Ed., 2019, 58, 3730–3747 CrossRef CAS PubMed.
- F. Strieth-Kalthoff, M. J. James, M. Teders, L. Pitzer and F. Glorius, Chem. Soc. Rev., 2018, 47, 7190–7202 RSC.
- S. Crespi and M. Fagnoni, Chem. Rev., 2020, 120, 9790–9833 CrossRef CAS PubMed.
- Y. S. Jiang and E. A. Weiss, J. Am. Chem. Soc., 2020, 142, 15219–15229 CrossRef CAS PubMed.
- X. Pang, N. Skillen, N. Gunaratne, D. W. Rooney and P. K. J. Robertson, J. Hazard. Mater., 2021, 402, 123461 CrossRef CAS PubMed.
- A. H. Mamaghani, F. Haghighat and C. S. Lee, Build. Environ., 2021, 189, 11 CrossRef.
- G. Guidetti, E. A. A. Pogna, L. Lombardi, F. Tomarchio, I. Polishchuk, R. R. M. Joosten, A. Ianiro, G. Soavi, N. A. J. M. Sommerdijk, H. Friedrich, B. Pokroy, A. K. Ott, M. Goisis, F. Zerbetto, G. Falini, M. Calvaresi, A. C. Ferrari, G. Cerullo and M. Montalti, Nanoscale, 2019, 11, 19301–19314 RSC.
- T. Banerjee, F. Podjaski, J. Kroger, B. P. Biswal and B. V. Lotsch, Nat. Rev. Mater., 2021, 6, 168–190 CrossRef CAS.
- T. Takata, J. Jiang, Y. Sakata, M. Nakabayashi, N. Shibata, V. Nandal, K. Seki, T. Hisatomi and K. Domen, Nature, 2020, 581, 411–414 CrossRef CAS PubMed.
- S. Zhang, P. C. Gu, R. Ma, C. T. Luo, T. Wen, G. X. Zhao, W. C. Cheng and X. K. Wang, Catal. Today, 2019, 335, 65–77 CrossRef CAS.
- Q. Tian, W. Yao, W. Wu and C. Jiang, Nanoscale Horiz., 2019, 4, 10–25 RSC.
- N. Corrigan, J. Yeow, P. Judzewitsch, J. Xu and C. Boyer, Angew. Chem., Int. Ed., 2019, 58, 5170–5189 CrossRef CAS PubMed.
- X.-C. Dai, M.-H. Huang, Y.-B. Li, T. Li, S. Hou, Z.-Q. Wei and F.-X. Xiao, J. Phys. Chem. C, 2020, 124, 4989–4998 CrossRef CAS.
- N. N. Vu, S. Kaliaguine and T. O. Do, ChemSusChem, 2020, 13, 3967–3991 CrossRef CAS PubMed.
- E. Borgarello, J. Kiwi, E. Pelizzetti, M. Visca and M. Grätzel, Nature, 1981, 289, 158–160 CrossRef CAS.
- Q. Wang and D. Astruc, Chem. Rev., 2020, 120, 1438–1511 CrossRef CAS PubMed.
- Q. Yang, M. L. Luo, K. W. Liu, H. M. Cao and H. J. Yan, Appl. Catal., B, 2020, 276, 18 Search PubMed.
- F. Glaser, C. Kerzig and O. S. Wenger, Angew. Chem., Int. Ed., 2020, 59, 10266–10284 CrossRef CAS PubMed.
-
M. Montalti, A. Credi, L. Prodi and M. T. Gandolfi, Handbook of Photochemistry, 3rd edn, Taylor & Francis, 2006 Search PubMed.
- C. Wu, K. Jung, Y. Ma, W. Liu and C. Boyer, Nat. Commun., 2021, 12, 478 CrossRef CAS PubMed.
- C. Wu, H. Chen, N. Corrigan, K. Jung, X. Kan, Z. Li, W. Liu, J. Xu and C. Boyer, J. Am. Chem. Soc., 2019, 141, 8207–8220 CrossRef CAS PubMed.
- C. Wu, N. Corrigan, C.-H. Lim, K. Jung, J. Zhu, G. Miyake, J. Xu and C. Boyer, Macromolecules, 2019, 52, 236–248 CrossRef CAS PubMed.
- S. Shanmugam, J. Xu and C. Boyer, Angew. Chem., Int. Ed., 2016, 55, 1036–1040 CrossRef CAS PubMed.
- S. Shanmugam, J. Xu and C. Boyer, Chem. Sci., 2015, 6, 1341–1349 RSC.
- M. J. Buriak, P. V. Kamat and K. S. Schanze, ACS Appl. Mater. Interfaces, 2014, 6, 11815–11816 CrossRef PubMed.
- M. Qureshi and K. Takanabe, Chem. Mater., 2017, 29, 158–167 CrossRef CAS.
- A. Naldoni, M. Altomare, G. Zoppellaro, N. Liu, S. Kment, R. Zboril and P. Schmuki, ACS Catal., 2019, 9, 345–364 CrossRef CAS PubMed.
- E. Peiris, S. Sarina, E. R. Waclawik, G. A. Ayoko, P. F. Han, J. F. Jia and H. Y. Zhu, Angew. Chem., Int. Ed., 2019, 58, 12032–12036 CrossRef CAS PubMed.
- Z. Hu, Y. Mi, Y. Ji, R. Wang, W. Zhou, X. Qiu, X. Liu, Z. Fang and X. Wu, Nanoscale, 2019, 11, 16445–16454 RSC.
- Y. Wang, X. H. Liu, Q. K. Wang, M. Quick, S. A. Kovalenko, Q. Y. Chen, N. Koch and N. Pinna, Angew. Chem., Int. Ed., 2020, 59, 7748–7754 CrossRef CAS PubMed.
- Z. Y. Zhang, C. R. Rogers and E. A. Weiss, J. Am. Chem. Soc., 2020, 142, 495–501 CrossRef CAS PubMed.
- T. Hisatomi and K. Domen, Nat. Catal., 2019, 2, 387–399 CrossRef CAS.
- A. Y. Meng, L. Y. Zhang, B. Cheng and J. G. Yu, Adv. Mater., 2019, 31, 1807660 CrossRef PubMed.
- W. Zhang, H. He, H. Li, L. Duan, L. Zu, Y. Zhai, W. Li, L. Wang, H. Fu and D. Y. Zhao, Adv. Energy Mater., 2021, 11, 2003303 CrossRef CAS.
- J. Warnan, J. Willkomm, J. N. Ng, R. Godin, S. Prantl, J. R. Durrant and E. Reisner, Chem. Sci., 2017, 8, 3070–3079 RSC.
- N. Manfredi, B. Cecconi, V. Calabrese, A. Minotti, F. Peri, R. Ruffo, M. Monai, I. Romero-Ocaña, T. Montini, P. Fornasiero and A. Abbotto, Chem. Commun., 2016, 52, 6977–6980 RSC.
- N. Manfredi, M. Monai, T. Montini, M. Salamone, R. Ruffo, P. Fornasiero and A. Abbotto, Sustainable Energy Fuels, 2017, 1, 694–698 RSC.
- J. Warnan, J. Willkomm, Y. Farré, Y. Pellegrin, M. Boujtita, F. Odobel and E. Reisner, Chem. Sci., 2019, 10, 2758–2766 RSC.
- Y. C. Xu, J. X. Zheng, J. O. Lindner, X. B. Wen, N. Q. Jiang, Z. C. Hu, L. L. Liu, F. Huang, F. Wurthner and Z. Q. Xie, Angew. Chem., Int. Ed., 2020, 59, 10363–10367 CrossRef CAS PubMed.
- I. Ghosh, T. Ghosh, J. I. Bardagi and B. König, Science, 2014, 346, 725–728 CrossRef CAS PubMed.
- L. Zeng, T. Liu, C. He, D. Shi, F. Zhang and C. Duan, J. Am. Chem. Soc., 2016, 138, 3958–3961 CrossRef CAS PubMed.
- M. Marchini, A. Gualandi, L. Mengozzi, P. Franchi, M. Lucarini, P. G. Cozzi, V. Balzani and P. Ceroni, Phys. Chem. Chem. Phys., 2018, 20, 8071–8076 RSC.
- C. J. Zeman, S. Kim, F. Zhang and K. S. Schanze, J. Am. Chem. Soc., 2020, 142, 2204–2207 CrossRef CAS PubMed.
- M. Bonchio, Z. Syrgiannis, M. Burian, N. Marino, E. Pizzolato, K. Dirian, F. Rigodanza, G. A. Volpato, G. La Ganga, N. Demitri, S. Berardi, H. Amenitsch, D. M. Guldi, S. Caramori, C. A. Bignozzi, A. Sartorel and M. Prato, Nat. Chem., 2019, 11, 146–153 CrossRef CAS PubMed.
- S.-L. Xie, J. Liu, L.-Z. Dong, S.-L. Li, Y.-Q. Lan and Z.-M. Su, Chem. Sci., 2019, 10, 185–190 RSC.
- Y. H. Zhong, Y. Lei, J. F. Huang, L. M. Xiao, X. L. Chen, T. Luo, S. Qin, J. Guo and J. M. Liu, J. Mater. Chem. A, 2020, 8, 8883–8891 RSC.
- D. Wang, Y. Wang, M. D. Brady, M. V. Sheridan, B. D. Sherman, B. H. Farnum, Y. Liu, S. L. Marquard, G. J. Meyer, C. J. Dares and T. J. Meyer, Chem. Sci., 2019, 10, 4436–4444 RSC.
- H. Huang, B. Pradhan, J. Hofkens, M. B. J. Roeffaers and J. A. Steele, ACS Energy Lett., 2020, 5, 1107–1123 CrossRef CAS.
- Z. J. Chen, Y. G. Hu, J. Wang, Q. Shen, Y. H. Zhang, C. Ding, Y. Bai, G. C. Jiang, Z. Q. Li and N. Gaponik, Chem. Mater., 2020, 32, 1517–1525 CrossRef CAS.
- W. J. Yin, B. C. Weng, J. Ge, Q. D. Sun, Z. Z. Li and Y. F. Yan, Energy Environ. Sci., 2019, 12, 442–462 RSC.
- H. Wang, X. Wang, H. Zhang, W. Ma, L. Wang and X. Zong, Nano Energy, 2020, 71, 104647 CrossRef CAS.
- R. Li, X. Li, J. Wu, X. Lv, Y.-Z. Zheng, Z. Zhao, X. Ding, X. Tao and J.-F. Chen, Appl. Catal., B, 2019, 259, 118075 CrossRef CAS.
- T. Oshima, S. Nishioka, Y. Kikuchi, S. Hirai, K. I. Yanagisawa, M. Eguchi, Y. Miseki, T. Yokoi, T. Yui, K. Kimoto, K. Sayama, O. Ishitani, T. E. Mallouk and K. Maeda, J. Am. Chem. Soc., 2020, 142, 8412–8420 CrossRef CAS PubMed.
- K.-Q. Lu, Y.-H. Li, F. Zhang, M.-Y. Qi, X. Chen, Z.-R. Tang, Y. M. A. Yamada, M. Anpo, M. Conte and Y.-J. Xu, Nat. Commun., 2020, 11, 5181 CrossRef CAS PubMed.
- A. H. Cheshme Khavar, G. Moussavi, K. Yaghmaeian, A. R. Mahjoub, N. Khedri, M. Dusek, T. Vaclavu and M. Hosseini, RSC Adv., 2020, 10, 22500–22514 RSC.
- H. Li, X. Jia, Q. Zhang and X. Wang, Chem, 2018, 4, 1510–1537 CrossRef CAS PubMed.
- C. K. Sumesh and S. C. Peter, Dalton Trans., 2019, 48, 12772–12802 RSC.
- J. Peng, X. Chen, W.-J. Ong, X. Zhao and N. Li, Chem, 2019, 5, 18–50 CAS.
- M. Jourshabani, B. K. Lee and Z. Shariatinia, Appl. Catal., B, 2020, 276, 28 CrossRef.
- C. Bie, H. Yu, B. Cheng, W. Ho, J. Fan and J. Yu, Adv. Mater., 2021, 33, 2003521 CrossRef CAS PubMed.
- D. Dinda, H. Park, H.-J. Lee, S. Oh and S. Y. Park, Carbon, 2020, 167, 760–769 CrossRef CAS.
- J. Pang, R. G. Mendes, A. Bachmatiuk, L. Zhao, H. Q. Ta, T. Gemming, H. Liu, Z. Liu and M. H. Rummeli, Chem. Soc. Rev., 2019, 48, 72–133 RSC.
- X. Xie and N. Zhang, Adv. Funct. Mater., 2020, 30, 2002528 CrossRef CAS.
- H. Wang, C. Qian, J. Liu, Y. Zeng, D. Wang, W. Zhou, L. Gu, H. Wu, G. Liu and Y. Zhao, J. Am. Chem. Soc., 2020, 142, 4862–4871 CrossRef CAS PubMed.
- R. Xiao, C. Zhao, Z. Zou, Z. Chen, L. Tian, H. Xu, H. Tang, Q. Liu, Z. Lin and X. Yang, Appl. Catal., B, 2020, 268, 118382 CrossRef CAS.
- S. Min, Y. Xue, F. Wang, Z. Zhang and H. Zhu, Chem. Commun., 2019, 55, 10631–10634 RSC.
- Y. Sun, Y. Sun, X. Meng, Y. Gao, Y. Dall'Agnese, G. Chen, C. Dall'Agnese and X.-F. Wang, Catal. Sci. Technol., 2019, 9, 310–315 RSC.
- X. H. Ren, D. Philo, Y. X. Li, L. Shi, K. Chang and J. H. Ye, Coord. Chem. Rev., 2020, 424, 213516 CrossRef CAS.
- D. Xia, Z. Shen, G. Huang, W. Wang, J. C. Yu and P. K. Wong, Environ. Sci. Technol., 2015, 49, 6264–6273 CrossRef CAS PubMed.
- L. F. Hong, R. T. Guo, Y. Yuan, X. Y. Ji, Z. D. Lin, Z. S. Li and W. G. Pan, ChemSusChem, 2021, 14, 539–557 CrossRef CAS PubMed.
- H. Liu, K. Hu, D. Yan, R. Chen, Y. Zou, H. Liu and S. Wang, Adv. Mater., 2018, 30, 1800295 CrossRef PubMed.
- T. Sakthivel, X. Huang, Y. Wu and S. Rtimi, Chem. Eng. J., 2020, 379, 122297 CrossRef CAS.
- Y. Abate, D. Akinwande, S. Gamage, H. Wang, M. Snure, N. Poudel and S. B. Cronin, Adv. Mater., 2018, 30, 1704749 CrossRef PubMed.
- B. Li, C. Lai, G. Zeng, D. Huang, L. Qin, M. Zhang, M. Cheng, X. Liu, H. Yi, C. Zhou, F. Huang, S. Liu and Y. Fu, Small, 2019, 15, 1804565 CrossRef PubMed.
- T. Sakthivel, X. Huang, Y. Wu and S. Rtimi, Chem. Eng. J., 2020, 379, 122297 CrossRef CAS.
- Q. Zhang, S. Huang, J. Deng, D. T. Gangadharan, F. Yang, Z. Xu, G. Giorgi, M. Palummo, M. Chaker and D. Ma, Adv. Funct. Mater., 2019, 29, 1902486 CrossRef.
- L. Mao, X. Cai, S. Yang, K. Han and J. Zhang, Appl. Catal., B, 2019, 242, 441–448 CrossRef CAS.
- M. Zhu, M. Fujitsuka, L. Zeng, M. Liu and T. Majima, Appl. Catal., B, 2019, 256, 117864 CrossRef CAS.
- Y. Xue, S. Min and F. Wang, Int. J. Hydrogen Energy, 2019, 44, 21873–21881 CrossRef CAS.
- A. Cadranel, J. T. Margraf, V. Strauss, T. Clark and D. M. Guldi, Acc. Chem. Res., 2019, 52, 955–963 CrossRef CAS PubMed.
- Y. Wang, X. Liu, X. Y. Han, R. Godin, J. L. Chen, W. Z. Zhou, C. R. Jiang, J. F. Thompson, K. B. Mustafa, S. A. Shevlin, J. R. Durrant, Z. X. Guo and J. W. Tang, Nat. Commun., 2020, 11, 9 CrossRef PubMed.
- R. Malik and V. K. Tomer, Renewable Sustainable Energy Rev., 2021, 135, 110235 CrossRef CAS.
- A. Kumar, P. Raizada, V. Kumar Thakur, V. Saini, A. Aslam Parwaz Khan, N. Singh and P. Singh, Chem. Eng. Sci., 2021, 230, 116219 CrossRef CAS.
- Y. Zhou, Z. Wang, L. Huang, S. Zaman, K. Lei, T. Yue, Z. a. Li, B. You and B. Y. Xia, Adv. Energy Mater., 2021, 2003159 CrossRef CAS.
- C. Zhang, Y. Li, D. M. Shuai, Y. Shen, W. Xiong and L. Q. Wang, Chemosphere, 2019, 214, 462–479 CrossRef CAS PubMed.
- K. R. Reddy, C. V. Reddy, M. N. Nadagouda, N. P. Shetti, S. Jaesool and T. M. Aminabhavi, J. Environ. Manage., 2019, 238, 25–40 CrossRef CAS PubMed.
- C. C. Wang, X. H. Yi and P. Wang, Appl. Catal., B, 2019, 247, 24–48 CrossRef CAS.
- M. Jourshabani, B.-K. Lee and Z. Shariatinia, Appl. Catal., B, 2020, 276, 119157 CrossRef CAS.
- S. Min and G. Lu, J. Phys. Chem. C, 2012, 116, 19644–19652 CrossRef CAS.
- X. Zhang, L. Yu, C. Zhuang, T. Peng, R. Li and X. Li, ACS Catal., 2014, 4, 162–170 CrossRef CAS.
- X. Zhang, T. Peng, L. Yu, R. Li, Q. Li and Z. Li, ACS Catal., 2015, 5, 504–510 CrossRef CAS.
- W. Lu, T. Xu, Y. Wang, H. Hu, N. Li, X. Jiang and W. Chen, Appl. Catal., B, 2016, 180, 20–28 CrossRef CAS.
- J. Liu, H. Shi, Q. Shen, C. Guo and G. Zhao, Green Chem., 2017, 19, 5900–5910 RSC.
- S. Mei, J. Gao, Y. Zhang, J. Yang, Y. Wu, X. Wang, R. Zhao, X. Zhai, C. Hao, R. Li and J. Yan, J. Colloid Interface Sci., 2017, 506, 58–65 CrossRef CAS PubMed.
- D. H. Wang, J. N. Pan, H. H. Li, J. J. Liu, Y. B. Wang, L. T. Kang and J. N. Yao, J. Mater. Chem. A, 2016, 4, 290–296 RSC.
- D. Yang, M. H. Cao, Q. X. Zhong, P. L. Li, X. H. Zhang and Q. Zhang, J. Mater. Chem. C, 2019, 7, 757–789 RSC.
- E. S. Da Silva, N. M. M. Moura, M. Neves, A. Coutinho, M. Prieto, C. G. Silva and J. L. Faria, Appl. Catal., B, 2018, 221, 56–69 CrossRef CAS.
- J. Wang, D. Liu, Q. Liu, T. Peng, R. Li and S. Zhou, Appl. Surf. Sci., 2019, 464, 255–261 CrossRef CAS.
- S. Tian, S. Chen, X. Ren, R. Cao, H. Hu and F. Bai, Nano Res., 2019, 12, 3109–3115 CrossRef CAS.
- Y.-j. Yang, B.-w. Sun, D.-j. Qian and M. Chen, Appl. Surf. Sci., 2019, 478, 1027–1036 CrossRef CAS.
- X. Zhang, L. Lin, D. Qu, J. Yang, Y. Weng, Z. Wang, Z. Sun, Y. Chen and T. He, Appl. Catal., B, 2020, 265, 118595 CrossRef.
- L. Li, G. B. Bodedla, Z. Liu and X. Zhu, Appl. Surf. Sci., 2020, 499, 143755 CrossRef CAS.
- L. Lin, C. C. Hou, X. H. Zhang, Y. J. Wang, Y. Chen and T. He, Appl. Catal., B, 2018, 221, 312–319 CrossRef CAS.
- S. Roy and E. Reisner, Angew. Chem., Int. Ed., 2019, 58, 12180–12184 CrossRef CAS PubMed.
- P. Zeng, J. Wang, Y. Guo, R. Li, G. Mei and T. Peng, Chem. Eng. J., 2019, 373, 651–659 CrossRef CAS.
- Y. Yi, S. Wang, H. Zhang, J. Liu, X. Lu, L. Jiang, C. Sui, H. Fan, S. Ai and J. Sun, J. Mater. Chem. C, 2020, 8, 17157–17161 RSC.
- Y.-J. Yuan, Z.-K. Shen, P. Wang, Z. Li, L. Pei, J. Zhong, Z. Ji, Z.-T. Yu and Z. Zou, Appl. Catal., B, 2020, 260, 118179 CrossRef CAS.
- Y. F. Liu, M. F. He, R. Guo, Z. R. Fang, S. F. Kang, Z. Ma, M. D. Dong, W. L. Wang and L. F. Cui, Appl. Catal., B, 2020, 260, 118137 CrossRef CAS.
- X.-B. Meng, J.-L. Sheng, H.-L. Tang, X.-J. Sun, H. Dong and F.-M. Zhang, Appl. Catal., B, 2019, 244, 340–346 CrossRef CAS.
- B. Q. Xia, J. R. Ran, S. M. Chen, L. Song, X. L. Zhang, L. Q. Jing and S. Z. Qiao, Nanoscale, 2019, 11, 8304–8309 RSC.
- W. Chen, B. Han, C. Tian, X. Liu, S. Liang, H. Deng and Z. Lin, Appl. Catal., B, 2019, 244, 996–1003 CrossRef CAS.
- Y. A. Wang, W. L. Zhen, Y. Q. Zeng, S. P. Wan, H. W. Guo, S. L. Zhang and Q. Zhong, J. Mater. Chem. A, 2020, 8, 6034–6040 RSC.
- J. T. Ren, Y. L. Zheng, K. Yuan, L. Zhou, K. Wu and Y. W. Zhang, Nanoscale, 2020, 12, 755–762 RSC.
- Q. Wang, G. Wang, X. Liang, X. Dong and X. Zhang, Appl. Surf. Sci., 2019, 467–468, 320–327 CAS.
- S. Feng, R. Wang, S. Feng, Z. Zhang and L. Mao, Res. Chem. Intermed., 2019, 45, 1263–1279 CrossRef CAS.
- P. Li, J. Li, X. Feng, J. Li, Y. Hao, J. Zhang, H. Wang, A. Yin, J. Zhou, X. Ma and B. Wang, Nat. Commun., 2019, 10, 2177 CrossRef.
- H. Liu, C. Xu, D. Li and H.-L. Jiang, Angew. Chem., Int. Ed., 2018, 57, 5379–5383 CrossRef CAS PubMed.
- S. J. Liu, C. Zhang, Y. D. Sun, Q. Chen, L. F. He, K. Zhang, J. Zhang, B. Liu and L. F. Chen, Coord. Chem. Rev., 2020, 413, 17 CrossRef.
- Y. S. Wei, M. Zhang, R. Q. Zou and Q. Xu, Chem. Rev., 2020, 120, 12089–12174 CrossRef CAS PubMed.
- M. M. Wang, Y. F. Tang and Y. D. Jin, ACS Catal., 2019, 9, 11502–11514 CrossRef CAS.
- C. Wang, X. Liu, N. Keser Demir, J. P. Chen and K. Li, Chem. Soc. Rev., 2016, 45, 5107–5134 RSC.
- S. Vajda and M. G. White, ACS Catal., 2015, 5, 7152–7176 CrossRef CAS.
- J. He, Y. Zhang, J. He, X. Zeng, X. Hou and Z. Long, Chem. Commun., 2018, 54, 8610–8613 RSC.
- M. A. Nasalevich, M. G. Goesten, T. J. Savenije, F. Kapteijn and J. Gascon, Chem. Commun., 2013, 49, 10575–10577 RSC.
- A. Cadiau, N. Kolobov, S. Srinivasan, M. G. Goesten, H. Haspel, A. V. Bavykina, M. R. Tchalala, P. Maity, A. Goryachev, A. S. Poryvaev, M. Eddaoudi, M. V. Fedin, O. F. Mohammed and J. Gascon, Angew. Chem., Int. Ed., 2020, 59, 13468–13472 CrossRef CAS PubMed.
- Y. Isaka, Y. Kawase, Y. Kuwahara, K. Mori and H. Yamashita, Angew. Chem., Int. Ed., 2019, 58, 5402–5406 CrossRef CAS PubMed.
- X.-K. Wang, J. Liu, L. Zhang, L.-Z. Dong, S.-L. Li, Y.-H. Kan, D.-S. Li and Y.-Q. Lan, ACS Catal., 2019, 9, 1726–1732 CrossRef CAS.
- J. Xu, Y. Qi, C. Wang and L. Wang, Appl. Catal., B, 2019, 241, 178–186 CrossRef CAS.
- Q. Li, Z. Fan, L. Zhang, Y. Li, C. Chen, R. Zhao and W. Zhu, J. Solid State Chem., 2019, 269, 465–475 CrossRef CAS.
- L. Zhang, X. Shi, Z. Zhang, R. P. Kuchel, R. Namivandi-Zangeneh, N. Corrigan, K. Jung, K. Liang and C. Boyer, Angew. Chem., Int. Ed., 2021, 60, 5489–5496 CrossRef CAS PubMed.
- K. Gottschling, G. Savasci, H. Vignolo-Gonzalez, S. Schmidt, P. Mauker, T. Banerjee, P. Rovo, C. Ochsenfeld and B. V. Lotsch, J. Am. Chem. Soc., 2020, 142, 12146–12156 CrossRef CAS PubMed.
- W. Huang, N. Huber, S. Jiang, K. Landfester and K. A. I. Zhang, Angew. Chem., Int. Ed., 2020, 59, 18368–18373 CrossRef CAS PubMed.
- R. Y. Liu, K. T. Tan, Y. F. Gong, Y. Z. Chen, Z. E. Li, S. L. Xie, T. He, Z. Lu, H. Yang and D. L. Jiang, Chem. Soc. Rev., 2021, 50, 120–242 RSC.
- K. Y. Geng, T. He, R. Y. Liu, S. Dalapati, K. T. Tan, Z. P. Li, S. S. Tao, Y. F. Gong, Q. H. Jiang and D. L. Jiang, Chem. Rev., 2020, 120, 8814–8933 CrossRef CAS PubMed.
- C. Krishnaraj, H. S. Jena, L. Bourda, A. Laemont, P. Pachfule, J. Roeser, C. V. Chandran, S. Borgmans, S. M. J. Rogge, K. Leus, C. V. Stevens, J. A. Martens, V. Van Speybroeck, E. Breynaert, A. Thomas and P. Van der Voort, J. Am. Chem. Soc., 2020, 142, 20107–20116 CrossRef CAS PubMed.
- N. Keller and T. Bein, Chem. Soc. Rev., 2021, 50, 1813–1845 RSC.
- W. Q. Li, X. F. Huang, T. W. Zeng, Y. H. A. Liu, W. B. Hu, H. Yang, Y. B. Zhang and K. Wen, Angew. Chem., Int. Ed., 2021, 60, 1869–1874 CrossRef CAS PubMed.
- Z.-Z. Gao, Z.-K. Wang, L. Wei, G. Yin, J. Tian, C.-Z. Liu, H. Wang, D.-W. Zhang, Y.-B. Zhang, X. Li, Y. Liu and Z.-T. Li, ACS Appl. Mater. Interfaces, 2020, 12, 1404–1411 CrossRef CAS PubMed.
- Z. Fu, X. Wang, A. M. Gardner, X. Wang, S. Y. Chong, G. Neri, A. J. Cowan, L. Liu, X. Li, A. Vogel, R. Clowes, M. Bilton, L. Chen, R. S. Sprick and A. I. Cooper, Chem. Sci., 2020, 11, 543–550 RSC.
- Z. Y. Dong, L. Zhang, J. Gong and Q. Zhao, Chem. Eng. J., 2021, 403, 10 CrossRef.
- X. X. Yu, Z. Z. Yang, B. Qiu, S. E. Guo, P. Yang, B. Yu, H. Y. Zhang, Y. F. Zhao, X. Z. Yang, B. X. Han and Z. M. Liu, Angew. Chem., Int. Ed., 2019, 58, 632–636 CrossRef CAS PubMed.
- X. Kang, X. W. Wu, X. Han, C. Yuan, Y. Liu and Y. Cui, Chem. Sci., 2020, 11, 1494–1502 RSC.
- F. Strieth-Kalthoff and F. Glorius, Chem, 2020, 6, 1888–1903 CAS.
- A. Bhattacherjee, M. Sneha, L. Lewis-Borrell, G. Amoruso, T. A. A. Oliver, J. Tyler, I. P. Clark and A. J. Orr-Ewing, J. Am. Chem. Soc., 2021, 143, 3613–3627 CrossRef CAS PubMed.
- M. Yang, J. E. Yarnell, K. El Roz and F. N. Castellano, ACS Appl. Energy Mater., 2020, 3, 1842–1853 CrossRef CAS.
- D. Kong, M. Munch, Q. Qiqige, C. J. C. Cooze, B. H. Rotstein and R. J. Lundgren, J. Am. Chem. Soc., 2021, 143, 2200–2206 CrossRef CAS PubMed.
- J.-H. Shon, D. Kim, M. D. Rathnayake, S. Sittel, J. Weaver and T. S. Teets, Chem. Sci., 2021, 12, 4069–4078 RSC.
- A. Shatskiy, A. Axelsson, E. V. Stepanova, J.-Q. Liu, A. Z. Temerdashev, B. P. Kore, B. Blomkvist, J. M. Gardner, P. Dinér and M. D. Kärkäs, Chem. Sci., 2021, 12, 5430–5437 RSC.
- L.-L. Liao, G.-M. Cao, Y.-X. Jiang, X.-H. Jin, X.-L. Hu, J. J. Chruma, G.-Q. Sun, Y.-Y. Gui and D.-G. Yu, J. Am. Chem. Soc., 2021, 143, 2812–2821 CrossRef CAS PubMed.
- K.-K. Chen, S. Guo, H. Liu, X. Li, Z.-M. Zhang and T.-B. Lu, Angew. Chem., Int. Ed., 2020, 59, 12951–12957 CrossRef CAS PubMed.
- Y. Zhang, T. S. Lee, J. M. Favale, D. C. Leary, J. L. Petersen, G. D. Scholes, F. N. Castellano and C. Milsmann, Nat. Chem., 2020, 12, 345–352 CrossRef CAS PubMed.
- B. D. Ravetz, A. B. Pun, E. M. Churchill, D. N. Congreve, T. Rovis and L. M. Campos, Nature, 2019, 565, 343–346 CrossRef CAS PubMed.
- A. Mahammed, K. P. Chen, J. Vestfrid, J. Z. Zhao and Z. Gross, Chem. Sci., 2019, 10, 7091–7103 RSC.
- I. A. MacKenzie, L. Wang, N. P. R. Onuska, O. F. Williams, K. Begam, A. M. Moran, B. D. Dunietz and D. A. Nicewicz, Nature, 2020, 580, 76–80 CrossRef CAS PubMed.
|
This journal is © The Royal Society of Chemistry 2021 |
Click here to see how this site uses Cookies. View our privacy policy here.